Synergetic enhancement effect of room temperature ionic liquids and ammonium pyrrolidine dithiocarbamate on the chemical vapor generation of iron, cobalt and nickel in environmental and biological samples by atomic absorption spectrometric determination
Received
30th August 2011
, Accepted 19th October 2011
First published on 14th November 2011
Abstract
A new method based on chemical vapor generation (CVG) coupled with flame atomic absorption spectrometry (FAAS) was developed for the determination of iron, cobalt and nickel in environmental and biological samples. Room temperature ionic liquids (RTIL) combined with ammonium pyrroldinedithiocarbamate (APDC) were used to synergetically enhance the CVG of iron, cobalt and nickel. The addition of RTIL leads to 2.5, 3.2, 3.0 times improvement in the CVG efficiencies of Fe, Co and Ni, respectively. The efficiencies for the CVG of Fe, Co and Ni in the presence of RTIL and APDC were 42%, 61% and 54%, respectively. Volatile species of iron, cobalt and nickel were effectively generated through reduction of acidified analyte solutions with KBH4 in the presence of 0.02% or 0.01% (m/v) APDC and 0.1% or 0.2% (m/v) 1-butyl-3-methylimidazolium hexafluorophosphate ([C4MIM]PF6). Some parameters that influenced CVG and subsequent determination were evaluated in detail, such as the concentrations of RTIL, APDC, nitric acid (HNO3) and KBH4; flow rates of carrier gas, lengths of reaction tube and transfer tube, as well as interferences. Under optimized conditions, the detection limits (LODs) for Fe, Co and Ni were 18, 14 and 11 ng mL−1, respectively. Relative standard deviations for five replicate determinations of the standard solution containing 500 ng mL−1Fe, Co and Ni were 5.3%, 4.6% and 4.3%, respectively. The proposed method was successfully applied to the determination of iron, cobalt and nickel in certified environmental and biological reference materials with satisfactory results.
1. Introduction
The chemical vapor generation technique (CVG) has been widely used in atomic spectroscopy because of its distinguishing characteristics, such as efficient matrix separation, high transport efficiency, nearly complete analyte atomization, and potential speciation analysis as compared with conventional pneumatic nebulization.1–3 Elements that can generate volatile species upon derivatization with tetrahydroborate(III) are still few, which stimulates the development of new CVG systems for more elements. In recent years, the CVG technique has expanded its scope to transition and noble metals following reaction with tetrahydroborate(III), such as Cu, Fe, Co, Zn, Mn, Au, Ag, Pt, and Pd.4–20 However, the CVG efficiency of transition and noble metals is much lower compared with conventional hydride-forming elements, so great efforts have been made to improve the CVG efficiency of transition and noble metals in the past few years. Trace amounts of complexing agents were found to be helpful for improving the CVG efficiency of transition and noble metals, such as sodium diethyl-dithiocarbamate (DDTC) for Au,21–231,10-phenanthroline for Cu,248-hydroxyquinoline (8-HQ) for Zn and Cd,25cetyltrimethylammonium bromide (CTAB)for Zn,26thiourea, cobalt ion and ascorbic acid for Cd.27,28
Conventional CVG procedures for nickel involve the reaction of the analyte with carbon monoxide to generate volatile nickel carbonyl species.29–31 However, the extremely toxic nature of both carbon monoxide and nickel carbonyl largely restricts its wide applications. Generation of volatile nickel species through reduction in acidified sample solutions with sodium tetrahydroborate(III) aqueous solution was reported by Guo et al.,32 In the process, carbon monoxide was discarded and this opened a novel door for the CVG of Ni. The transport of iron, cobalt and nickel to the atom cell during a hydride generation process were also reported by Wickstrom and his co-workers.33 Recently, Pohl et al.34 found volatile species of nickel and cobalt during hydride generation, but the efficiency of CVG were quite low. Zheng et al.35 introduced an UV photochemical vapor generation for Ni and Fe using isotope dilution ICP-MS determination.
Room temperature ionic liquids (RTIL) have been applied in various fields of chemistry because of their fascinating properties, such as negligible vapor pressure, good thermal stability, tunable viscosity and good solubility for inorganic and organic compounds.36 These characteristics make ionic liquids suitable to be used as the extraction solvent for separation and preconcentration of various organic compounds and metal ions.37,38 Recently, RTIL was reported to enhance the CVG efficiency of Au (3.6 times), Ag (2.7 times) and Cu (4.8 times), and the enhancement mechanism was also studied.39,40 Yan et al.41 discovered the synergetic enhancement effect of ionic liquid and sodium diethyldithiocarbamate (DDTC) on the CVG of nickel. To the best of our knowledge, the synergetic enhancement effect of RTIL and APDC on the CVG of iron, cobalt and nickel for atomic absorption spectrometric determination in environmental and biological samples has not been reported yet.
The present work aimed to develop a new CVG system for iron, cobalt and nickel with synergetic enhancement effect of RTIL and APDC. The effect of concentrations of RTIL and APDC on the CVG of iron, cobalt and nickel, variables of the CVG and atomic absorption spectrometry, and interference of coexisting ions were investigated systematically. The proposed method possesses the advantages of low sample and reagent consumption, high sensitivity, good precision, fine selectivity. Furthermore, this method was successfully applied to quantify iron, cobalt and nickel in environmental and biological samples with satisfactory results.
2. Experimental
2.1. Instrumentation
A model AA-6601F flame atomic absorption spectrometer equipped with deuterium lamp background correction (Shimadzu Instrumental Co., Japan) was used for the determination of iron, cobalt and nickel. Hollow cathode lamps of iron, cobalt and nickel (Ningqiang Source, Hengshui, China) were used as the radiation source. The CVG system consisted of two peristaltic pumps (model HL-2, Huxi Analytical Instrumental Co., Shanghai, China) and a lab-made gas-liquid phase separator (GLS). Peristaltic pumps were used to deliver acidified sample and borohydride solutions to the reaction tube. The volatile species of iron, cobalt and nickel that evolved from the reaction tube were swept into a FAAS atomizer with an argon flow. The argon flow was controlled by a flowmeter in order to operate at low flow rate. PTFE tube (1 mm i.d) was used as both a transfer tube and reaction tube. The schematic diagram of the CVG-FAAS system is illustrated in Fig. 1.
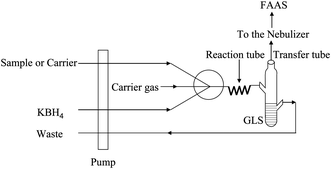 |
| Fig. 1 Schematic diagram of the CVG-FAAS system, GLS: gas/liquid separator. | |
2.2. Reagent and materials
The stock standard solutions of Fe(III), Co(II) and Ni(II) (1 mg mL−1) were purchased from the National Center for Reference Materials (Beijing, China). Working standard solutions were obtained by serial dilution of the stock standard solutions. All chemicals used were at least of analytical-reagent grade and all solutions were prepared with double distilled water (DDW). Ammonium pyrroldinedithiocarbamate (APDC) solution of 0.02% (m/v) was prepared by dissolving appropriate amounts of APDC in DDW. RTIL of 1-butyl-3-methylimidazolium hexafluorophosphate ([C4MIM]PF6) was purchased from Chengjie Chemistry Corporation (Shanghai, China). Diluted HNO3 solution was used as the carrier solution and also as the medium for subsequent CVG. KBH4 solution of 2.0% (m/v) was prepared by dissolving appropriate amounts of KBH4 in 0.5% (m/v) potassium hydroxide (KOH) solution. APDC, KBH4, HNO3 and KOH used in this experiment were all obtained from Jingmi Chemical Reagent Corporation (Nanning, China).
2.3. Procedure of CVG
The schematic diagram of the CVG-FAAS system was shown in Fig. 1. In step 1, two PTFE delivering tubes were put into the sample solution and KBH4 solution, respectively. In step 2, acidified sample solution and KBH4 solution were sucked for 10 s and the sample solution was stored in a sample storage coil. Then in step 3, the delivering tube for the sample solution was changed to the carrier solution. At the last step (step 4), sample solution was pushed by the carrier into the reaction coil to mix with the KBH4 solution for the CVG reaction. The generated volatile species of iron, cobalt and nickel were separated from the gas-liquid separator and swept into the FAAS atomizer with the aid of the carrier gas. The operating conditions of the instrument are summarized in Table 1.
Table 1 Recommended operating conditions
Parameter |
Settings |
|
|
Fe
|
Co
|
Ni
|
Lamp current (mA) |
12 |
12 |
12 |
Acetylene flow rate (L min−1) |
2.2 |
1.6 |
1.6 |
Air flow rate (L min−1) |
8.0 |
6.0 |
6.0 |
Spectral bandpass (nm) |
0.2 |
0.2 |
0.2 |
Radiation wavelength (nm) |
248.3 |
240.7 |
232.0 |
Concentration of HNO3 (v/v) |
1.5% |
1.5% |
1.5% |
Concentration of KBH4 (m/v) |
2.5% |
2.5% |
2.0% |
Concentration of RTIL (v/v) |
0.1% |
0.2% |
0.2% |
Carrier gas (argon) flow rate (mL min−1) |
100 |
150 |
150 |
Concentration of APDC (m/v) |
0.02% |
0.02% |
0.01% |
The following certified environmental and biological reference materials were purchased from Institute of Reference Materials of State of Environment Protection Agency (Beijing, China), which were employed to validate the developed CVG-FAAS method based on synergetic enhancement of RTIL and APDC: GBW(E) 080195 (water sample), GBW08607 (water sample), GSBZ50009-88 (water sample), GBW10016 (Tea), GBW07601a (Hair), GBW09101b (Hair), GBW07318(GSD-14) (Sediment), GBW07407(GSS-7) (Soil). For the determination of Ni, three certified reference water samples were appropriately diluted with DDW. Three biological reference materials and two environmental reference materials were used for the determination of Fe and Co respectively. Microwave digestion was chosen for decomposing the certified reference samples and the digestion procedure was based on a reference method.42 The residues were transferred into a 25 mL volumetric flask, appropriate amounts of RTIL, APDC and HNO3 were added and the solution was made up to volume with DDW.
3. Results and discussion
3.1. Effect of RTIL and APDC
The traditional CVG efficiency of transition and noble metals is much lower. Thus many enhanced reagents were used to improve CVG efficiency, such as complexing agents, surfactants, molecular compounds and metal ions etc.23,26,28RTIL were employed to improve the CVG efficiency of Au, Ag, Ni and Cu, and possible enhanced mechanisms were also reported.39–41 In the present work, the effects of individual APDC or the combination of APDC and RTIL on the CVG of Fe, Co and Ni were investigated in detail. The concentration of APDC was very important to the CVG of Fe, Co and Ni. The effect of the concentration of APDC on the CVG of 500 ng mL−1Fe, Co and Ni in the absence of RTIL was studied. The results are shown in Fig. 2. It can be seen that the optimum concentration of APDC was 0.02% (m/v) for Fe and Co, 0.01% (m/v) for Ni.
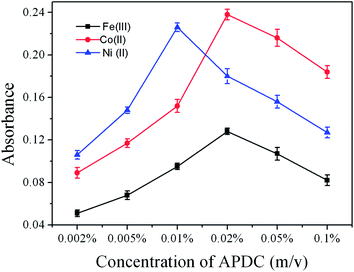 |
| Fig. 2 Effect of concentration of APDC on the absorbance of Fe(III), Co(II) and Ni(II) (500 ng mL−1 each) in the absence of RTIL. All other conditions as in Table 1. | |
The role of the RTIL can be assumed to inhibit further coalescence processes and hence prevent the loss of the nanoparticles in the CVG of transition and noble metals. Thus, the RTIL may act as a stabilizing agent in the CVG system. Some nanoparticles may be produced in the process of CVG of Fe, Co and Ni. The mechanisms of the effect of RTIL on the CVG of Fe, Co and Ni may be the same as those reported by Yan and his co-workers.39,401-Butyl-3-methylimidazolium hexafluorophosphate was used to enhance the CVG of Fe, Co and Ni in this experiment. The effects of the concentration of RTIL on the CVG of 500 ng mL−1Fe, Co and Ni in the presence of 0.02% (m/v) or 0.01% (m/v) APDC were studied. Results are shown in Fig. 3. The optimum concentration of RTIL was found to be 0.2% (v/v) for Co(II) and Ni(II), 0.1% (v/v) for Fe(III). Absorbances of 500 ng mL−1Fe(III), Co(II) and Ni(II) in the presence of RTIL were compared with those in the absence of RTIL (Fig. 2). The signal intensity was improved 2.5, 3.2, 3.0 times in the CVG for Fe(III), Co(II) and Ni(II) respectively. Based on the results in Fig. 2 and 3, the combination of APDC and RTIL created a synergetic enhancement effect on the CVG of Fe, Co and Ni. Therefore, 0.02% or 0.01% (m/v) APDC in combination with 0.1% or 0.2% (v/v) RTIL, were used to improve the CVG of Fe(III), Co(II) and Ni(II) in this work.
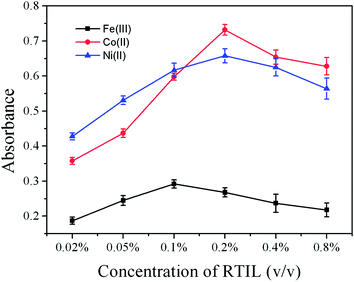 |
| Fig. 3 Effect of the concentration of RTIL on the absorbance of Fe(III), Co(II) and Ni(II) (500 ng mL−1 each) in the presence of 0.02% (m/v) or 0.01% (m/v) APDC. All other conditions as in Table 1. | |
3.2.
CVG variables
The CVG efficiency was highly dependent on the reaction acidity and acid types. A preliminary comparison between HCl, HNO3 and H2SO4 showed that HNO3 was the best medium for the CVG of Fe(III), Co(II) and Ni(II). The effect of the concentration of HNO3 on the CVG of Fe(III), Co(II) and Ni(II) was studied in the range of 0.5% to 3.0% (v/v). As can be seen from Fig. 4, the absorbances of Fe(III), Co(II) and Ni(II) were all optimal at the HNO3 concentration of 1.5% (v/v). Therefore, 1.5% (v/v) HNO3 was selected for further experiments. The acid concentration in the carrier solution was the same as that in the sample solution.
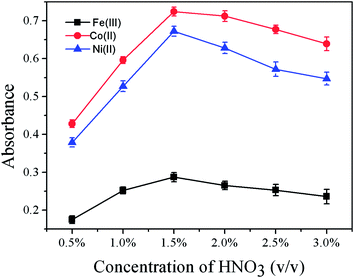 |
| Fig. 4 Effect of concentration of HNO3 on the absorbance of Fe(III), Co(II) and Ni(II) (500 ng mL−1 each). All other conditions as in Table 1. | |
The concentration of KBH4 had a significant impact on the CVG of Fe(III), Co(II) and Ni(II). A series of KBH4 concentrations in the range of 1.0% to 4.0% (m/v) were used. The optimum concentration of KBH4 was 2.5% (m/v) for Fe(III) and Co(II), 2.0% (m/v) for Ni(II). Lower concentrations of KBH4 probably gave incomplete reduction of the analytes. However, higher concentrations of KBH4 produced excessive hydrogen, brought about an unstable acetylene-air flame, and increased the instability of the signals. Thus, 2.5% (m/v) KBH4 for Fe(III) and Co(II), 2.0% (m/v) KBH4 for Ni(II) were selected for further experiments.
3.3.
AAS instrumental variables
The volatile species of Fe(III), Co(II) and Ni(II) were highly unstable and fast gas-liquid separation should be a prerequisite. The effect of carrier flow rate on the CVG of Fe(III), Co(II) and Ni(II) was studied in the range from 50 to 300 mL min−1. The optimum carrier flow rates were 150 mL min−1 for Co(II) and Ni(II), 100 mL min−1 for Fe(III). At low flow rates, the volatile species would not efficiently reach the atomizer due to adsorption and coalescence of nanoparticles. However, at high carrier gas flow rate, the dilution of the volatile species would result in the decrease of the signal intensity. Therefore, a flow rate of 150, 150 and 100 mL min−1 was used throughout for Co(II), Ni(II) and Fe(III), respectively.
The length of the reaction tube had an important influence on the CVG of Fe(III), Co(II) and Ni(II), as it was a compromise between the sampling amount and fast gas-liquid separation. The length of the reaction tube was studied in the range of 8 to 18 cm. The results are shown in Fig. 5, 12 cm was found to be optimal. The length of the transferring tube between the gas-liquid separator and the nebulizer was also carefully studied. As can be seen from Fig. 6, the optimal signal intensity was found at 7 cm. Guo et al.32 pointed out that nickel volatile species may be decomposed during transportation and the resultant Ni atoms might be adsorbed on the active sites of the inner wall surface of the transferring tube, thus the length of the transferring tube should be kept as short as possible. However, in this work, we found that if the transferring tube was too short, the signal intensity would be decreased. Thus, the length of the transferring tube was selected at 7 cm.
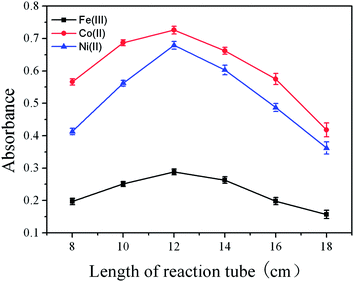 |
| Fig. 5 Effect of length of reaction tube on the absorbance of Fe(III), Co(II) and Ni(II) (500 ng mL−1 each). All other conditions as in Table 1. | |
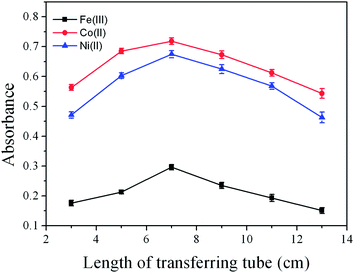 |
| Fig. 6 Effect of length of transferring tube on the absorbance of Fe(III), Co(II) and Ni(II) (500 ng mL−1 each). All other conditions as in Table 1. | |
3.4. Evaluation of interference
In this work, several metal and metalloid ions were selected for evaluation of the selectivity of the proposed method. Potential interfering ions Zn(II), Mn(II), Cu(II), Au(I), Ag(I), Bi(III), Pb(II), Cd(II), Ge(IV), Sn(II), Sb(III), As(III) and Se(IV) on the determination of 500 ng mL−1Fe(III), Co(II) and Ni(II) were examined in the presence of 0.1% (v/v) or 0.2% (v/v) RTIL and 0.02% (m/v) or 0.01% (m/v) APDC. All other conditions are shown in Table 1. The interferences from Fe(III), Co(II) and Ni(II) on each other were also tested. The results are shown in Table 2. All studied coexisting ions were found not to affect the determination of the CVG of Fe(III), Co(II) and Ni(II) when the concentration of coexisting ions was less than 30-fold that of Fe(III), Co(II) and Ni(II). Therefore, the selectivity of the proposed method was fairly satisfactory.
Table 2 Interference from co-existing ions on the chemical vapor generation of Fe(III), Co(II) and Ni(II) (500 ng mL−1)
Co-existing ions |
[M]/[Fe(III)] |
Recovery (%, mean ± S.D., n = 3) |
[M]/[Co(II)] |
Recovery (%, mean ± S.D., n = 3) |
[M]/[Ni(II)] |
Recovery (%, mean ± S.D., n = 3) |
Zn(II)
|
100 |
98 ± 1 |
50 |
92 ± 2 |
500 |
95 ± 2 |
Mn(II)
|
200 |
96 ± 2 |
100 |
95 ± 1 |
500 |
94 ± 1 |
Cu(II)
|
100 |
91 ± 2 |
100 |
97 ± 2 |
200 |
91 ± 1 |
Au(I)
|
50 |
95 ± 3 |
50 |
90 ± 1 |
200 |
98 ± 3 |
Ag(I)
|
100 |
96 ± 1 |
50 |
92 ± 3 |
200 |
93 ± 1 |
Bi(III)
|
100 |
93 ± 1 |
50 |
96 ± 1 |
50 |
95 ± 2 |
Pb(II)
|
50 |
97 ± 2 |
50 |
90 ± 5 |
50 |
99 ± 2 |
Cd(II)
|
50 |
99 ± 4 |
50 |
94 ± 1 |
100 |
91 ± 1 |
Ge(IV)
|
100 |
95 ± 1 |
100 |
93 ± 3 |
50 |
97 ± 4 |
Sn(II)
|
100 |
90 ± 1 |
100 |
96 ± 2 |
50 |
92 ± 3 |
As(III)
|
50 |
98 ± 3 |
50 |
97 ± 2 |
50 |
93 ± 2 |
Sb(III)
|
100 |
96 ± 2 |
50 |
91 ± 3 |
50 |
98 ± 1 |
Se(IV)
|
50 |
95 ± 1 |
50 |
92 ± 2 |
50 |
97 ± 2 |
Fe(III)
|
— |
— |
30 |
98 ± 5 |
50 |
93 ± 2 |
Co(II)
|
30 |
95 ± 2 |
— |
— |
50 |
91 ± 4 |
Ni(II)
|
50 |
98 ± 4 |
30 |
90 ± 2 |
— |
— |
3.5. Analytical performance and application
The figures of merit for the developed CVG-FAAS method for the determination of Fe, Co and Ni based on the synergetic enhancement effect of RTIL and APDC were evaluated. Under the optimal experimental conditions, the calibration curve was linear in the range of 20 to 2000 ng mL−1 for Fe (R2 > 0.991), 10 to 800 ng mL−1 for Co (R2 > 0.999) and 10 to 800 ng mL−1for Ni (R2 > 0.998). The limits of detection for Fe, Co and Ni were 18, 14 and 11 ng mL−1, respectively, which were obtained on the basis of 3σ criterion for eleven replicated measurements of the blank signal. Relative standard deviations for five replicate determinations of the standard solution containing 500 ng mL−1Fe, Co and Ni were 5.3%, 4.6% and 4.3%, respectively.
To validate the potential application of the proposed method, eight certified reference materials (CRMs), GBW(E) 080195 (Water sample), GBW08607 (Water sample), GSBZ50009-88 (Water sample), GBW10016 (Tea), GBW07601a (Hair), GBW09101b (Hair), GBW07318(GSD-14) (Sediment), GBW07407(GSS-7) (Soil) were analyzed for Fe, Co and Ni determination. The analytical results are shown in Table 3. The found values in these CRMs by the proposed CVG-FAAS method based on the synergetic enhancement effect of RTIL and APDC were in good agreement with the certified values.
Table 3 Analytical results for Fe, Co and Ni in reference materials. (mean ± S.D., n = 3)
Sample |
Element |
Found value |
Certified value |
GBW10016 (Tea) |
Fe
|
238 ± 15 (μg g−1) |
242 ± 18 (μg g−1) |
GBW07601a (Hair) |
Fe
|
34 ± 4 (μg g−1) |
36 ± 5 (μg g−1) |
GBW09101b (Hair) |
Fe
|
158 ± 18 (μg g−1) |
160 ± 16 (μg g−1) |
GBW07318(GSD-14) (Sediment) |
Co
|
27 ± 3 (μg g−1) |
28 ± 2 (μg g−1) |
GBW07407(GSS-7) (Soil) |
Co
|
92 ± 8 (μg g−1) |
97 ± 6 (μg g−1) |
GBW(E)080195 (Water sample) |
Ni
|
471 ± 25 (ng mL−1) |
476 ± 30 (ng mL−1) |
GBW08607 (Water sample) |
Ni
|
509 ± 10 (ng mL−1) |
515 ± 6 (ng mL−1) |
GSBZ50009-88 (Water sample) |
Ni
|
990 ± 50 (ng mL−1) |
997 ± 48 (ng mL−1) |
4. Conclusion
1-Butyl-3-methylimidazolium hexafluorophosphate and APDC were introduced in the CVG-FAAS method, and the CVG efficiencies of Fe, Co and Ni were improved greatly under the synergetic enhancement effect. The efficiencies for the CVG of Fe, Co and Ni in the presence of RTIL and APDC were 42%, 61% and 54%, respectively. The addition of RTIL leads to 2.5, 3.2, 3.0 times improvement in the CVG efficiencies of Fe, Co and Ni, respectively. The developed method offers the merits of high sensitivity, good accuracy, rapidity, simplicity, ease of use, low cost, and wide practicability.
Acknowledgements
The authors gratefully acknowledge the financial support for this project from the Foundation of Education of Guangxi (200911MS204) and Foundation of Doctor of Yulin Normal College (G2010004).
References
- R. E. Sturgeon and Z. Mester, Appl. Spectrosc., 2002, 56, 202A–213A CrossRef CAS.
- R. E. Sturgeon, X. Guo and Z. Mester, Anal. Bioanal. Chem., 2005, 382, 881–883 CrossRef CAS.
- Y. H. He, X. D. Hou, C. B. Zheng and R. E. Sturgeon, Anal. Bioanal. Chem., 2007, 388, 769–774 CrossRef CAS.
- P. Pohl and W. Zyrnicki, J. Anal. At. Spectrom., 2002, 17, 746–749 RSC.
- P. Pohl and W. Zyrnicki, J. Anal. At. Spectrom., 2001, 16, 1442–1445 RSC.
- Y. L. Feng, R. E. Sturgeon and J. W. Lam, J. Anal. At. Spectrom., 2003, 18, 1435–1442 RSC.
- X. C. Duan, R. L. McLaughlin, I. D. Brindle and A. Conn, J. Anal. At. Spectrom., 2002, 17, 227–231 RSC.
- A. S. Luna, R. E. Sturgeon and R. C. de Campos, Anal. Chem., 2000, 72, 3523–3531 CrossRef CAS.
- Y. L. Feng, J. W. Lam and R. E. Sturgeon, Analyst, 2001, 126, 1833–1837 RSC.
- L. He, X. F. Zhu, L. Wu and X. D. Hou, Atom. Spectrosc., 2008, 29, 93–98 CAS.
- P. Pohl, TrAC, Trends Anal. Chem., 2004, 23, 87–101 CrossRef CAS.
- P. Pohl and B. Prusisz, Anal. Bioanal. Chem., 2007, 388, 753–762 CrossRef CAS.
- T. Matousek, Anal. Bioanal. Chem., 2007, 388, 763–767 CrossRef.
- P. Pohl and W. Zyrnicki, J. Anal. At. Spectrom., 2003, 18, 798–801 RSC.
- Y. Arslan, T. Matousek, J. Kratzer, S. Musil, M. Vobecky, O. Y. Ataman and J. Dedina, J. Anal. At. Spectrom., 2011, 26, 828–837 RSC.
- S. Musil, J. Kratzer, M. Vobecky, O. Benada and T. Matousek, J. Anal. At. Spectrom., 2010, 25, 1618–1626 RSC.
- H. Matusiewicz and M. Slachcinski, Spectrosc. Lett., 2010, 43, 172–182 CrossRef CAS.
- H. Matusiewicz and M. Slachcinski, J. Anal. At. Spectrom., 2010, 25, 1324–1333 RSC.
- J. Villanueva-Alonso, E. Pena-Vazquez and P. Barmejo-Barrera, Spectrochim. Acta, Part B, 2009, 64, 659–665 CrossRef.
- E. Pena-Vazquez, J. Villanueva-Alonso and P. Barmejo-Barrera, J. Anal. At. Spectrom., 2007, 22, 642–649 RSC.
- X. G. Du and S. K. Xu, Fresenius J. Anal. Chem., 2001, 370, 1065–1070 CrossRef CAS.
- H. B. Ma, X. F. Fan, H. Y. Zhou and S. K. Xu, Spectrochim. Acta, Part B, 2003, 58, 33–41 CrossRef.
- Z. X. Li, J. Anal. At. Spectrom., 2006, 21, 435–438 RSC.
- H. Y. Zhou, S. K. Xu and Z.L., Spectrosc. Spectr. Anal., 2000, 20, 525–528 CAS.
- H. W. Sun and R. Suo, Anal. Chim. Acta, 2004, 509, 71–76 CrossRef CAS.
- H. W. Sun, R. Suo and Y. K. Lu, Anal. Chim. Acta, 2002, 457, 305–310 CrossRef CAS.
- X. W. Guo and X. M. Guo, Anal. Chim. Acta, 1995, 310, 377–385 CrossRef CAS.
- Y. K. Lu, H. W. Sun, C. G. Yuan and X. P. Yan, Anal. Chem., 2002, 74, 1525–1529 CrossRef.
- R. E. Sturgeon, S. N. Willie and S. S. Berman, J. Anal. At. Spectrom., 1989, 4, 443–446 RSC.
- C. J. Park and S. A. Yim, J. Anal. At. Spectrom., 1999, 14, 1061–1065 RSC.
- B. Dočekal, P. Marek and M. Vobecký, J. Anal. At. Spectrom., 2000, 15, 131–135 RSC.
- X. M. Guo, B. L. Huang, Z. H. Sun, R. Q. Ke, Q. Q. Wang and Z. B. Gong, Spectrochim. Acta, Part B, 2000, 55, 943–950 CrossRef.
- T. Wickstrom, W. Lund and R. Bye, Analyst, 1996, 121, 201–204 RSC.
- P. Pohl and W. Zyrnicki, Anal. Chim. Acta, 2001, 429, 135–143 CrossRef CAS.
- C. B. Zheng, L. Yang, R. E. Sturgeon and X. D. Hou, Anal. Chem., 2010, 82, 3899–3904 CrossRef CAS.
- F. Endres and S. Z. E. Abedin, Phys. Chem. Chem. Phys., 2006, 8, 2101–2116 RSC.
- R. Liu, J. F. Liu, Y. G. Yin, X. L. Hu and G. B. Jiang, Anal. Bioanal. Chem., 2009, 393, 871–883 CrossRef CAS.
- Z. Y. Li, Y. C. Pei, H. Y. Wang, J. Fan and J. J. Wang, TrAC, Trends Anal. Chem., 2010, 29, 1336–1346 CrossRef CAS.
- C. Zhang, Y. Li, X. Y. Cui, Y. Jiang and X. P. Yan, J. Anal. At. Spectrom., 2008, 23, 1372–1377 RSC.
- C. Zhang, Y. Li, P. Wu, Y. Jiang, Q. Liu and X. P. Yan, Anal. Chim. Acta, 2009, 650, 59–64 CrossRef CAS.
- C. Zhang, Y. Li, P. Wu and X. P. Yan, Anal. Chim. Acta, 2009, 652, 143–147 CrossRef CAS.
- Y. P. Ke, Q. Sun, Z. R. Yang, J. J. Xin, L. Chen and X. D. Hou, Spectrosc. Lett., 2006, 29, 29–43 CrossRef.
|
This journal is © The Royal Society of Chemistry 2012 |
Click here to see how this site uses Cookies. View our privacy policy here.