DOI:
10.1039/C1RA00420D
(Paper)
RSC Adv., 2012,
2, 453-460
Role of microorganism growth phase in the accumulation and characteristics of biomacromolecules (BMM) in a membrane bioreactor†
Received
7th July 2011
, Accepted 15th September 2011
First published on 8th November 2011
Abstract
The objective of this study was to highlight the significance of microorganism growth on the production of biomacromolecules (BMM) in a membrane bioreactor (MBR). During the MBR operation, both polysaccharides and proteins in the sludge supernatant were found to increase steadily in exponential growth phase (EGP) due to higher organic loading rates and microbial primary metabolism. Subsequently, both increased continuously and then decreased sharply in the following deceleration growth phase (DGP). Finally, the BMM maintained a low and steady level as the sludge reached stationary growth phase (SGP). The results of batch experiments showed that the sludge under DGP was prone to produce much more BMM than that under SGP as a result of a nutrients decrease and higher microbial secondary metabolism activities. Furthermore, large-molecular weight (MW) compounds (>100 kDa) made up the majority of BMM in the EGP and DGP. In contrast, the small-MW compounds (<5 kDa) became a more and more important BMM fraction as the microorganism growth shifted to the SGP. The characterization by three-dimensional excitation-emission matrix (EEM) fluorescence spectroscopy indicated that the fluorescence compounds in the BMM pool were mainly comprised of aromatic and tryptophan protein-like substances, and humic substances. The protein-like substances were related with microorganism growth more greatly than the humic substances. Generally, the microorganism physiological stages (i.e.growth/decay) play a crucial role in the formation of BMM in the MBRs.
1. Introduction
Biomacromolecules (BMM), representing colloidal and soluble microbial products, are mainly composed of a pool of complex organic compounds of microbial origin such as polysaccharides, proteins and humic substances. They can be further classified into two categories based on microorganism growth:1 substrate utilization-associated-products (UAP) which are produced during substrate metabolism, and biomass-associated-products (BAP) which are usually produced due to hydrolysis of bound extracellular polymeric substances (EPS) under substrate deficiency. BMM often plays an important role in the fouling development of membrane bioreactors (MBRs), particularly responsible for irreversible fouling.2–4
In view of the significance of BMM for membrane fouling,5–11 various operating conditions have been identified to affect BMM production/elimination in MBRs.12 For example, Jin et al.13 showed that keeping higher dissolved oxygen (DO) concentrations (> 3.0 mg L−1) in the MBR would be helpful for the control of BMM. A low operating temperature, such as in wintertime, can enable more BMM production , e.g., ref. 14. In particular, the quantity and quality of BMM are also impacted by solid retention times (SRTs). Ng et al.15 found that proteins, polysaccharides, and total organic carbon in the sludge supernatant decreased as SRTs increased from 3 to 5, 10, and 20 days. Similar results were also reported by Malamis and Andreadakis.16 Essentially, the change of operating conditions (e.g., temperature, feedwater loading rate, SRTs) will disturb or alter the microorganism metabolism activities or physiological stages (i.e., growth/decay). In fact, the sludge with a short SRT has higher metabolism activities than that with a long SRT. As such, microorganism growth is more significant under short SRT operation modes; however, decay occurs more often under long SRTs.15 Some studies have reported that a short SRT was involved in severe membrane fouling due to the production of high concentrations of bound EPS and BMM.17–19 Correspondingly, a long SRT is expected to reduce membrane fouling due to the microbial utilization of the BMM. However, the mechanisms underlying the production/elimination of bound EPS and BMM in sludge supernatant have not been understood well in a MBR, and especially little attention has been paid to the role of microbial growth stages in the process. Thus, microorganism metabolism activities or growth/decay stages as key factors determining BMM formation should be investigated thoroughly.
Previous studies regarding BMM formation by microorganisms under different growth phases were based on short-term batch tests and pure bacteria cultures. For example, a batch study showed that polysaccharides in free EPS greatly decreased as the growth phase progressed from exponential phase to stationary phase.20 Similarly, Eboigbodin et al.21 reported that the BMM produced by Escherichia coli depended on both substrate types and its growth stages. Additionally, Badireddy et al.22,23 also found the differences in protein secondary structures and functionalized carbohydrates of bound EPS between exponential phase and stationary phase. Indeed, the results obtained from these studies with batch feeding mode can to some extent provide valuable information with respect to the production of BMM in the course of microorganism growth. Nevertheless, most of the MBRs are usually operated with continuous feeding mode and with mixed bacteria populations. So far, there is no investigation that elucidates how BMM are produced during different microorganism growth phases in open systems like MBRs; which, however, is of high significance for understanding the mechanisms underlying BMM formation in such continuously fed processes. A detailed investigation with a focus on the role of microorganism growth will be expected to provide novel insights into BMM formation mechanisms in MBRs.
Therefore, the main objective of this study is to understand the role of microorganism growth in the accumulation and characteristics of BMM based on the long-term MBR experiment. As mentioned above, to observe the role of microorganism growth rather than decay in BMM formation more clearly, a MBR operated with a short SRT of 5 days was investigated in this work. Specifically, the concentration and molecular weight (MW) of BMM produced by the sludge under different growth phases were analyzed. The chemical constituents of BMM compounds were characterized by three-dimensional excitation-emission matrix (EEM) fluorescence spectroscopy. The production potential of BMM was investigated by a series of batch experiments.
2. Experimental section
2.1
MBR operation
An aerobic lab-scale MBR with an effective volume of 5.5 L was studied (see Fig. S1 of the ESI†). A 0.1 m2 hollow fiber membrane module (PVDF, 0.01 μm, Litree Corp., China) was submerged in the MBR. Chemical cleaning on membranes was performed once the trans-membrane pressure (TMP) reached 0.025 MPa. Hydraulic retention time (HRT), and SRT of the MBR were set at 5.5–6 h and 5 days, respectively; that is, the membrane flux was kept at around 10 L/(m2 h) and 1.1 L of excess activated sludge mixed liquor was discharged per day. During the whole study, the DO concentration of the sludge was maintained at 4–5 mg L−1 by controlling aeration rate at approx. 150 L h−1. In addition, the MBR was operated in an air-conditioned room, where the temperature was kept at 20 ± 1 °C. A complex synthetic wastewater simulating municipal wastewater (see Table S1 of the ESI†), was used as feedwater to ensure the consistent quality of the influent and thus a steady microbial community was maintained in the MBR. Trace mineral nutrients were added for the optimum growth of activated sludge. Prior to this study, the MBR with an initial volatile suspended solids (VSS) of around 1200 mg L−1 was operated at the above mentioned operating conditions for more than one month to acclimatise the seed sludge until the VSS concentration and the MBR performance reached stable levels. After that, part of the sludge was discharged, and the MBR was operated again at the initial VSS concentration of approx. 1200 mg L−1 for about 2 months to study the formation of BMM over the microorganism growth process. During the MBR operation, the average removal efficiencies of chemical oxygen demand (COD) and ammonia nitrogen (NH4+-N) reached 91% and 68%, respectively (see Fig. S2 of ESI†).
2.2 Batch tests for the study of BMM production potential
The microorganisms under different growth stages may have a different BMM production potential. In this study, the production potential of BMM was investigated by BAP generation. The UAP was not studied for the following reasons: (1) UAP only predominate BMM production when substrate loading rate is very high;24 (2) the production rate of UAP is proportional to the substrate consume rate; that is, the production rate of UAP in the MBRs can be considered as steady because the MBRs are operated with a continuous feeding mode; (3) crucially, the UAP seem to be readily biodegraded to small-MW compounds so that they can be discharged constantly from the MBR.24 In view of these issues, a series of batch tests under starved conditions (i.e., without substrate addition) were performed on the 1st, 4th, 9th, 15th, 37th, 50th, and 57th day of the MBR operation to explore the BAP production/degradation during different microorganism growth phases. Approximately 200-300 mL of activated sludge was taken from the MBR, and diluted to 1 L with deionized water and then transferred to a batch reactor. The DO concentration and temperature of the batch reactor were the same as the lab-scale MBR. The pH was always kept at in the range 7.0–7.5. During the batch tests, proteins (Pn) and polysaccharides (Ps) of the supernatant were analyzed at 0 min (before starting the batch test), 16 h, 24 h, 40 h, 52 h, 64 h, and 72 h. The net content of BAP produced by per gram biomass was calculated as following: | 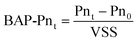 | (1) |
| 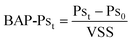 | (2) |
where, BAP-Pnt/BAP-Pst are the Pn/Ps content of BAP produced by per gram biomass at time of t (mg g−1-VSS), VSS is the concentration of the mixed liquid volatile suspended solids (g L−1), Pnt/Pst and Pn0/Ps0 are the Pn/Ps concentrations in the supernatant at time of t and 0 min (mg L−1), respectively.
2.3 Measurement of molecular weight (MW) distribution
The MW distribution of BMM, which was pre-filtrated with a filter paper, was determined by filtering the samples through a series of polyethersulfone membranes with different molecular weight cutoffs (MWCO) (0.45 μm, 100 kDa, 30 kDa, 5 kDa). The filtration was performed in a stirred dead-end membrane filtration cell (MSC300, Mosu corp., Shanghai, China). As such, five fractions of BMM compounds can be obtained: larger than 0.45 μm, 0.45 μm-100 kDa, 100–30 kDa, 30–5 kDa, smaller than 5 kDa. The concentration of polysaccharides and proteins in each fraction were monitored.
2.4 Chemical analysis
The extraction of bound EPS from sludge flocs was performed with cation exchange resin (Na-form, Amberlite IR-120, USA) according to the method of Frølund et al.25 All the samples (i.e. the feedwater, the effluent and sludge supernatant) were filtered through a coarse filter for polysaccharide and protein analysis. This was due to the fact that the colloidal and soluble organic material are the major BMM components affecting membrane fouling.8 The polysaccharides and proteins were quantified according to ref. 26,27. The measurements of polysaccharide and protein were performed in duplication, and the average value was given with standard deviations smaller than 5%. The influence of nitrate and nitrite on polysaccharide measurements was corrected. The concentrations of COD, NH4+-N, and VSS were measured according to the Chinese NEPA standard methods.28EEM spectra of BMM were analyzed by fluorescence spectrometry (F-4500 FL spectrophotometer, Hitachi, Japan). The use of the EEM technique was similar to that presented by Huang et al.29 Volumetric integration of the EEM peaks was performed according to the method of Chen et al.,30 to calculate the content of a given BMM component semi-quantitatively. The volumetric proportion of a given EEM peak in one spectrum was described using fluorescence regional integration (FRI).
3. Results
3.1 Influence of microorganism growth on BMM accumulation
Fig. 1a shows the variation of the concentrations of polysaccharides, proteins, and VSS in the mixed liquor over the entire operating process. According to the development of VSS concentrations, a typical microorganism growth curve with three distinctive phases can be observed: (I) exponential growth phase (EGP, 0–5 days), (II) deceleration growth phase (DGP, 6–20 days), and (III) stationary growth phase (SGP, 21–57 days). As the sludge had been acclimatised for over one month, no lag growth phase was found in the curve. At the beginning of the MBR operation, the bioreactor had a lower VSS of approx. 1200 mg L−1. As such, the microorganisms involved in the bioreactor were exposed to a higher organic loading rate (see Table 1), leading to a higher microorganism growth rate in the initial operating time. However, the microorganisms were gradually subject to an increase of substrate limitation because of the increasing sludge concentration, which subsequently resulted in the slowdown of the microorganism growth rate (i.e., DGP) and an SGP was finally reached (i.e. the microorganism growth was balanced with sludge decay and sludge discharge). Interestingly, it was found that concentrations of both polysaccharides and proteins increased steadily in the EGP. In the DGP, however, their concentrations increased rapidly (from day 5 to day 10); subsequently, both of them declined sharply (i.e., from day 11 to day 20). Additionally, it is found that the polysaccharides had a much higher level than proteins, particularly in the operating time from day 11 to day 20. In the SGP, the BMM compounds were found to have a low and steady level. Similarly, Masse et al.19 also observed the same accumulation trend of soluble COD in a submerged MBR (SRT = 37.2 days) for real domestic wastewater treatment. Likewise, Gao et al.31 applied a SRT of 210 days in a MBR to treat the synthetic ammonia-bearing inorganic wastewater and also observed the transient accumulation of TOC in the sludge supernatant. Apparently, the concentrations of BMM strongly depend on microorganism growth. Moreover, the change of bound EPS were also found to rely on microorganism growth, e.g., more and more biopolymers were stored by the microorganisms during their growth (see Table 1). Importantly, the rapid proliferation of microorganisms enabled the polysaccharides to be stored more rapidly than the proteins. The results of Omoike et al.20 also revealed that polysaccharides were enriched during the exponential growth phase.
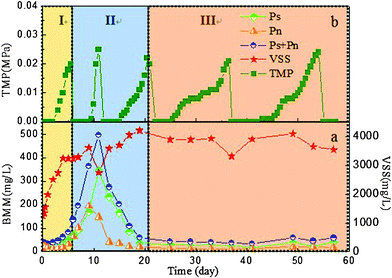 |
| Fig. 1 Evolution of BMM/VSS concentrations (a) and TMP (b) over operating time. | |
Table 1
Polysaccharides (Ps) and proteins (Pn) of influent, sludge supernatant, effluent, and bound-EPS, and the rejection degree of the BMM, and the organic loading rate of microorganisms in the different growth phasesa
|
Exponential growth phase (EGP) (0–5 days) |
Deceleration growth phase (DGP) (6–20 days) |
Stationary growth phase (SGP) (21–57 days) |
Pn |
Ps |
Ps/Pn |
Pn |
Ps |
Ps/Pn |
Pn |
Ps |
Ps/Pn |
Rejection degree = (BMMsupernantant-BMMpermeate)/BMMsupernatant, and all the values of the different growth phase were given in average values and standard deviations.
|
Influent (mg L−1) |
68.4 ± 4.8 |
245.3 ± 21.0 |
3.6 ± 0.4 |
63.0 ± 4.2 |
221 ± 28.8 |
3.5 ± 0.4 |
60.6 ± 4.4 |
199.6 ± 32.1 |
3.0 ± 1.1 |
Supernatant (mg L−1) |
20.6 ± 6.0 |
23.3 ± 14.2 |
1.1 ± 0.6 |
78.5 ± 59.3 |
150.6 ± 95.6 |
2.6 ± 1.7 |
15.1 ± 3.8 |
27.5 ± 3.7 |
1.9 ± 0.5 |
Effluent (mg L−1) |
14.8 ± 3.0 |
3.5 ± 2.0 |
0.23 ± 0.11 |
14.4 ± 4.7 |
4.6 ± 5.1 |
0.29 ± 0.22 |
11.2 ± 2.6 |
6.3 ± 1.2 |
0.57 ± 0.08 |
Rejection degree (%) |
24.7 ± 15.3 |
79.1 ± 16.5 |
— |
68.5 ± 21.7 |
96.6 ± 2.4 |
— |
23.8 ± 14.2 |
77.2 ± 2.5 |
— |
Bound-EPS(mg g−1-VSS) |
34.0 ± 6.5 |
15.7 ± 4.6 |
0.47 ± 0.13 |
39.5 ± 6.4 |
14.0 ± 6.1 |
0.34 ± 0.11 |
30.1 ± 7.7 |
7.3 ± 1.8 |
0.25 ± 0.04 |
Loading rate(g g−1-VSS d) |
0.85 ± 0.34 |
3.01 ± 1.18 |
— |
0.43 ± 0.06 |
1.49 ± 0.21 |
— |
0.39 ± 0.04 |
1.28 ± 0.24 |
— |
Fig. 1b shows the development of TMP of the membranes during the MBR operation. The filtration cycles were determined as 6, 5, 10, 16, and 17 days, respectively. It suggested that there was a much higher membrane fouling rate in the EGP and DGP The coincident evolution trend of BMM accumulation rate and TMP increasing rate implies that BMM concentration might be a potential fouling factor affecting membrane fouling. Furthermore, the large MW of BMM in the exponential and transitional phases was another reason leading to the severe membrane fouling (see the following sections).
3.2 Influence of microorganism growth on the size nature of BMM compounds
Global characteristics such as MW of BMM compounds are of particular concern for understanding the production/degradation mechanism and fouling propensity of BMM in MBRs. During membrane filtration, BMM compounds with large-MW tend to be rejected by the membranes and consequently accumulate on the membranes. In contrast, the small-MW fractions are prone to pass the membranes and present in the MBR permeate. To have a better understanding of the role of microorganism growth on BMM characteristics, the MW of BMM was also regularly measured (see Fig. 2). Here, the BMM compounds larger than 0.45 μm were an indicator of the presence of colloids or supramolecules which are aggregates of two or more molecular entities held together by intermolecular forces. Roughly, the proteins were mainly composed of colloids and small-MW substances (<5 kDa), and the majority of polysaccharides consisted of compounds larger than 100 kDa and those smaller than 5 kDa. It is interesting to note that the proteins on day 1 were mainly made up of small-MW compounds (e.g., more than 50% of proteins had an MW less than 5 kDa), and no colloidal proteins could be detected. It is possible that the small-MW compounds in the initial operation could derive from feedwater, because at that time the sludge had a higher loading rate and the feedwater composed of small-MW compounds (see Fig. S3 of the ESI†) could not be totally degraded. As such, the remaining feedwater in the MBR would impact the MW distribution of BMM. However, the large-MW compounds (i.e., >100 kDa) accounted for a great portion in EGP and DGP (i.e., the data for day 4, 8 and 13). For example, the large-MW compounds on day 8 and day 13 had a percentage up to 87% for both proteins and polysaccharides, indicating that the BMM were dominated by large biopolymers at that time. It indicates that the influence of remaining feed substrates mentioned above could be neglected. Additionally, it can be noted that the large-MW proteins and polysaccharides were of different compositions in the EGP and DGP. The large-MW proteins had a much larger proportion in the range >0.45 μm than those in the range 100 kDa–0.45 μm. The large-MW polysaccharides, in contrast, were mainly derived from the fraction in the size range 100 kDa–0.45 μm. This phenomenon reveals that the colloids in the sludge supernatant were possibly mainly composed of proteins, and the biopolymers (i.e., 100 kDa–0.45 μm) consisted predominantly of polysaccharides. However, the percentage of large-MW compounds decreased over operating time when the sludge reached the SGP. Correspondingly, the small-MW compounds (i.e., < 5 kDa) progressively became the majority of BMM fractions in the SGP, e.g., the percentages of small-MW compounds for proteins and polysaccharides were up to 52 and 53%, respectively, on the 57th day.
In this work, the average rejection degree of proteins was 24.7, 68.5 and 23.8% in the EGP, DGP and SGP, respectively. In comparison, the polysaccharides had an average rejection degree of 79.1, 96.6 and 77.2% , respectively (see Table 1). The higher rejection degree of both fractions in the DGP was mostly attributed to their large MW. The high rejection degree could in turn enable more large-MW BMM to be accumulated in the MBR, which finally led to a great increase of these compounds in the bioreactor.32 But, this needs to be further investigated due to their complex interrelation. Besides membrane rejection, another possibility for the variation of MW distribution in the course of MBR operation is the change of BAP production rate. It will be discussed in the following section 3.4.
3.3 Influence of microorganism growth on chemical constituents of BMM
To further understand the characteristics of BMM in different microorganism growth phases, three-dimensional EEM was applied to reveal the chemical components of BMM. Though the EEM technique is not able to detect polysaccharides,33 it can provide more detailed clues with respect to the other chemical compositions of BMM. EEM data of four typical BMM samples is shown in Fig. 3 (more EEM spectra are shown in Fig. S5 of the ESI†). Four peaks were detected by the EEM. The peaks at Ex/Em of 230–240/335–360 (Peak A) and 275–285/325–340 (Peak B) are associated with the aromatic protein-like substances and tryptophan protein-like substances, respectively.34 The other two peaks at 345–355/425–440 (Peak C) and 240–265/430–445 (Peak D) are attributed to humic acids and fulvic acids, respectively.35
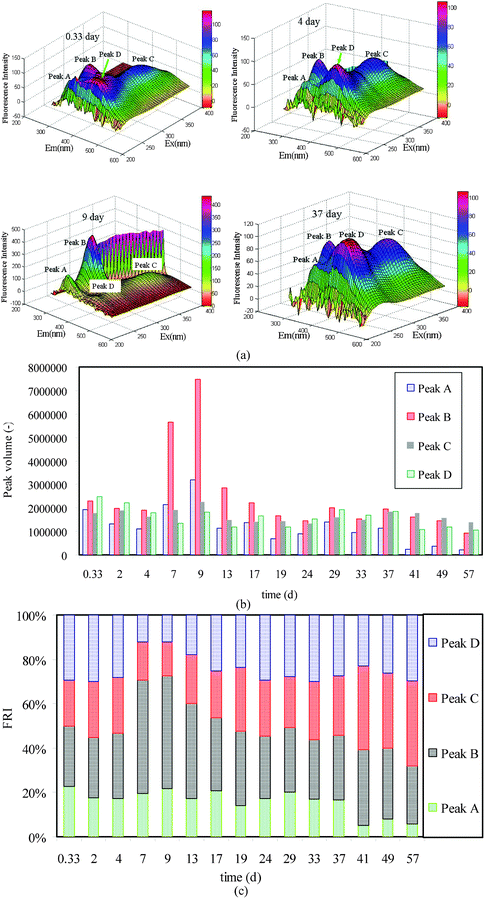 |
| Fig. 3
EEM
spectra of typical BMM samples (a), volumetric integration (b) and FRI (c) of EEM peaks in the course of MBR operation. | |
The fluorescence peak locations were found to be roughly the same in the whole MBR operation, but there were significant differences in the peak intensities, particularly for Peak A and Peak B. The variation of the peak volume was obtained by integrating the peak, which can be used to describe the content of one BMM component. As presented in Fig. 3b, the change of protein-like substances especially for the tryptophan proteins follows the similar trend of the protein concentrations quantified by the colorimetric method (Fig. 1a). Unlike protein peaks, the volume of Peak C and Peak D did not change significantly, indicating that they were ostensibly non-growth associated. It should be mentioned that the humics are usually of a much smaller MW than proteins and polysaccharides.36 Because of this, humic acids and fulvic acids are more readily discharged to membrane permeate,37–39 resulting in a more steady level of these compounds in the MBR sludge suspension. More details of this assumption will be present in the section 3.4. In contrast to our present results, Liet al.40 reported that the extracellular proteins and humic acids in an SBR were mainly associated with substrate utilization, while the fulvic acids were non-growth associated. The different results between our current work and that of Liet al.40 are possible due to the following reasons: (1) different feedwater types; (2) different feeding modes; (3) different bioreactor configurations and; (4) different sampling strategies. Moreover, the results of FRI shown in Fig. 3c suggest that the chemical compositions of BMM were varied, too. It can be clearly noted that the fluorescence active compounds in the BMM pool were mainly dominated by aromatic and tryptophan protein-like substances in the DGP . However, the proportion of humics (i.e., humic acids and fulvic acids) increased and gradually became the majority of BMM as the microorganism growth shifted to the SGP.
3.4 Production potential of BMM based on microorganism growth
To more clearly understand the production potential of BMM by the sludge under different growth phases, a series of BAP batch experiments were performed. Fig. 4 shows the production of proteins and polysaccharides in the batch experiments. For the batch experiment performed on day 1, the proteins were generated gradually on the whole process; however, the polysaccharides concentrations were decreased at 64th and 72th h, suggesting that the degradation rate of polysaccharides was greater than their production rate at that time. It was also found that both proteins and polysaccharides had high production rate on day 4 and day 9; therefore, it comes as no surprise that in the DGP there were high levels of BMM in the MBR. A clear trend can also be observed that the production rate of polysaccharides and proteins in the BAP batch study decreased rapidly as soon as the DGP was reached. Generally, the evolution trend of BAP in Fig. 4 is similar to that of BMM in Fig. 1a. Thus, the BAP production was one of the main reasons leading to BMM accumulation in the MBR sludge. Additionally, according to the results of bound EPS (Table 1), in the EGP and DGP there were sufficient EPS compounds which can be hydrolyzed to BAP due to microorganism metabolism. Niet al.41 also reported that a pool of organic compounds called growth-associated BAP (GBAP) could be produced in the microbial growth phase. Our current work, together with the data of Niet al.,41 indicates that besides biomass concentration, the growth stage of microorganisms is another important factor determining the production of BAP. In addition, some BMM compounds can be utilized by the starved microorganisms.42,43 Possibly, due to the simultaneous but varied production and elimination rates of proteins and polysaccharides, the BAP concentrations of each batch experiment fluctuated. For instance, the protein concentrations in the batch experiment of day 9 increased on 16th and 24th hour (i.e., production rate was larger than elimination rate), but it declined greatly in the following hours (i.e., elimination rate was larger than production rate). Particular interestingly, in comparison with the production rate of polysaccharides, that of proteins declined much earlier on the batch test of day 9. This was also one reason that the polysaccharides had a much higher level than proteins in the operating time from day 11 to day 20.
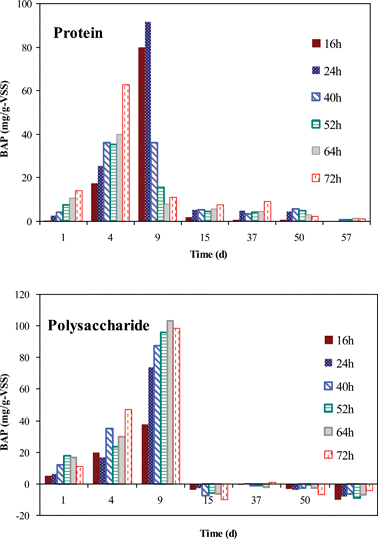 |
| Fig. 4 BAP production of the sludge taken from different operating time of the MBR. | |
As shown in Fig. 4, the polysaccharide production rate was always lower than its degradation rate in the SGP (i.e., the negative value from 15th to 57th day), implying that the polysaccharides in the MBR sludge was more labile. The feedwater used in this work had a much higher polysaccharide concentration of approx. 230 mg L−1 than the protein concentration of approx. 65 mg L−1, leading to more polysaccharide remaining in the MBR sludge as a consequence of the higher polysaccharide loading rate. In addition to this, as a result of their large MW, polysaccharide compounds were more readily retained by the membranes than proteins.37,44 Therefore, the high polysaccharide loading rate and rejection degree would eventually cause a higher polysaccharide concentration in the MBR sludge, though their BAP production potential was low or negative. The results of the BAP study could explain the importance of microorganism growth on BMM accumulation in MBRs. In brief, the results show that the sludge under the EGP and DGP would have a high potential to produce BMM.
The size nature and chemical composition of three BAP samples were also analyzed to get more evidence (see Fig. S6 of ESI†). In this work, we found that the BAP compounds mainly consisted of large-MW compounds on day 4 and 9. However, the proportion of large-MW compounds in BAP decreased on day 37 (data not shown). The similar evolution trend of BMM and the corresponding BAP including their concentration and MW over the whole MBR operation further confirm that the accumulation of BMM in the MBR sludge was mainly due to BAP production. In addition, some specialized bacterial strains able to degrade BMM compounds can be enriched during the long-term operation of MBRs, which can degrade large-MW polysaccharides and proteins into smaller molecules.3,45 In addition to these reasons, our current results show that the BAP produced by the microorganisms under SGP were of small MW. In addition, the EEM data of BAP samples also indicate that both the aromatic proteins and the tryptophan proteins were more growth-related than humics (data not shown).
4. Discussion
In this study, we report on the formation of BMM compounds in an MBR by focusing on the role of microorganism growth. The results of this work provide some interesting findings and updated knowledge on BMM formation mechanisms. Both quantity and quality of BMM in the MBR were found to depend on the growth stage of their producers. In the more rapid growth phases (i.e., EGP and DGP), the microorganisms seemed to produce abundant BMM compounds with large-MW. In addition, based on the EEM measurements, chemical compositions of BMM was also observed to rely on microorganism growth, despite the fact that the EEM spectrometer cannot detect all BMM compounds present in the BMM pool. According to these phenomena, it is worth pointing out that the growth stage of microorganisms should be fully considered when we study the formation of UAP and BAP by means of batch experiments.
In fact, the accumulation behavior of BMM compounds in the startup period of MBRs has been previously reported or mentioned in a number of publications.19,31,32 The accumulation of BMM was often attributed to the role of membrane rejection which can reject the large-MW compounds. The reduction of BMM levels in the period of steady operation was considered to be possible due to the liable BMM utilization by microorganisms under starvation conditions. These reported findings or hypothesis are useful for the design and operation of MBR plants. However, the mechanisms underlying the formation and elimination of BMM are not yet clarified so far. Apart from the operating conditions and substrate types, the formation of BMM in an MBR depends on the following factors: (i) change of microbial community, (ii) membrane rejection and, (iii) microorganism growth stage. Due to the joint-influence of these factors, it is difficult to give a clear conclusion that which factor is more important and how these factors are working on the BMM formation. However, microorganism growth and decay are ubiquitous in biological wastewater treatments. Therefore, this work is aimed to perform an in-depth and comprehensive study on BMM formation with a focus on microorganism growth stage. In this study, the sludge was acclimatised in advance, so the influence of shift of microbial community structure on the formation and elimination of BMM can be ruled out. In addition, to exclude the role of membrane rejection on BMM, a series of batch experiments were carried out to know the production potential of BMM by the MBR sludge. As discussed before (see Section 2.2), the production potential of BMM, in this study, was indicated by BAP formation. Results of the BAP batch study demonstrate that microorganism proliferation is indeed an important reason affecting BMM accumulation in MBRs. Clearly, the intensive microorganism proliferation can cause the production of more BMM in the growth phase. In the growth phase, the microorganisms can uptake the substrate faster due to the need of proliferation. At the same time, a part of substrate was stored or transformed by the cells as the form of biopolymers such as bound EPS. The results in Table 1 can not only suggest that more EPS were stored by the sludge flocs as a result of their rapid growth rates, but also indicate that the EPS composition such as the polysaccharide-protein ratio was influenced by the microorganism growth. As expected, a high EPS content can lead to a high production rate of BAP, especially during the DGP due to the abruptly limited nutrients, and hence a high concentration of BMM in the bioreactor.
Moreover, it should be borne in mind that the cell death will produce a great deal of BMM because of the release of EPS and biomass decay. The high BMM concentration on day 11, for example, can be possibly attributed to sludge decay. The relative steady values of VSS concentration in the SGP suggested that the sludge decay was balanced by new cell proliferation, although the VSS concentrations fluctuated which was due to the slight decrease of membrane flux and hence the decrease of substrate supply. It should be noted that the MBR operated in our study was subject to a short SRT of 5 days. Therefore, the frequent sludge discharge can induce new cell synthesis, which eventually enables the sludge to have satisfying metabolism activities for organic degradation including BMM elimination. Generally, the BMM or membrane fouling might be controlled by keeping the sludge in SGP. The findings obtained in this work also indicate that the control of BMM should be varied according to microorganism growth, e.g., dosing more coagulants/flocculants/adsorbents into MBR sludge when it is subject to rapid growth. Additionally, the reagents inhibiting EPS secretion also could be provided to reducing membrane fouling.4 It should be pointed out that the large-MW BMM compounds (>100 kDa) were the main fouling-causing substances in MBRs.8,46 Therefore, mitigating the production and accumulation of these large-MW substances is of great importance for membrane fouling control.
5. Concluding remarks
In summary, the results of this work reinforced the current knowledge of BMM formation mechanism in MBRs. Both quantity and quality of BMM could be impacted by microorganism growth stages. A great amount of BMM compounds could be produced by the sludge or microorganisms under the DGP . In contrast, a steady and low level of BMM was found as the microorganism growth reached SGP. Based on BAP study, the sludge under DGP had high potential to produce BMM due to abruptly limited nutrients and higher microbial secondary metabolism activities. This is a new and updated explanation to reveal the formation mechanism of BMM in the startup of MBRs. The microorganism growth phase could impact the size and chemical compositions of the BMM compounds strongly. The BMM produced in the EGP and DGP contained more large-MW compounds (> 100 kDa). It should be noted that the influence of the change of microbial community structure in the startup of MBR plants used for wastewater treatment is another research topic of high interest. Additionally, more detailed characterization of the chemical/structural composition of the BMM produced by microorganisms under different growth stage is needed in the future.
Acknowledgements
This work was supported by Specialized Research Fund for the Doctoral Program of Higher Education of China (No. 20100171120014) and the financial support of the Fundamental Research Funds for the Central Universities (No. 2010380003161541). Litree Corporation was thanked for the supply of the PVDF membrane modules.
References
- C. S. Laspidou and B. E. Rittmann, Water Res., 2002, 36, 2711–2720 CrossRef CAS.
- X. M. Wang and T. D. Waite, Environ. Sci. Technol., 2009, 43, 9341–9347 CrossRef CAS.
- X. Huang, R. Liu and Y. Qian, Process Biochem., 2000, 36, 401–406 CrossRef CAS.
- A. R. Badireddy, S. Chellam, S. Yanina, P. Gassman and K.M. Rosso, Biotechnol. Bioeng., 2008, 99, 634–643 CrossRef CAS.
- S. Liang, C. Liu and L. Song, Water Res., 2007, 41, 95–101 CrossRef CAS.
- S. Liang, Y. Zhao, C. Liu and L. Song, J. Membr. Sci., 2008, 310, 503–511 CrossRef CAS.
- X. Zheng, M. Ernst and M. Jekel, Water Res., 2009, 43, 238–244 CrossRef CAS.
- S. Rosenberger, C. Laabs, B. Lesjean, R. Gnirss, G. Amy, M. Jekel and J. C. Schrotter, Water Res., 2006, 40, 710–720 CrossRef CAS.
- S. Judd, Trends Biotechnol., 2008, 26, 109–116 CrossRef CAS.
- S. I. Patsios and A. J. Karabelas, J. Membr. Sci., 2011, 372, 102–115 CrossRef CAS.
- H. J. Lin, K. Xie, B. Mahendran, D. M. Bagley, K. T. Leung, S. N. Liss and B. Q. Liao, Water Res., 2009, 43, 3827–3837 CrossRef CAS.
- F. Meng, S. R. Chae, A. Drews, M. Kraume, H. S. Shin and F. Yang, Water Res., 2009, 43, 1489–1512 CrossRef CAS.
- Y.-L. Jin, W.-N. Lee, C.-H. Lee, I.-S. Chang, X. Huang and T. Swaminathan, Water Res., 2006, 40, 2829–2836 CrossRef CAS.
- T. Miyoshi, T. Tsuyuhara, R. Ogyu, K. Kimura and Y. Watanabe, Water Res., 2009, 43, 5109–5118 CrossRef CAS.
- H. Y. Ng, T. W. Tan and S. L. Ong, Environ. Sci. Technol., 2006, 40, 2706–2713 CrossRef CAS.
- S. Malamis and A. Andreadakis, Bioresour. Technol., 2009, 100, 3350–3357 CrossRef CAS.
- Z. Ahmed, J. Cho, B. R. Lim, K. G. Song and K. H. Ahn, J. Membr. Sci., 2007, 287, 211–218 CrossRef CAS.
- D. Al-Halbouni, J. Hollender, J. Traber, S. Lyko, T. Wintgens, T. Melin, D. Tacke, A. Janot and W. Dott, Water Res., 2008, 42, 1475–1488 CrossRef CAS.
- A. Masse, M. Sperandio and C. Cabassud, Water Res., 2006, 40, 2405–2415 CrossRef CAS.
- A. Omoike and J. Chorover, Biomacromolecules, 2004, 5, 1219–1230 CrossRef CAS.
- K. E. Eboigbodin and C. A. Biggs, Biomacromolecules, 2008, 9, 686–695 CrossRef CAS.
- A. R. Badireddy and S. Chellam, J. Appl. Microbiol., 2011, 110, 1426–1437 CrossRef CAS.
- A. R. Badireddy, S. Chellam, P. L. Gassman, M. H. Engelhard, A. S. Lea and K. M. Rosso, Water Res., 2010, 44, 4505–4516 CrossRef CAS.
- B.-J. Ni, R. J. Zeng, F. Fang, W.-M. Xie, G.-P. Sheng and H.-Q. Yu, Water Res., 2010, 44, 2292–2302 CrossRef CAS.
- B. Fround, R. Palmgren, K. Keiding and P. H. Nielsen, Water Res., 1996, 30, 1749–1758 CrossRef.
- M. Dubois, K. Gilles, J. Hamilton, P. Rebers and F. Smith, Anal. Chem., 1956, 28, 350–356 CrossRef CAS.
- O. H. Lowry, N. J. Rosebrough, A. L. Farr and R. J. Randall, Journal of Biological Chemistry, 1951, 193, 265–275 CAS.
-
Chinese-NEPA, Water and Wastewater Monitoring Methods., Beijing: Environmental Science Publishing House. 1997 Search PubMed.
- G. C. Huang, F. G. Meng, X. Zheng, Y. Wang, Z. G. Wang, H. J. Liu and M. Jekel, Appl. Microbiol. Biotechnol., 2011, 90, 1795–1803 CrossRef CAS.
- W. Chen, P. Westerhoff, J. A. Leenheer and K. Booksh, Environ. Sci. Technol., 2003, 37, 5701–5710 CrossRef CAS.
- M. C. Gao, M. Yang, H. Y. Li, Q. X. Yang and Y. Zhang, J. Biotechnol., 2004, 108, 265–269 CrossRef CAS.
- H. S. Shin and S. T. Kang, Water Res., 2003, 37, 121–127 CrossRef CAS.
- N. Her, G. Amy, D. McKnight, J. Sohn and Y. Yoon, Water Res., 2003, 37, 4295–4303 CrossRef CAS.
- G.-P. Sheng and H.-Q. Yu, Water Res., 2006, 40, 1233–1239 CrossRef CAS.
- Z. Wang, Z. Wu and S. Tang, Water Res., 2009, 43, 2504–2512 CrossRef CAS.
- F. Meng, Z. Zhou, B.-J. Ni, X. Zheng, G. Huang, X. Jia, S. Li, Y. Xiong and M. Kraume, Water Res., 2011, 45, 4661–4671 CrossRef CAS.
- F. Meng, A. Drews, R. Mehrez, V. Iversen, M. Ernst, F. Yang, M. Jekel and M. Kraume, Environ. Sci. Technol., 2009, 43, 8821–6 CrossRef CAS.
- S. Liang, L. F. Song and C. Liu, Water Res., 2007, 41, 95–101 CrossRef CAS.
- Z. W. Wang, Z. C. Wu and S. J. Tang, Water Res., 2009, 43, 1533–1540 CrossRef CAS.
- W.-H. Li, G.-P. Sheng, X.-W. Liu and H.-Q. Yu, Water Res., 2008, 42, 3173–3181 CrossRef CAS.
- B. J. Ni, R. J. Zeng, F. Fang, W. M. Xie, G. P. Sheng and H. Q. Yu, Water Res., 2010, 44, 2292–2302 CrossRef CAS.
- Z. W. Wang, Y. Liu and J. H. Tay, Appl. Microbiol. Biotechnol., 2007, 74, 462–466 CrossRef CAS.
- X. Zhang and P. L. Bishop, Chemosphere, 2003, 50, 63–69 CrossRef CAS.
- A. Drews, J. Mante, V. Iversen, M. Vocks, B. Lesjean and M. Kraume, Water Res., 2007, 41, 3850–3858 CrossRef CAS.
- D. Okamura, Y. Mori, T. Hashimoto and K. Hori, Environ. Sci. Technol., 2010, 44, 8644–8648 CrossRef CAS.
- W.-T. Zhao, Y.-X. Shen, K. Xiao and X. Huang, Bioresour. Technol., 2010, 101, 3876–3883 CrossRef CAS.
Footnote |
† Electronic supplementary information (ESI) available: The MBR set-up, feedwater composition, molecular weight distribution of feedwater and BAP samples, EEM data of BAP samples. See DOI: 10.1039/c1ra00420d |
|
This journal is © The Royal Society of Chemistry 2012 |
Click here to see how this site uses Cookies. View our privacy policy here.