DOI:
10.1039/C2RA00006G
(Paper)
RSC Adv., 2012,
2, 5837-5843
Fabrication of antibacterial silver nanoparticle—sodium alginate–chitosan composite films†
Received
3rd January 2012
, Accepted 12th April 2012
First published on 13th April 2012
Introduction
In recent years, silver nanoparticles (Ag NPs) have gained widespread attention in biotechnological and biomedical fields as catalysts,1 biosensors,2 antibacterial and anticancer agents.3–12 In principle, the synthesis of Ag NPs involves reduction of a silver salt with reducing agent in the presence of a stabilizer that prevents aggregation of NPs. Generally chemical reductants like sodium borohydride,3,8 hydrazine,13 dimethylformamide14 and stabilizers such as polyaniline (PANI)15 and polyvinylpyrrolidone (PVP)16 are used. However, they are generally associated with biological and environmental risks owing to their toxicity and non biocompatibility. Hence, the development of eco-friendly ‘green’ processes using naturally occurring carbohydrate based biopolymers as templates, for synthesis as well as stabilization has gained impetus because of their excellent biocompatibility.17–19 Our laboratory, in this regard, has previously developed a ‘green’ method of synthesizing Ag NPs using chitosan as reductant as well as stabilizer and demonstrated the successful application of thus prepared chitosan-Ag NPs nanocomposite as a potential antibacterial agent.4
Alginate is a naturally occurring poly-anionic polysaccharide derived from brown marine algae and composed of 1, 4-linked β-D-mannuronic and α-L-guluronic residues in varying proportions. It is a cheap, biocompatible and an environmentally benign biopolymer having numerous applications in the biotechnology industry as non-toxic food additive, thickening agent, gelling agent, emulsifier and colloidal stabilizer.20 However, there are very few reports available on the use of alginate for synthesizing metal NPs. Liu et al. reported the synthesis of Ag NPs using sodium alginate as a stabilizer under gamma radiation.21 Here isopropanol was added as a scavenger of hydroxyl radical. On the other hand, Pal et al. synthesized gold NPs using sodium alginate by UV photoactivation.22 However, both the methods mentioned above involve usage of high-energy irradiation which may not be favorable.
Alginate has a potential to form edible films which exhibit poor water resistance due to hydrophilicity owing to presence of carboxylic and hydroxyl groups. To overcome this, alginate has been crosslinked with polyvalent metal cations like Ca2+ or polyamines like chitosan, with which it can ionically interact to produce strong gels or insoluble polymers. Chitosan is a linear copolymer of β-(1–4)-2-acetamido-2-deoxy-β-D-glucose and β-(1–4)-2-amine-2-deoxyb-β-D-glucose, mainly obtained by deacetylation of chitin which is a natural polysaccharide present in crustacean shells. Chitosan can form films with excellent mechanical properties. The polyelectrolyte complex (PEC), obtained from the ionic interactions between the protonated amino groups of chitosan and carboxylate groups of alginate, has been shown to have reduced tendency of swelling and enhanced mechanical properties as compared to their constituent polymers.23 The alginate-chitosan PECs and calcium crosslinked hydrogels have potential applications in immobilization of living cells,24 controlled release delivery systems,25–29 wound dressing,30,31 membranes,32etc.
Herein, we report the development of a novel, facile and ‘green’ route for synthesizing Ag NPs using alginate as both reducing and stabilizing agent in aqueous medium by heating alone. No external agent was added. The synthesized alginate capped Ag NPs were characterized and found to be antibacterial. Due to the poor binding affinity with various surfaces, Ag NPs are usually incorporated in some matrix or solid supports for practical applications. Several studies, in this context, have demonstrated successful incorporation of Ag NPs in food packaging films33,34 and coatings on medical devices35,36 as antimicrobial agent. Thus, our next objective was to fabricate films of alginate capped Ag NPs for practical purposes. But since alginate film dissolves in aqueous solutions and exhibits poor mechanical stability,23 Alg–Ag NPs were blended with different ratios of chitosan to improve film properties. The films were characterized and mechanical properties studied. The biocidal activity of blended film was investigated against two Gram positive and three Gram negative bacterial strains and was found to be highly effective against each of them.To the best of our knowledge, this is the first time that an antibacterial film comprised of three components viz. Ag NPs, alginate and chitosan has been developed.
Materials and methods
Bacterial strains and growth conditions
The five bacterial strains used in the present study included two Gram positive strains of Bacillus cereus MTCC 1305, and Enterococcus faecalis MTCC 439 and three Gram negative strains of Escherichia coli, Enterobacter aerogenes MTCC 2822, and Pseudomonas aeruginosa MTCC 2488. Enterococcus faecalis MTCC 439 was grown in BHI broth medium at 37 °C at 220 rpm for 12 h, whereas B.cereus, Ps. aeruginosa MTCC 2488 and Eb. aerogenes MTCC 2822 were grown in NB medium at 37 °C at 220 rpm for 12 h. The recombinant GFP-expressing E. coli cells (DH5α) were grown in LB media.
Preparation of alginate capped Ag NPs (AlgnAg NPs) composite
Alg–Ag NPs composite was synthesized by adding 2 ml of freshly prepared 1.0 × 10−2 M AgNO3 solution to 50 ml of 0.2% (w/v) alginate solution, with constant stirring at 90 °C. The appearance of brown colour after 1 h indicated formation of Ag NPs.
Characterization of the composite
The formation of Ag NPs was monitored by making UV-Vis spectroscopic measurements using a spectrophotometer (Lambda 45; Perkin-Elmer, Fremont, CA). For transmission electron microscopy (TEM), 5 μl of the sample was drop-cast onto a carbon-coated copper TEM grid, which was subsequently air-dried. The drop-coated grid was analyzed by a high resolution transmission electron microscope (JEM 2100; Jeol, Peabody, MA, USA) operating at an accelerating voltage of 200 keV. The selected area electron diffraction (SAED) pattern of Ag NP was also recorded using the same instrument. The histograms for particle size distribution were constructed analyzing several frames of similar images. The zeta potential of Ag NPs was measured using Delsa ™ Nano C particle analyzer.
Preparation of alginate-Ag NPs-chitosan (Alg–Ag NPs-Chi) blended films
Films were prepared by varying the ratio of alginate and chitosan to 1
:
1, 2
:
1, and 4
:
1. In detail, 1
:
1 film was prepared by adding 6 ml of chitosan solution (0.1% w/v in a 1
:
1 v/v solvent mixture of 2% aqueous acetic acid and acetone) to 3 ml of Alg–Ag NPs solution under vigorous stirring at room temperature for 20 min. This was repeated five times, the resultant suspension (45 ml after pooling) was cast into a polyethylene petri dish and dried for 24 h at 37 °C in an incubator (Reico Equipment & Instrument Pvt. Ltd.). By a similar casting method, control films of chitosan and sodium alginate were also prepared. All films were stored in a desiccator at room temperature until use.
Characterization of films
The surface morphology of the films was examined by field emission scanning electron microscopy (FESEM) and light microscopy. For FESEM, the films were sputter-coated with gold film using a sputter coater (SC7620“Mini”, Polaron Sputter Coater, Quorum Technologies, Newhaven, England) and imaged under FESEM (Carl Zeiss, SIGMA VP). Optical microscopy images were acquired with a Carl Zeiss Axioskop 2 Mat Microscope fitted with a digital camera.The Fourier transform infrared (FTIR) spectra of the films were recorded using a Perkin-Elmer Spectrum one spectrometer. X-ray diffraction (XRD) patterns of the solid product of Alg–Ag NPs obtained after drying the colloidal dispersion and films were recorded with a Bruker D8 ADVANCE (Bruker AXS Inc.) XRD machine using Cu-Kα (λ = 1.54 Å) source.
Water uptake studies
Triplicate samples of each film were weighed by using a weighing balance (GR-202, A&D Company Ltd.) and equilibrated in 15 ml of PBS (pH 7.4) at room temperature. After 24 h, the films were retrieved, blotted of excess water and weighed immediately. The procedure was repeated until the membrane attained constant weight. The % uptake was determined by the following equation:
% uptake = [(wet weight − dry weight)/dry weight] × 100 |
Mechanical properties
The mechanical properties of films were measured using a Deben MICROTEST (Gatan) machine running at a speed of 5 mm min−1. Tensile strength was calculated by dividing the maximum force at break by the cross-sectional area of film (N m−2 = Pascal). Percent elongation at break was calculated on the basis of length extended as compared to the original length of the film. The reported film properties were average of measurements on three different films. The film thickness was measured by FESEM (Carl Zeiss, SIGMA VP) and the average of eight measurements is reported.
Antibacterial activity of films
The agar diffusion method was used to determine the antimicrobial effects of films on bacterial strains.The suspensions of bacterial cultures in the range of 105–106 cfu ml−1 were made and streaked over the surface of agar plates to obtain uniform growth. After the plates dried for 5 min, films of diameters 1, 1.5, and 2 cm were placed on the top of the swabbed agar. The zones of inhibition were measured after incubating the plates at 37 °C overnight. The reported values are an average of three independent experiments carried out with each strain.
Results and discussion
Synthesis and characterization of Alg–Ag NPs
In the present study, Ag NPs have been synthesized in a ‘green’ method using natural biopolymer alginate by simply heating an aqueous mixture of sodium alginate and AgNO3. Here, alginate plays dual role of both stabilizer and reducing agent. In the presence of alginate, the band at ∼301 nm exhibited by AgNO3 in aqueous solution disappears indicating possible chelation of Ag+ by the adjacent hydroxyl and carboxylic groups of alginate (Fig. 1a). The Ag+ chelate produces Ag0 on heating. The synthesis was carried out at ambient conditions without producing any interfering impurities like silver oxide. This method was found to produce Ag NPs reproducibly.
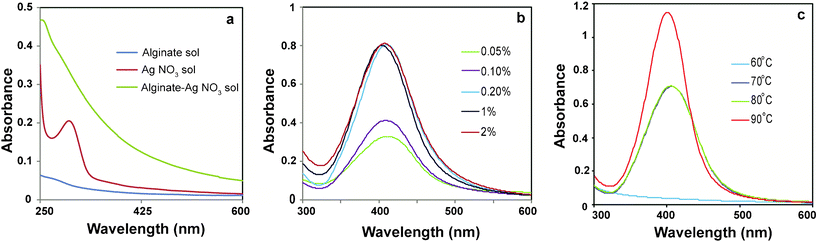 |
| Fig. 1 UV-Vis spectra of (a) alginate, AgNO3 and mixture of alginate and AgNO3 solutions, (b) Ag NPs synthesized using different concentrations of alginate at 80 °C and (c) Ag NPs at different temperatures keeping alginate concentration 0.2% (w/v). | |
In order to optimize the synthesis of Ag NPs, the concentration of alginate was varied (0.05, 0.1, 0.2, 1.0, 2.0 wt%) while keeping AgNO3 concentration and reaction temperature constant at 4 × 10−4 M and 80 °C, respectively. After 1 h of reaction, the color of the reaction mixture changed from yellow to light brown to dark brown with increasing alginate concentration, indicating the formation of Ag NPs. The UV-Vis absorption spectroscopy of the samples revealed the appearance of a peak at 407 nm, which is characteristic of surface plasmon resonance (SPR) of Ag NPs. As is evident from Fig. 1b, the intensity of the peak increased as the concentration of alginate increased till it became constant at an alginate concentration of 0.2% (w/v) or more, indicating complete reduction of silver ions at those concentrations of alginate.
Next, the influence of reaction temperature on the synthesis of Ag NPs was investigated at constant AgNO3 (4 × 10−4 M) and alginate concentration (0.2%, w/v). Fig. 1c compares the UV-Vis absorption spectra of Ag NPs obtained at different temperatures. The absence of an SPR band in UV-Vis spectrum indicated that no Ag NPs were formed at 60 °C. As the temperature was raised to 70 °C or above, a well defined plasmon band appeared at 407 nm. At 90 °C, the intensity of the SPR peak increased with a concomitant shift to lower wavelength of 402 nm indicating the formation of smaller Ag NPs. Also, the peak was sharp and symmetric indicating a narrow size distribution of Ag NPs synthesized at 90 °C. TEM analysis (Fig. 2a) showed that the Ag NPs were well-dispersed and spherical in shape. The particle size distribution, shown in Fig. 2d, revealed that the size of the particles was in the range of 5 to 21 nm with a mean diameter of 14 ± 3 nm. The observed lattice fringes in the HRTEM image (Fig. 2b) indicated the crystalline structure of Ag NPs. Also, the selected area electron diffraction pattern (SAED) of AgNPs (Fig. 2c) supported the formation of polycrystalline AgNPs. Fig. S1 shows the XRD pattern of Alg–Ag NPs.†
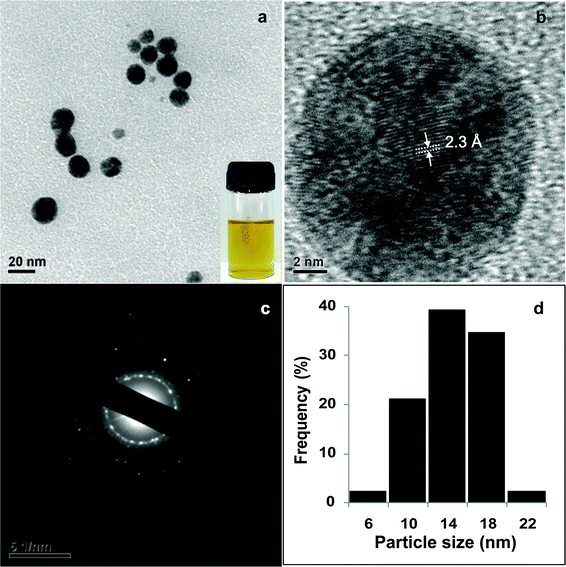 |
| Fig. 2 a) TEM image of Ag NPs synthesized using 0.2% alginate at 90 °C, inset shows the photo of Ag NPs (b) HRTEM image of a single Ag NP, (c) corresponding SAED pattern and (d) particle size distribution of Ag NPs. | |
Ag NPs were also prepared at higher AgNO3 concentrations (2 × 10−3 and 4 × 10−3 M) keeping alginate concentration and temperature as 0.2% and 90 °C respectively. UV-Vis spectra (Fig. S2†) and TEM studies (Fig. S3†) showed that particles of various shapes and sizes were formed. Thus, the optimum reaction conditions (alginate = 0.2%, AgNO3 = 4 × 10−4 M at 90 °C) were standardized to obtain uniform Ag NPs for experiments.
The Alg–Ag NPs were found to be antibacterial against E. coli (Gram negative) and B.cereus (Gram positive) strains as indicated by zones of inhibition around the wells loaded with Alg–Ag NPs in bacterial culture plates (Fig. S4†). Also, there was no zone of inhibition around the well containing only alginate solution, indicating no antibacterial activity of alginate.
Fabrication and characterization of Alg–Ag NPs-Chi blended films
In view of increasing popularity of Ag NPs based biomaterials to control infections,37,38 our next objective was to develop antibacterial films with Alg–Ag NPs synthesized in the present study for practical application. Alg–Ag NPs were blended with varying amounts of chitosan to have films with improved properties in comparision to Alg–Ag NPs film. However, the challenge was to have homogenous films with uniform concentration of Ag NPs throughout the film. This is because when chitosan is added to alginate solution, insoluble heterogenous coacervates are formed due to rapid interaction between protonated amino groups of chitosan and carboxylic groups of alginate. Hence, in order to ensure the homogeneity of films, acetone was used as a part of solvent for chitosan. Previously, Yan et al.32 had successfully used acetone to prepare coacervates for casting into homogenous films. The acetone ensures that chitosan remains in a less extended conformation so that its interaction with carboxylic groups of alginate is restricted.
The chitosan blended Alg–Ag NPs films (Alg–Ag NPs-Chi) in the present study were prepared as shown in Scheme 1, with various weight ratios of alginate and chitosan (1
:
1, 2
:
1, 4
:
1). Control films made up of only alginate and only chitosan were also prepared. It was found that with the increase in sodium alginate content, the films became fragile and difficult to handle. Hence, the film prepared with 1
:
1 ratio of alginate and chitosan was chosen for further studies as it was easier to handle.The control films were colourless and transparent in contrast to blended films which were yellow in colour due to presence of Ag NPs (Fig. S5†). The colour of the films did not change on storage for several weeks indicating stability of Ag NPs. The NPs can be seen in the FESEM image of 1
:
1 blended film (Fig. 3b) in the form of small particles, which revealed that the NPs were well dispersed throughout the film. On the other hand, the chitosan film did not contain any such small particulate structure (Fig. 3a). The observed cracks in the film could have occured during drying especially in the vacuum. The optical micrographs showed that chitosan and 1
:
1 blended films had smoother surfaces as compared to 2
:
1 and 4
:
1 films which had striated and irregular surface morphology (Fig. S6†).
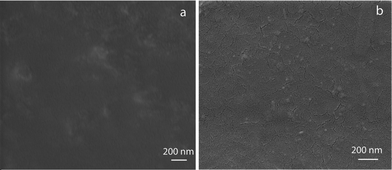 |
| Fig. 3 FESEM images of (a) chitosan film and (b) 1 : 1 blended film showing Ag NPs. | |
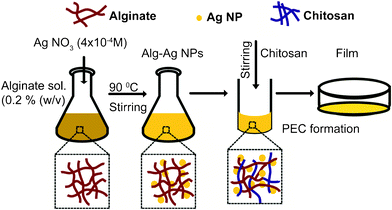 |
| Scheme 1 Schematic illustration of procedure used for the preparation of Alg–Ag NPs-chi blended films. | |
The FTIR studies of the films indicated the interaction between alginate and chitosan polymers (Fig. 4). The absorption bands at 1607 and 1415 cm−1 for alginate film were due to the respective asymmetric and symmetric stretching vibrations of carboxylate anions. The absorption band at 1607 cm−1 shifted to 1633 cm−1 and the band at 1415 cm−1 shifted to 1384 cm−1 after alginate reduced the silver ions to form Alg–Ag NPs. Also, the broad band at 3366 cm−1 corresponding to a stretching vibration of O–H groups in alginate shifted to 3428 cm−1 in Alg–Ag NPs. The peak shifting could be due to bond formation between silver and oxygen. Thus, from above, it can be inferred that perhaps both hydroxyl and carboxyl groups of alginate were involved in the synthesis and stabilization of Ag NPs. Previously, Kora et al. have also reported changes in shape and peak positions of hydroxyl and carboxylate groups of gum kondagogu, a polysaccharide, when used for Ag NPs synthesis.39 For chitosan film, the absorption bands at 1639, 1558, and 1324 cm−1 represented characteristic amide I, amide II and amide III bands respectively. The FTIR spectra of Alg–Ag NPs-Chi film showed a broad band from 1566 to 1647 cm−1 that arose from overlapping bands from amide of chitosan and carboxyl anions of alginate40 confirming the interaction between alginate and chitosan.
The XRD patterns of different films are shown in Fig. 5. The chitosan films showed two peaks at 2θ of 9.4 and 11.6°, corresponding to hydrated and anhydrous chitosan crystals, respectively. An additional broad peak that extended from 15.5 to 24° showed the amorphous region of the film.41 The diffractogram of alginate film consisted of two broad bands at 13.5 and 21.5° of low intensity. In Alg–Ag NPs-Chi film, the peaks corresponding to the crystalline region of chitosan almost disappeared and broad bands appeared at 14.2 and 21° indicating the amorphous morphology of the complex due to strong interactions between alginate and chitosan that destroyed the close packing of polymers.34 In addition, the Alg–Ag NPs-Chi film had a well defined characteristic diffraction peak at 38.3°, and weak peaks at 44.2 and 64.5°, respectively, which corresponded to (111), (200) and (220) planes of face centered cubic (fcc) crystal structure of metallic silver,42 indicating the presence of Ag NPs in the blended film.
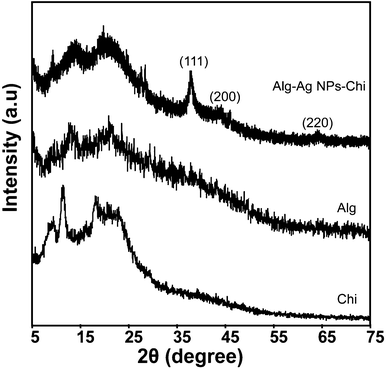 |
| Fig. 5 XRD of 1 : 1 blended (containing Ag NPs), alginate and chitosan films. | |
Water uptake studies
As the swelling behavior of an antibacterial film is an important aspect of its successful biomedical application, the water uptake capabilities of the blended films were investigated. Table 1 shows the water uptake behavior of the films in PBS (pH 7.4) at room temperature. The alginate film completely dissolved in PBS in contrast to blended films that remained stable. The % water uptake of chitosan film was highest compared to the blended films. This indicates that, in blended films, there is formation of a polyelectrolyte complex between the amino groups of chitosan and the carboxylic groups of alginate. The polymer chains became more entangled thereby restricting their mobility and thus limiting water uptake.43,44 Among the blended films, 1
:
1 film demonstrated slightly higher % water uptake than either 2
:
1 or 4
:
1 blended films.
Table 1 Water uptake of different films
Film |
% Water uptake (Mean ± SD, n = 3) |
Completely soluble in water, hence could not be measured.
|
Alg |
—a |
Chi |
45.4 ± 5.6 |
1 : 1 |
19.8 ± 1.2 |
2 : 1 |
15.3 ± 0.5 |
4 : 1 |
16.6 ± 0.3 |
Mechanical properties
The mechanical properties of the films were studied and are summarized in Table 2. Tensile strength measures the strength and elongation at break indicates stretchability of the film prior to breakage. The mechanical data of alginate film could not be obtained due to difficulty in peeling off the film from the casting plate. On the other hand, the blended films showed improved mechanical properties in comparision to alginate film with the 1
:
1 film demonstrating superior properties than the 2
:
1 film. It was found that the values of tensile strength and Young's modulus for the 1
:
1 blended film were comparatively less than that of the chitosan film. However, the elongation at break value for the 1
:
1 film was higher than chitosan film indicating higher elasticity of the 1
:
1 film.
Table 2 Mean mechanical properties of the films
Film |
Thickness (μm)a |
Elongation at break (%)b |
Tensile Strength (MPa)b |
Young's Modulus (MPa)b |
Mean ± SD (n = 8).
Mean ± SD (n = 3).
|
1 : 1 |
5.562 ± 0.43 |
23.80 ± 2.53 |
16.55 ± 3.62 |
520 ± 88.82 |
2 : 1 |
5.606 ± 0.38 |
11.14 ± 0.83 |
7.42 ± 1.82 |
398 ± 44.36 |
Chi |
5.547 ± 0.19 |
10.38 ± 0.39 |
24.77 ± 6.22 |
3496 ± 479.6 |
Antibacterial activity of Alg–Ag NPs-Chi blended films
In order to evaluate practical applicability of the blended film, the 1
:
1 film was cut into small circular pieces of diameters 1, 1.5, and 2 cm and tested against two Gram positive strains viz, Bacillus cereus MTCC 1305, and Enterococcus faecalis MTCC 439 and three Gram-negative strains viz, Escherichia coli, Enterobacter aerogenes MTCC 2822, and Pseudomonas aeruginosa MTCC 2488. Table 3 shows the antibacterial effect of blended films in terms of zone of inhibition. The results indicated that the film was antibacterial against both Gram negative and Gram positive bacteria with more inhibition against Gram positive bacteria as revealed by the size of the inhibitory zone. It should be mentioned here that the Gram positive bacteria have a less negative charge on the cell surface as compared to Gram negative bacteria.45 Alg–Ag NPs synthesized in the present study had a zeta potential value of −16.37 mV. The results presented in Table 3 implied that the net charge on Ag NPs might have remained negative after the addition of chitosan. However, Ag NPs are known to be reactive and thus will interact with the cell-surface of the bacteria eventually killing them. Now, since the composite is overall negatively charged and Gram negative bacteria have comparatively higher negative surface charge, the composite will interact less with them in comparison to the Gram positive bacteria. Hence the composite will be more effective against Gram positive bacteria, which has been the case herein. Previously Kora et al.39 also reported negatively charged Ag NPs to be more effective against Gram positive bacteria. Besides, it was found that the size of the inhibitory zone increased with an increase in film size due to the higher content of Ag NPs. The control film made only of chitosan did not show any inhibitory zone indicating non antibacterial activity of the film.The lack of antibacterial activity of chitosan films in contrast to antibacterial chitosan solutions has already been reported earlier.46 Also, as expected the film made of alginate and chitosan only without Ag NPs did not show any antibacterial activity.
Table 3 Antibacterial activity of 1
:
1 blended film for varying film diameter (dF)
Strains |
Zone of inhibition (mm)a |
|
d
F = 1 cm |
d
F = 1.5 cm |
d
F = 2 cm |
Mean ± SD (n = 9)
|
Gram negative
|
Escherichia coli
|
0.5 ± 0.03 |
1.5 ± 0.05 |
1.9 ± 0.05 |
Enterobacter aerogenes
|
0.7 ± 0.05 |
1.4 ± 0.02 |
1.5 ± 0.07 |
Pseudomonas aeruginosa
|
2.5 ± 0.03 |
2.8 ± 0.05 |
3.1 ± 0.08 |
Gram positive
|
Bacillus cereus
|
5.9 ± 0.03 |
5.9 ± 0.04 |
6.0 ± 0.03 |
Enterococcus faecalis
|
4.7 ± 0.04 |
4.9 ± 0.05 |
5.1 ± 0.04 |
Conclusion
The above study presents a new, facile and ‘green’ method for the synthesis of Ag NPs in aqueous medium using a natural and biodegradable polymer, sodium alginate which acted as a reducing as well as a stabilizing agent. The synthesized Alg–Ag NPs were uniform in size (14 ± 3 nm) and antibacterial. The as-synthesized Alg–Ag NPs were blended with chitosan and cast into stable films having improved properties in comparison to alginate film. The films were characterized and their mechanical properties studied. FTIR, XRD and water uptake studies of blended film indicated an interaction between alginate and chitosan polymers. The blended film was stable under storage and demonstrated effective bactericidal activity against both Gram positive and Gram negative bacteria with more efficacy against Gram positive bacteria. The present approach of synthesis of Ag NPs utilized green chemistry principles and thus, films prepared from as-synthesized NPs can potentially be used for food packaging, as wound dressings, graftings onto various implants and several other biotechnological and biomedical applications.
Acknowledgements
This research was supported by the Department of Biotechnology (Nos. No. BT/49/NE/TBP/2010, and BT/01/NE/PS/08), Department of Science and Technology (SR/S5/NM-108/2006). Assistance from CIF, IIT Guwahati is acknowledged.
References
- G. Merga, R. Wilson, G. Lynn, B. H. Milosavljevic and D. Meisel, J. Phys. Chem. C, 2007, 111, 12220–12226 CAS
.
- A. J. Haes and R. P. Van Duyne, J. Am. Chem. Soc., 2002, 124, 10596–10604 CrossRef CAS
.
- S. K. Gogoi, P. Gopinath, A. Paul, A. Ramesh, S. S. Ghosh and A. Chattopadhyay, Langmuir, 2006, 22, 9322–9328 CrossRef CAS
.
- P. Sanpui, A. Murugadoss, P. V. D. Prasad, S. S. Ghosh and A. Chattopadhyay, Int. J. Food Microbiol., 2008, 124, 142–146 CrossRef CAS
.
- M. Banerjee, S. Mallick, A. Paul, A. Chattopadhyay and S. S. Ghosh, Langmuir, 2010, 26, 5901–5908 CrossRef CAS
.
- I. Sondi and B. Salopek-Sondi, J. Colloid Interface Sci., 2004, 275, 177–182 CrossRef CAS
.
- J. R. Morones, J. L. Elechiguerra, A. Camacho, K. Holt, J. B. Kouri, J. T. Ramírez and M. J. Yacaman, Nanotechnology, 2005, 16, 2346–2353 CrossRef CAS
.
- J. S. Kim, E. Kuk, K. N. Yu, J.H. Kim, S. J. Park, H. J. Lee, S. H. Kim, Y. K. Park, Y. H. Park, C.-Y. Hwang, Y.-K. Kim, Y.-S. Lee, D. H. Jeong and M.-H. Cho, Nanomed.: Nanotechnol., Biol. Med., 2007, 3, 95–101 CrossRef CAS
.
- Y.-H. Hsin, C.-F. Chen, S. Huang, T.-S. Shih, P.-S. Lai and P. J. Chueh, Toxicol. Lett., 2008, 179, 130–139 CrossRef CAS
.
- R. Foldbjerg, D. A. Dang and H. Autrup, Arch. Toxicol., 2010, 85, 743–750 CrossRef
.
- P. AshaRani, M. P. Hande and S. Valiyaveettil, BMC Cell Biol., 2009, 10, 65 CrossRef CAS
.
- P. Sanpui, A. Chattopadhyay and S. S. Ghosh, ACS Appl. Mater. Interfaces, 2011, 3, 218–228 CAS
.
- U. Nickel, A. zu Castell, K. Pöppl and S. Schneider, Langmuir, 2000, 16, 9087–9091 CrossRef CAS
.
- I. Pastoriza-Santos and L. M. Liz-Marzán, Langmuir, 1999, 15, 948–951 CrossRef CAS
.
- Y. Kang, S. Choi, A. Gopalan, K. Lee, H. Kang and Y. Song, J. Non-Cryst. Solids, 2006, 352, 463–468 CrossRef CAS
.
- K. Chou and Y. Lai, Mater. Chem. Phys., 2004, 83, 82–88 CrossRef CAS
.
- P. Raveendran, J. Fu and S. L. Wallen, J. Am. Chem. Soc., 2003, 125, 13940–13941 CrossRef CAS
.
- J. He, T. Kunitake and A. Nakao, Chem. Mater., 2003, 15, 4401–4406 CrossRef CAS
.
- D. K. Božanić, S. Dimitrijević-Branković, N. Bibić, A. S. Luyt and V. Djoković, Carbohydr. Polym., 2011, 83, 883–890 CrossRef
.
- M. G. Carneiro-da-Cunha, M. A. Cerqueira, B. W. S. Souza, S. Carvalho, M. A. C. Quintas, J. A. Teixeira and A. A. Vicente, Carbohydr. Polym., 2010, 82, 153–159 CrossRef CAS
.
- Y. Liu, S. Chen, L. Zhong and G. Wu, Radiat. Phys. Chem., 2009, 78, 251–255 CrossRef CAS
.
- A. Pal, K. Esumi and T. Pal, J. Colloid Interface Sci., 2005, 288, 396–401 CrossRef CAS
.
- B. Smitha, S. Sridhar and A. A. Khan, Eur. Polym. J., 2005, 41, 1859–1866 CrossRef CAS
.
- F. Lim and A. Sun, Science, 1980, 210, 908–910 CAS
.
- B. Sarmento, D. Ferreira, F. Veiga and A. Ribeiro, Carbohydr. Polym., 2006, 66, 1–7 CrossRef CAS
.
- K. L. Douglas and M. Tabrizian, J. Biomater. Sci., Polym. Ed., 2005, 16, 43–56 CrossRef CAS
.
- G. Coppi, V. Iannuccelli, E. Leo, M. T. Bernabei and R. Cameroni, Drug Dev. Ind. Pharm., 2001, 27, 393–400 CrossRef CAS
.
- X. Yan, E. Khor and L. Y. Lim, Chem. Pharm. Bull., 2000, 48, 941–946 CrossRef CAS
.
- A. J. Ribeiro, C. Silva, D. Ferreira and F. Veiga, Eur. J. Pharm. Sci., 2005, 25, 31–40 CrossRef CAS
.
- X. Meng, F. Tian, J. Yang, C.-N. He, N. Xing and F. Li, J. Mater. Sci.: Mater. Med., 2010, 21, 1751–1759 CrossRef CAS
.
- L. Wang, E. Khor, A. Wee and L. Y. Lim, J. Biomed. Mater. Res., 2002, 63, 610–618 CrossRef CAS
.
- T. W. Chen, S. J. Chang, G. C.-C. Niu, Y. T. Hsu and S. M. Kuo, J. Appl. Polym. Sci., 2006, 102, 4528–4534 CrossRef CAS
.
- R. Tankhiwale and S. K. Bajpai, Colloids Surf., B, 2009, 69, 164–168 CrossRef CAS
.
- R. Yoksan and S. Chirachanchai, Mater. Sci. Eng., C, 2010, 30, 891–897 CrossRef CAS
.
- D. Roe, B. Karandikar, N. Bonn-Savage, B. Gibbins and J.-B. Roullet, J. Antimicrob. Chemother., 2008, 61, 869–876 CrossRef CAS
.
- S. T. Dubas, S. Wacharanad and P. Potiyaraj, Colloids Surf., A, 2011, 380, 25–28 CrossRef CAS
.
- M. L. W. Knetsch and L. H. Koole, Polymers, 2011, 3, 340–366 CrossRef CAS
.
- M. Rai, A. Yadav and A. Gade, Biotechnol. Adv., 2009, 27, 76–83 CrossRef CAS
.
- A. J. Kora, R. B. Sashidhar and J. Arunachalam, Carbohydr. Polym., 2010, 82, 670–679 CrossRef CAS
.
- X. Li, H. Xie, J. Lin, W. Xie and X. Ma, Polym. Degrad. Stab., 2009, 94, 1–6 CrossRef CAS
.
- X.-L. Yan, E. Khor and L.-Y. Lim, J. Biomed. Mater. Res., 2001, 58, 358–365 CrossRef CAS
.
- P. Gong, H. Li, X. He, K. Wang, J. Hu, W. Tan, S. Zhang and X. Yang, Nanotechnology, 2007, 18, 285604 CrossRef
.
- Y.-H. Lin, H.-F. Liang, C.-K. Chung, M.-C. Chen and H.-W. Sung, Biomaterials, 2005, 26, 2105–2113 CrossRef CAS
.
- G. Pasparakis and N. Bouropoulos, Int. J. Pharm., 2006, 323, 34–42 CrossRef CAS
.
- Y.-C. Chung, Y.-P. Su, C.-C. Chen, G. Jia, H.-L. Wang, J. C. G. Wu and J.-G. Lin, Acta Pharmacol. Sin, 2004, 25, 932–936 CAS
.
- L. J. R. Foster and J. Butt, Biotechnol.
Lett., 2011, 33, 417–421 CrossRef CAS
.
|
This journal is © The Royal Society of Chemistry 2012 |
Click here to see how this site uses Cookies. View our privacy policy here.