DOI:
10.1039/C1AN15639J
(Paper)
Analyst, 2012,
137, 140-144
Disposable electrochemiluminescent biosensor using bidentate-chelated CdTe quantum dots as emitters for sensitive detection of glucose
Received
24th July 2011
, Accepted 29th September 2011
First published on 28th October 2011
Abstract
A novel disposable solid-state electrochemiluminescent (ECL) biosensor was fabricated by immobilizing glucose oxidase and surface-unpassivated CdTe quantum dots (QDs) on a screen-printed carbon electrode (SPCE). The surface morphology of the biosensor was characterized with scanning electron microscopy and atomic force microscopy. With dissolved O2 as an endogenous coreactant, QDs/SPCE showed strong ECL emission in pH 9.0 HCl-Tris buffer solution with low ECL peak potential at −0.89 V. The ECL intensity was twice that with hydrogen peroxide as coreactant at the same concentration. This phenomenon meant the ECL decreased upon consumption of dissolved O2 and thus could be applied to the construction of oxidase-based ECL biosensors. With glucose oxidase as a model enzyme, the biosensor showed rapid response to glucose with a linear range of 0.8 to 100 μM and a detection limit of 0.3 μM. Further detection of glucose contained in human serum samples showed acceptable sensitivity and selectivity. This work provided a promising application of QDs in ECL-based disposable biosensors.
Introduction
Electrochemiluminescence (ECL) is a sensitive technique, in which species are generated at an electrode surface, and then undergo an electron-transfer reaction to form an excited state that emits light.1 Due to the integrated advantages of electrochemistry and chemiluminescence, ECL has been widely applied not only in the direct detection of biomolecules2 but also in comprehensive analytical systems coupled with other separation techniques like chromatography3 and electrophoresis.4 For example, a highly sensitive competitive immunosensor based on the ECL of CdTe quantum dots (QDs) has been constructed for the detection of human IgG with an enzymatic amplification,5 and capillary electrophoresis coupled with ECL detection has been developed for the separation and detection of roundup ready soy.4a These ECL systems usually employ glassy carbon,5 paraffin-impregnated graphite,6 platinum4a and gold mesh as working electrodes,7 which are disadvantageous to the practical application of the ECL technique.
Owing to the low-cost, disposability and mass production capacity, screen-printed electrodes (SPEs) have become a powerful tool for the development of commercial biosensors.8 They have been used for ECL detection of oxalate, lactate, glucose and choline with luminol or [Ru(bpy)3]2+ derivates as light emitters at relatively high potentials.9 Although different QDs have been synthesized as ECL emitters for design of multifarious biosensing strategies10 since the first report on the ECL behavior of QDs,11 and the ECL of QDs possesses many advantages such as neutral detection conditions, facile realization in both cathodic and anodic processes and low applied potentials, these electrodes have not yet been used for the development of QDs-based ECL biosensors.
In order to realize the commercial application of QDs-based ECL biosensors, this work coimmobilized for the first time QDs and enzyme on a screen-printed carbon electrode (SPCE) to prepare a disposable ECL biosensor (Scheme 1). Due to the relatively low solubility of bidentate-chelated CdTe QDs in aqueous solution, the QDs could be directly immobilized on an electrode surface. By combining the surface-unpassivated QDs with an endogenous coreactant, dissolved O2, the as-prepared biosensor showed strong ECL emission at low applied potential.12 Furthermore, the ECL intensity was twice that in nitrogen-saturated buffer containing hydrogen peroxide at the same concentration as dissolved O2, thus the consumption of dissolved O2 in the oxidation reaction catalyzed by oxidase led to a biosensing response to its substrate.2a The proposed disposable ECL biosensor for glucose could be successfully applied to the detection of blood sugar content in human serum samples. This biosensor offers a new opportunity for development of QDs-based ECL techniques in real practice.
Experimental
Materials and reagents
Meso-2,3-dimercaptosuccinic acid (DMSA), glutaraldehyde (25% aqueous solution) and cadmium chloride (CdCl2·2.5H2O) were purchased from Alfa Aesar China Ltd. (China). Chitosan (≥85% deacetylation), tris(hydroxymethyl)-aminomethane hydrochloride and tris(hydroxymethyl)-aminomethane (Tris, reagent grade), glucose oxidase (GOx, 141200 U g−1) and D-(+)-glucose were purchased from Sigma Chemical Co. (MO, USA). Glucose stock solutions were mutarotated overnight at room temperature before use. Isopropyl alcohol (A.R.) was purchased from Nanjing chemical reagent Co., Ltd. A tellurium rod (4 mm in diameter) was purchased from Leshan Kayada Photoelectricity Co. (China). All other chemicals were of analytical grade and used without further purification. 0.1 M pH 9.0 HCl-Tris buffer solution containing 0.1 M KNO3 as the supporting electrolyte was prepared for ECL detection. Ultrapure water (≥18 MΩ, Milli-Q, Millipore) was used throughout the work. Human serum samples were obtained from Jiangsu Institute of Cancer Research (Nanjing, China).
Instrumentation
The synthesis of CdTe/DMSA QDs was carried out at a CHI 812B. Cyclic voltammetric (CV) and ECL experiments were performed on MPI-A multifunctional electrochemical and chemiluminescent system (Xi'an Remex Analytical Instrument Co., Ltd. China) at room temperature. The SPCE was manufactured in standard three-electrode configuration with a carbon paste working electrode, an Ag/AgCl reference electrode and a carbon counter electrode (Scheme 1). The emission window was placed in front of the photomultiplier tube (detection range from 300 to 650 nm) biased at −800 V. UV-vis absorption spectra were recorded with a UV-3600 UV-vis-NIR photospectrometer (Shimadzu Co., Japan). Scanning electron microscopic (SEM) images were obtained using a Hitachi S-4800 scanning electron microscope (Japan). Tapping mode atomic force microscopic (AFM) images were acquired under ambient conditions by using an Agilent 5500 AFM/SPM system. As a control, the concentrations of glucose in clinical human serum samples were obtained from the Roche module p800 automatic biochemical analyzer (Switzerland).
Preparation of CdTe/DMSA QDs
For the synthesis of CdTe QDs the green method with an electrogenerated precursor was referred to.13 Briefly, the CdTe QDs precursor was produced on a CHI 812B using a Te rod as the working electrode at −1.0 V (vs. Ag/AgCl) in 20 mL 0.01 M NaOH containing 12 μmol CdCl2 and 32.9 μmol DMSA as stabilizer under N2 atmosphere. The resulting solution was refluxed at 80 °C for 20 h to obtain the CdTe/DMSA QDs and stored at 4 °C prior to use. The as-prepared QDs showed an absorption peak at 463 nm and an absorbance of 0.415, according to Peng's empirical equation,14 the corresponding diameter was 0.94 nm with the concentration of 47.3 μM.
Preparation of disposable solid-state ECL biosensor
Firstly, 80 μL of QDs solution was mixed with 80 μL of isopropyl alcohol, and centrifuged at 8000 rcf min−1 for 5 min. The precipitation was twice washed with a 1
:
1 mixture of isopropyl alcohol and water, and then dissolved in 2.5 μL water and dropped onto the working electrode of SPCE. After drying in air, 2 μL of 0.025% chitosan solution was coated on the QD film for covalently binding of GOx by activating the chitosan film with 5 μL of 2% glutaraldehyde (in 0.01 M PBS, pH 7.4) for 2 h and incubating 5 μL of GOx (4.0 mg mL−1 in 0.01 M PBS, pH 7.4) for 60 min at room temperature and overnight at 4 °C. The resulting GOx-chitosan/QDs/SPCE was slowly washed with a stream of 0.01 M pH 7.4 PBS for further use. When not in use, the GOx-chitosan/QDs/SPCEs were stored at 4 °C.
Results and discussion
Morphology characterization
The SEM image of the CdTe/DMSA QDs modified SPCE revealed a uniform film with homogeneous diameter of around 20 nm (Fig. 1A). After being covered with chitosan, the morphology became flatter, indicating the assembly of a chitosan membrane (Fig. 1B). When GOx was conjugated on the chitosan/QDs/SPCE, the membrane showed rougher morphology (Fig. 1C), which validated the successful conjugation of GOx to the SPCE surface. The AFM images of QDs, chitosan/QDs and GOx-chitosan/QDs modified mica sheets further demonstrated these morphology changes (Fig. 1D–F). The 3D bird’s eye view of the typical AFM image of QDs indicated the film was porous and the average thickness of QDs aggregations was about 30 to 40 nm (Fig. 1D). The coverage of chitosan produced a height of around 60 nm (Fig. 1E). After GOx was covalently bound to the chitosan surface, the height became 125 nm (Fig. 1F), suggesting the successful binding.
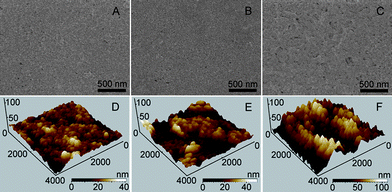 |
| Fig. 1 SEM (A–C) and AFM (D–F) characterizations of QDs/SPCE (A and D), chitosan/QDs/SPCE (B and E) and GOx-chitosan/QDs/SPCE (C and F). | |
CV and ECL behaviors of QDs modified SPCE
In air-saturated pH 9.0 HCl-Tris buffer solution, the chitosan/QDs/SPCE showed a reduction wave starting at about −0.52 V with a sharp increase of reduction current from −0.74 V, which could be attributed to the reduction of QDs to form the electron-injected QDs, respectively (Fig. 2A, inset). In the meantime, the strong ECL emission of QDs was observed at the peak potential of −0.89 V with a starting potential of −0.69 (Fig. 2A). According to the previous mechanism, the ECL process of QDs could be expressed as follows:2a,15 | O2 + 2e− + 2H2O → H2O2 + 2OH− | (1) |
| CdTe QDs + ne− → nCdTe QDs˙− | (2) |
| O2 + 2CdTe QDs˙− + 2H+ → 2CdTe QDs* + H2O2 | (3) |
| H2O2 + 2CdTe QDs˙− → 2CdTe QDs* + 2OH− | (4) |
| Then CdTe QDs* → CdTe QDs + hv | (5) |
During the cathodic scan, one dissolved O2 molecule reacted with four electron-injected QDs, CdTe QDs˙−, to produce excited CdTe QDs, CdTe QDs*, which then emitted ECL (eqn (3)–(5)). In this ECL system no exogenous coreactant was introduced, and the formed H2O2 was also the coreactant for ECL emission of QDs (eqn (4)). Thus, the chitosan/QDs/SPCE could produce an ECL signal in nitrogen-saturated detection solution containing 320 μM H2O2 (Fig. 2B). Interestingly, the ECL intensity was half of that in O2-saturated solution. Considering the same concentration of 320 μM H2O2 as saturated dissolved O2,16eqn (3)–(5) were verified. Thus dissolved O2 was a more efficient coreactant to produce the ECL emission of QDs. The strong and stable ECL of QDs/SPCE with the endogenous coreactant dissolved O2 could be desirable to construct an enzyme-based ECL biosensing platform.
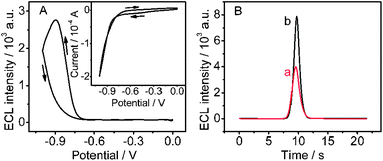 |
| Fig. 2 (A) Cyclic ECL curve and CV curve (inset) of chitosan/QDs/SPCE in air saturated 0.1 M pH 9.0 HCl-Tris buffer and (B) ECL-time curves of chitosan/QDs/SPCE in pH 9.0 HCl-Tris buffer solution containing 0.32 mM H2O2 (a) and saturated O2 (b). Scan rate: 100 mV s−1. | |
Condition optimization for ECL detection
To apply the functional film in biosensing, several experimental parameters including solution pH, the amounts of QDs deposited as well as the immobilized GOx were optimized. The ECL intensity increased with the increasing pH of detection solution from 6.0 to 9.0, and reached a platform after pH 9.0 (Fig. 3A). Considering that pH 10.0 was unfavorable for bioanalysis applications, 0.1 M pH 9.0 HCl-Tris buffer solution was used throughout the following experiments.
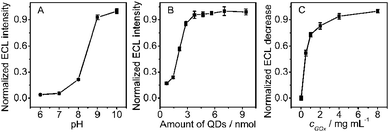 |
| Fig. 3 Effects of (A) pH of air-saturated 0.1 M HCl-Tris buffer and (B) amount of QDs on ECL intensity of chitosan/QDs/SPCE, and (C) concentration of 5 μL GOx solution used for biosensor preparation on ECL response to 0.1 mM glucose, collected at −0.89 V (n = 3). | |
The quantity of the excited state QDs essentially depended on the amount of QDs. With an increase of QDs density, the formed individual nanocrystal species increased during the cathodic scan, leading to enhancement in ECL intensity (Fig. 3B). The ECL intensity reached a platform when 3.76 nmol QDs were cast on SPCE due to the close-packed structure of QDs (Fig. 1A). Although more QDs could increase the QDs density on the electrode surface, the ECL intensity did not obviously increase, which was attributed to the increasing film thickness that inhibited the electron exchange. Since the ECL intensity of 3.76 nmol QDs was 95.7% of the strongest intensity at 7.05 nmol and strong enough for sensitive detection, 3.76 nmol of QDs was chosen for preparation of the QDs modified SPCEs.
The ECL intensity of GOx-chitosan/QDs/SPCE decreased upon addition of glucose in the detection solution, which resulted from the consumption of dissolved O2, a more efficient coreactant than the formed H2O2, in the enzymatic oxidation of glucose by O2 (eqn (6)). |  | (6) |
The enzymatic reaction rate was proportional to the amount of GOx bound to the electrode surface. Thus the ECL decrease of GOx-chitosan/QDs/SPCE increased with the increasing concentration of 5 μL GOx used for biosensor preparation and then tended to the maximum at 4.0 mg mL−1 GOx (Fig. 3C), indicating the saturating binding of GOx on the chitosan membrane. Thus 5 μL of 4.0 mg mL−1 GOx solution was used for the biosensor preparation.
Under optimized conditions, a strong and stable ECL signal could be obtained on the GOx-chitosan/QDs/SPCE. The decrease of ECL signal was proportional to the concentration of glucose in a range of 0.8 to 100 μM (r = 0.995) (Fig. 4). The detection limit was 0.3 μM (S/N = 3), which was lower than that of the ECL strategy at paraffin-impregnated graphite electrode,2a electrochemical17 and chemiluminescence methods.18 Due to the sensitive ECL response and the limited saturating amount of bound GOx, the biosensor showed a low upper limit of detection.
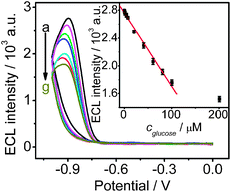 |
| Fig. 4 Cyclic ECL curves of GOx-chitosan/QDs/SPCE in air-saturated 0.1 M pH 9.0 HCl-Tris buffer in presence of (a–g) 0, 7.6, 20.0, 40.0, 60.0, 80.0, and 100.0 μM glucose. Inset: plot of ECL intensity vs. glucose concentration (n = 3). | |
Interference, stability and reproducibility of the proposed sensor
To evaluate the selectivity of the proposed ECL biosensor, the effect of foreign species on the ECL intensity was detected. 0.1 mM of Mg2+, Al3+, Ca2+, Ni2+, Co2+, Zn2+, F−, Cl−, Br−, I−, ascorbic acid (AA), uric acid (UA) and fructose were added into the blank detection solution, respectively. As shown in Fig. 5, no obvious change of ECL intensity could be observed with other species, and only AA and UA had a slight effect on the ECL intensity, indicating the acceptable selectivity of this biosensor.
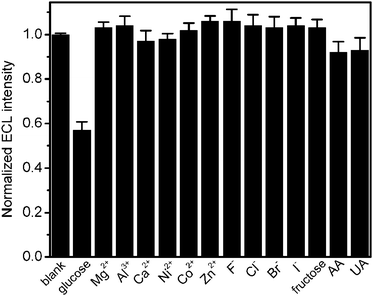 |
| Fig. 5 Normalized ECL intensities of GOx-chitosan/QDs/SPCE in air-saturated 0.1 M pH 9.0 HCl-Tris buffer containing 0.1 mM individual ions (n = 3). | |
Ten measurements of ECL emission upon continuous cyclic scans of chitosan/QDs and GOx-chitosan/QDs modified SPCEs in air-saturated detection solution showed signals with relative standard deviations (RSDs) of 1.9 and 3.4%, respectively, indicating the reliability and stability of the biosensors. The RSD of five parallel measurements (intra-assay) at 40 μM glucose with one biosensor was 1.6%, indicating a good precision. The ECL detection in the presence of 40 μM glucose with five biosensors fabricated independently (inter-assay) showed the RSD of 3.7%, giving acceptable fabrication reproducibility. After the biosensor was stored for 30 days in 4 °C, the ECL response to 50 μM glucose did not show any obvious decline when measured once every five days, indicating acceptable storage stability.
Real samples analysis
To evaluate the analytical reliability and application potential of the proposed method, the disposable QDs-based ECL biosensor was employed to detect glucose content in human serum samples. Serum samples were diluted by 200 times with 0.1 M pH 9.0 HCl-Tris buffer solution prior to the assay. The results were 5.03 ± 0.27, 9.52 ± 0.36 and 8.34 ± 0.19 mM (n = 3) for three samples, which were consistent with the reference values of 5.29, 9.40, and 7.98 mM detected on a Roche automatic biochemical analyzer, respectively, indicating acceptable accuracy. When 0.005 and 0.05 mM glucose solution were injected into the detection solution containing serum sample, the corresponding recoveries were 96.2 ± 4.7% and 102.3 ± 5.4% (n = 3), respectively, indicating good precision.
Conclusions
A disposable QDs-based ECL biosensor has been proposed by immobilizing surface-unpassivated CdTe QDs as ECL emitters and glucose oxidase on SPCE. Due to the porous structure of the QDs film on the SPCE, the resulting biosensor shows strong ECL emission with relatively low ECL peak potential by using dissolved O2 or H2O2 as ECL coreactant. Based on the different efficiencies of dissolved O2 and H2O2 as coreactants, the consumption of dissolved O2 in the enzymatic oxidation of glucose by O2 has been applied in ECL biosensing of glucose. The biosensor shows good performance with acceptable sensitivity, selectivity and precision, and has successfully been used to detect the glucose content in human serum samples. The SPCE-based ECL biosensor not only provides a convenient and low-cost platform to monitor target molecules but also extends the application of QDs-based ECL biosensors.
Acknowledgements
This research was financially supported by Important National S&T Specific Project (2009ZX10004-313), National Basic Research Program of China (2010CB732400) and National Natural Science Foundation of China (20821063, 20875044 and 21075060).
Notes and references
-
(a) M. M. Richter, Chem. Rev., 2004, 104, 3003 CrossRef CAS;
(b) W. J. Miao, Chem. Rev., 2008, 108, 2506 CrossRef CAS.
-
(a) H. Jiang and H. X. Ju, Chem. Commun., 2007, 404 RSC;
(b) X. Zhu, Z. Y. Lin, L. F. Chen, B. Qiu and G. N. Chen, Chem. Commun., 2009, 6050 RSC;
(c) J. X. Li, L. X. Yang, S. L. Luo, B. B. Chen, J. Li, H. L. Lin, Q. Y. Cai and S. Z. Yao, Anal. Chem., 2010, 82, 7357 CrossRef CAS;
(d) X. Yang, R. Yuan, Y. Q. Chai, Y. Zhuo, L. Mao and S. R. Yuan, Biosens. Bioelectron., 2010, 25, 1851 CrossRef CAS.
-
(a) Y. J. Park, D. W. Lee and W. Y. Lee, Anal. Chim. Acta, 2002, 471, 51 CrossRef CAS;
(b) Y. Yamanaka, M. Miyamoto, Y. Tanaka, A. Nagumo, Y. Katsuki, Y. Fukuda, M. Yoshimatsu, A. Takeshige, T. Kondo, A. Fujishima and K. Honda, Electrochim. Acta, 2008, 53, 5397 CrossRef CAS.
-
(a) L. H. Guo, H. H. Yang, B. Qiu, X. Y. Xiao, L. L. Xue, D. Kim and G. N. Chen, Anal. Chem., 2009, 81, 9578 CrossRef CAS;
(b) G. M. Cao, Q. Liu, Y. Huang, W. Li and S. Z. Yao, Electrophoresis, 2010, 31, 1055 CrossRef CAS.
- X. Liu, Y. Y. Zhang, J. P. Lei, Y. D. Xue, L. X. Cheng and H. X. Ju, Anal. Chem., 2010, 82, 7351 CrossRef CAS.
- X. Liu and H. X. Ju, Anal. Chem., 2008, 80, 5377 CrossRef CAS.
- G. F. Jie, J. J. Zhang, D. C. Wang, C. Cheng, H. Y. Chen and J. J. Zhu, Anal. Chem., 2008, 80, 4033 CrossRef CAS.
-
(a) I. Rohn, W. Künnecke and U. Bilitewski, Anal. Chem., 1995, 67, 2304 CrossRef CAS;
(b) T. L. Ma, T. Kida, M. Akiyama, K. Inoue, S. J. Tsunematsu, K. Yao, H. Noma and E. Abe, Electrochem. Commun., 2003, 5, 369 CrossRef CAS;
(c) C. A. Marquette, M. F. Lawrence and L. J. Blum, Anal. Chem., 2006, 78, 959 CrossRef CAS;
(d) H. Nakamura, K. Tohyama, M. Tanaka, S. Shinohara, Y. Tokunaga, F. Kurusu, S. Koide, M. Gotoh and I. Karube, Biosens. Bioelectron., 2007, 23, 621 CrossRef CAS;
(e) T. Schüler, T. Asmus, W. Fritzsche and R. Möller, Biosens. Bioelectron., 2009, 24, 2077 CrossRef CAS.
-
(a) M. H. Chiu, H. Wu, J. C. Chen, G. Muthuraman and J. M. Zen, Electroanalysis, 2007, 19, 2301 CrossRef CAS;
(b) C. A. Marquette, A. Degiuli and L. J. Blum, Biosens. Bioelectron., 2003, 19, 433 CrossRef CAS;
(c) A. Sassolas, L. J. Blum and B. D. Leca-Bouvier, Anal. Bioanal. Chem., 2008, 390, 865 CrossRef CAS;
(d) J. B. Claver, M. C. V. Mirón and L. F. Capitán-Vallvey, Analyst, 2009, 134, 1423 RSC;
(e) Z. M. Redha, S. J. Baldock, P. R. Fielden, N. J. Goddard, B. J. T. Brown, B. G. D. Haggett, R. Andres and B. J. Birch, Electroanalysis, 2009, 21, 422 CrossRef CAS.
-
(a) R. X. Zhang, L. Z. Fan, Y. P. Fang and S. H. Yang, J. Mater. Chem., 2008, 18, 4964 RSC;
(b) X. F. Wang, Y. Zhou, J. J. Xu and H. Y. Chen, Adv. Funct. Mater., 2009, 19, 1444 CrossRef CAS;
(c) Y. S. Guo, X. P. Jia and S. S. Zhang, Chem. Commun., 2011, 47, 725 RSC.
- Z. F. Ding, B. M. Quinn, S. K. Haram, L. E. Pell, B. A. Korgel and A. J. Bard, Science, 2002, 296, 1293 CrossRef CAS.
- X. Liu, L. X. Cheng, J. P. Lei, H. Liu and H. X. Ju, Chem.–Eur. J., 2010, 16, 10764 CrossRef CAS.
- C. W. Ge, M. Xu, J. Liu, J. P. Lei and H. X. Ju, Chem. Commun., 2008, 450 RSC.
- W. W. Yu, L. H. Qu, W. Z. Guo and X. G. Peng, Chem. Mater., 2003, 15, 2854 CrossRef CAS.
- Y. M. Fang, J. J. Sun, A. H. Wu, X. L. Su and G. N. Chen, Langmuir, 2009, 25, 555 CrossRef CAS.
-
D. R. Lide, CRC Handbook of Chemistry and Physics, 88th ed., CRC Press Inc., Boca Raton, 2008 Search PubMed.
-
(a) W. J. Li, R. Yuan and Y. Q. Chai, J. Phys. Chem. C, 2010, 114, 21397 CrossRef CAS;
(b) L. Rassaei and F. Marken, Anal. Chem., 2010, 82, 7063 CrossRef CAS;
(c) W. T. Shi and Z. F. Ma, Biosens. Bioelectron., 2010, 26, 1098 CrossRef CAS.
- A. A. M. Santafé, B. Doumèche, L. J. Blum, A. P. Girard-Egrot and C. A. Marquette, Anal. Chem., 2010, 82, 2401 CrossRef CAS.
|
This journal is © The Royal Society of Chemistry 2012 |
Click here to see how this site uses Cookies. View our privacy policy here.