DOI:
10.1039/C2RA20956J
(Paper)
RSC Adv., 2012,
2, 8793-8796
Green dielectric materials composed of natural graphite minerals and biodegradable polymer†‡
Received
16th May 2012
, Accepted 27th July 2012
First published on 30th July 2012
Abstract
Polymeric nanocomposites are a promising dielectric due to their low cost, easy processability and flexibility but most polymers are difficult to treat and recycle leading to “white pollution”. Here, we report an eco-friendly “green” dielectric material made from natural graphite minerals and biodegradable poly(butylene succinate) synthesized by solution casting and hot pressing. Dielectric constant of resulting composite films agreed quite well with percolation theory and a low percolation threshold fc = 5.98% was obtained due to a high aspect ratio of natural graphite minerals. Composite films possessed a high dielectric constant of 113, around 28 times higher than that of pristine PBS (103) when volume fraction reached 5.5%. Furthermore, dynamic mechanical properties of composite films can be improved at the same time. Dielectric constant of composite films can be greatly improved by incorporating natural graphite minerals while mechanical flexibility can be maintained to be as good as that of pure polymer matrix.
Introduction
Dielectric materials with high dielectric constant (K) have been extensively investigated due to their wide applications in fields of actuators,1 electric power systems2 and electromagnetic interference shielding.3 Polymeric dielectric materials have outstanding tunability, easy processability and flexibility compared to metal-oxide and ceramic dielectrics.4 However, dielectric constants of general polymers are so low (K < 10) for most applications that several methods have been proposed to enhance dielectric constants of polymers.5–7 Integration of conductive fillers with insulating polymers as fundamental compositions to fabricate polymeric nanocomposites is a promising strategy to improve dielectric constants of polymers. Dielectric constant of polymers can be dramatically increased under a very low concentration of conductive fillers while the flexibility of polymers can be maintained, especially for conductive fillers with large aspect ratios.8,9 As the volume fraction of conductive filler reaches the percolation threshold (fc), the dielectric constant increases dramatically according to the power law:10–13where Km is the dielectric constant of polymer matrix and s is an exponent. This phenomenon can be explained as conductive fillers come into contact with each other resulting in insulator–conductor transition as the volume fraction of the minor phase approaches a critical value percolation threshold.
Commonly selected polymer matrices are poly(vinylidene fluoride) (PVDF), epoxy, polyimide (PI) and polystyrene (PS).14–17 Although these polymers have excellent mechanical, thermal and electrical properties, they are not degradable and nor easy to treat and recycle which leads to “white pollution”.18,19 Taken environmental protection into consideration, fabrication of high K polymeric dielectric materials with biodegradable polymers such as poly(3-hydroxybutyrate-co-3-hydroxyvalerate) (PHBV), poly(lactic acid) (PLA), poly(ε-caprolactone) (PCL), and poly(butylene succinate) (PBS) is of great scientific and commercial importance. Recently, investigations concerned about dielectric properties of biodegradable polymers have been focused on the effects of components of copolymers, molecular structure and crystallization behavior on their dielectric properties. Saad synthesized copolymer poly(ester-urethane)s composed of poly[(R)-3-hydroxybutyrate]-diol (PHB-diol) and poly(butyleneadipate)-diol (PBA-diol). Dielectric loss values decreased with increasing content of hard segment PHB.20 Hirai found that hydroxyl and carbonyl polar groups were preferred to improve the dielectric constant of starch ester (SE).21 Tai investigated the relationship between dielectric behaviors and crystal structure of pure PBS.22 Few researches about dielectric properties of nanocomposites based on biodegradable polymers have been reported. Duan selected graphite nanosheets (GNs) and multi-walled carbon nanotubes (MWCNTs) to enhance the dielectric constant of polylactide (PLA).23 Ke used different models to elucidate the dielectric properties of poly(3-hydroxybutyrate-co-3-hydroxyhexanoate) (PHBHHx)-BaTiO3 nanocomposites that can be potentially applied as biomedical materials.24 Among various biodegradable polymers, PBS possesses excellent thermal, mechanical and processing properties similar to polyethylene (PE) and is commercially available.22,25 In our previous report, a high dielectric constant, all-organic composite film was achieved by combining polyaniline (PANI) filler with PBS.26 Although PANI fibers are low cost and easily synthesize, synthetic PANI cannot be degradable and recycled.
In order to make polymeric nanocomposites fully eco-friendly, natural graphite minerals are selected as functional components. Besides environmental friendliness, natural graphite minerals have excellent mechanical strength, thermal and chemical stability, electrical conductivity and large aspect ratio.27,28 To the best of our knowledge, there have been no reports on “green” high K dielectric materials based on natural graphite minerals and biodegradable polymers. As the dispersion of filler in the polymer matrix plays a key role in the final performance, a two-step process is designed and the resulting material with 5.5% natural graphite minerals boast a high dielectric constant of 113 (103 Hz) that is 28 times higher than that of pure PBS.
Results and discussion
Morphology and structure of nano-natural graphite and composite films
Percolation phenomena are usually observed in particle-filled polymer nanocomposites. For percolative nanocomposites, percolation threshold is an important parameter that is influenced by processing conditions, size and shape of fillers.11 In order to reduce percolation threshold that was beneficial to maintain mechanical flexibility of polymeric nanocomposites, natural graphite minerals with large aspect ratios were prepared by ultrasonic processing. From Fig. S1 and Fig. S2 (ESI†), it can be observed that the thickness of treated natural graphite minerals was around 13 to 40 nm and the diameter about 5 to 20 μm (aspect ratio of 1.5 × 103 to 1.2 × 102). Fig. 1(a) showed fracture morphology of pure PBS. The dense structure of PBS was attributed to the hot pressing process. As shown in Fig. 1(b) and (c), natural graphite flakes exhibited good dispersion stability in the PBS matrix due to solution casting and hot pressing (see Fig. S3, ESI†). The nano-natural graphite well dispersed in PBS dissolved in DMAc solution for vigorous stirring made the nano-natural graphite homogeneously distribute in NNGM/PBS composite pellets. The hot pressing process during which composite films were prepared by mould pressing NNGM/PBS composite pellets several times further improved dispersion of NNGM in PBS. As volume fraction reaches 5.5%, natural graphite flakes were almost in contact with each other forming a conductive network. As shown in Fig. 1(d), the composite film with 5.5% retained good flexibility just as pure PBS matrix.
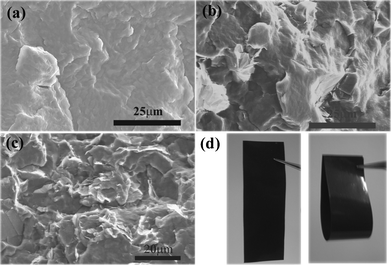 |
| Fig. 1 SEM micrographs of fracture surface of (a) pure PBS and NNGM/PBS composite films with (b) 2.5% and (c) 5.5%. (d) NNGM/PBS composite film with 5.5%. | |
Additionally, the interaction between natural graphite flakes and PBS was another reason for good dispersion of natural graphite flakes in PBS matrix. The interaction between natural graphite flakes and PBS was analyzed by Raman spectroscopy, DMA and XRD. Raman results of NNGM and NNGM/PBS composite films with 2.5% NNGM were shown in Fig. S4 (see ESI†). For pristine NNGM, the peak at 1339.6 cm−1 assigned to disorder-induced D band from the multiple photo scattering of defects or amorphous carbon. The peak located at 1579.1 cm−1 assigned to G band is assigned to the stretching of the conjugated double bonds corresponding to sp2 hybridization. For NNGM/PBS composite films with 2.5%, D band shift was attributed to compressive forces associated with polymer chains on the NNGM. Natural graphite flakes are electron-rich molecules and PBS has a variety of electron deficient ester groups. The donor–acceptor complexes were formed between natural graphite flakes and PBS matrix at high processing temperature and pressure (180 °C and 20 MPa) resulting in excellent dispersion of natural graphite flakes in PBS matrix.29 Dynamic mechanical analysis (DMA) also illustrated the interaction between natural graphite flakes and PBS. From Fig. S5(a) (see ESI†), the tanδ peak of pure PBS at around −23 °C corresponded to the glass-transition temperature. For NNGM/PBS composite films, tanδ peak moved towards a high temperature due to the hindering action between natural graphite minerals and polymer chain indicated interactions between natural graphite minerals and PBS. Similar results have been reported for polyamide/clay nanocomposites by Tjong.30 From Fig. S5(b),† G′ were 4.2 × 103 MPa and 6.4 × 103 MPa for pure PBS and NNGM/PBS composite films with 5.5%, respectively. Dynamic mechanical properties of composite films can be improved with the addition of NNGM.
Fig. 2 showed that pristine PBS had two diffraction peaks at 2θ = 19.3° and 21.7° corresponding to (020), (021) planes of PBS crystals, indicating PBS was semi-crystalline.31 For composite films, two new diffraction peaks at 2θ = 26.0° and 28.7° assigned to nano-natural graphite minerals appeared. Additionally, main diffraction peaks of PBS shifted to higher angles. This phenomenon can be explained by the interactions between natural graphite flakes and PBS under high processing temperature and pressure and incorporation of nano-natural graphite minerals influenced crystallization behavior of PBS which agreed with Raman and DMA analysis.
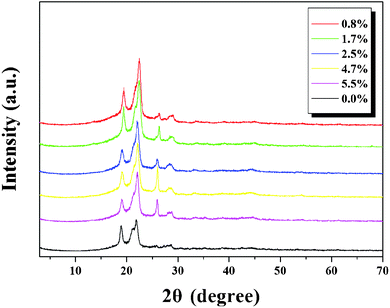 |
| Fig. 2 XRD patterns of pure PBS and NNGM/PBS nanocomposites. | |
Dielectric properties of composite films
Fig. 3 shows the dielectric constants of nano-natural graphite minerals (NNGM)/PBS composite films as a function of NNGM volume fraction and frequency at ambient temperature. When the volume fraction of NNGM was lower than 1.7%, the dielectric constant of composite films was almost the same as that of pure PBS and independent of frequency. When the volume fraction of NNGM was higher than 2.5%, tyhe dielectric constant of the composite films increased with increasing volume fraction of NNGM and was apparently dependent of frequency. A change in trend of dielectric constant was related to Maxwell–Wagner–Sillars (MWS) interfacial polarization for composite films. For composite films with a volume fraction of NNGM lower than 1.7%, the concentration of NNGM was so low that MWS interfacial polarization was so weak the dielectric constant was dominated by PBS. For composite films with a volume fraction of NNGM higher than 2.5%, the dielectric constant was dominated by MWS interfacial polarization. Dielectric constants decreased with increasing frequency because orientation polarization of dipoles formed at insulator/conductor interfaces cannot follow the change in the electrical polarity.31
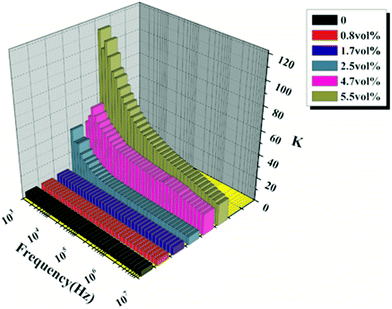 |
| Fig. 3 Dependence of frequency and volume fraction of natural graphite minerals on dielectric constant. | |
In order to investigate relationships between structures and properties of composite films, dielectric constants were plotted as a function of volume fraction of NNGM (Fig. 4). Dielectric constants of composite films slightly improved as volume fraction was lower than 2.5% and dramatically increased to 113, approximately 28 times higher than that of pure PBS as the volume fraction reached 5.5%. This phenomenon follows quite well the percolation theory. According to percolation theory, the best fit of a dielectric constant to eqn (1) was shown in Fig. 4 giving fc = 5.98% and critical exponent s = 1.1. The percolation threshold fc was lower than that of Ag and Ni filled polymeric composites because of the large aspect ratio of natural graphite minerals. Composite films with a volume fraction lower than fc had better dynamic mechanical properties than that of PBS matrix (see Fig. S5, ESI†). The s value was higher than universal value (sun = 0.8–1) but close to that of exfoliated graphite/PVDF.8 As percolation threshold fc was paid great attention for percolative nanocomposites, relationships between particles size and percolation threshold fc were investigated according to Garboczi's study:32
where A
f was aspect ratio of nano-natural graphite
minerals. Accordingly,
eqn (2) yielded A
f = 21, that was considerably smaller than value observed from Fig. S1 and Fig. S2 (see ESI
†). Natural graphite flakes were inclined to stack layer by layer when the volume fraction was near the percolation threshold
fc during solution casting and hot pressing due to van der Waals force. Thus, several originally separated natural graphite flakes were compacted together which increased thickness and decreased the aspect ratio of natural graphite flakes in
PBS matrix.
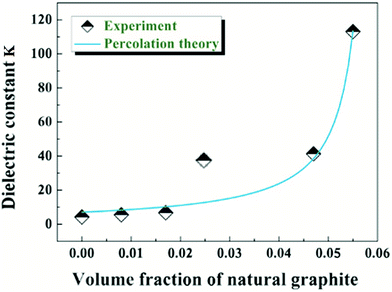 |
| Fig. 4 Best fits of dielectric constant (103 Hz at room temperature) to eqn (1). | |
Experimental
Materials
The biodegradable polymer matrix PBS (average Mw∼530
000) was supplied by the Technical Institute of Physics and Chemistry, Chinese Academy of Sciences. Natural graphite minerals (≥99% pure) with a density of 0.8 g cm−3 and conductivity of 11.8 s cm−1 were obtained from Jinghua Company, Shandong, China. The average size of natural graphite minerals are 425 mesh.
Preparation of composite films
Nano-natural graphite was prepared from natural graphite minerals using ultrasonic techniques.33 5 g natural graphite was dispersed in 1 L ethanol/water (7/3) solution and ultrasonicated for 6 h. NNGM/PBS composite films were prepared by two steps. Typical preparation process for composite film with NNGM volume fraction of 0.8% was as follows: 0.04 g nano-natural graphite and 4 g PBS were dispersed in 30 mL N,N-dimethylacetamide (DMAc) solution and vigorously stirred for 12 h in order to make nano-natural graphite well dispersed in PBS. After drying for 24 h, NNGM/PBS composite pellets were made by complete removal of DMAc. The composite film was prepared by mould pressing composite pellets at 180 °C and 20 MPa several times. Composite films with different volume fractions of NNGM (v = 0, 0.8, 1.7, 2.5, 4.7, and 5.5%) were obtained. Pure PBS film was synthesized by the same preparation procedure as that of composite films just without adding nano-natural graphite. The average thickness of these composite films was about 150 μm.
Characterizations
The morphology of materials was examined by scanning electron microscopy (SEM, JSE-6301F, JEOL). In dielectric measurements, top and bottom electrodes were made by coating silver paint on both sides of the composite films. The frequency dependence on dielectric constant was measured using a frequency response analyzer (Novocontrol Alpha-analyzer) over a broad frequency range (103 Hz to 10 MHz). X-Ray diffraction (XRD) patterns were acquired on a Rigaku D/max-rA X-ray diffractometer. The samples were scanned at 8° min−1 using Cu-Kα radiation (0.154 nm) emitted from a filament (40 kV and 100 mA). A LabRAM Aramis spectrometer was used for recording the Raman spectra using Ar-ion excitation sources (514.5 nm) below 20 mW of laser power. DMA experiments were conducted on TA Instrument DMA 2980 in the film tension mode at a fixed frequency of 1 Hz. Dimensions of samples were 20 × 8 mm2. Samples were tested in a temperature range from −100 °C to 100 °C under N2 at a heating rate of 3 °C min−1.
Conclusion
Eco-friendly composite films composed of natural graphite minerals and biodegradable polymer were prepared by a two-step method involving solution casting and hot pressing. SEM revealed good dispersion of natural graphite minerals in PBS matrix and composite films exhibited a high dielectric constant of 113 that was about 28 times higher than that of the pristine polymer matrix while the mechanical flexibility was maintained. The enhancement of dielectric constant was attributed to MWS interfacial polarization. Incorporation of natural graphite minerals with low loading improved the dielectric constant as well as the storage modulus of nano-natural graphite minerals (NNGM)/PBS composite films.
Acknowledgements
This study was jointly supported by the Key Project of Chinese Ministry of Education (No.107023), Fundamental Research Funds for the Central Universities (2011PY0180, 2011PY0181), National Laboratory of Minerals of China University of Geosciences (Beijing) (09B003), and the City University of Hong Kong Strategic Research Grant (SRG) No.7008009.
References
- M. Molberg, D. Crespy, P. Rupper, F. Nüeschl, J. A. E. Månson, C. Löwe1 and D. M. Oprisl, Adv. Funct. Mater., 2010, 20, 3280 CrossRef CAS.
- B. J. Chu, X. Zhou, K. L. Ren, B. Neese, M. R. Lin, Q. Wang, F. Bauer and Q. M. Zhang, Science, 2006, 313, 1887 CrossRef CAS.
- M. S. Cao, W. L. Song, Z. L. Hou, B. Wen and J. Yuan, Carbon, 2010, 48, 788 CrossRef CAS.
- P. Thomas, K. T. Varughese, K. Dwarakanath and K. B. R. Varma, Compos. Sci. Technol., 2010, 70, 539 CrossRef CAS.
- Q. M. Zhang, H. F. Li, M. Poh, F. Xia, Z. Y. Cheng, H. S. Xu and C. Huang, Nature, 2002, 419, 284 CrossRef CAS.
- Y. Bai, Z. Y. Cheng, V. Bharti, H. S. Xu and Q. M. Zhang, Appl. Phys. Lett., 2000, 76, 3804 CrossRef CAS.
- L. Qi, B. I. Lee, S. H. Chen, W. D. Samuels and G. J. Exarhos, Adv. Mater., 2005, 17, 1777 CrossRef CAS.
- F. He, S. Lau, H. L. Chan and J. T. Fan, Adv. Mater., 2009, 21, 710 CrossRef CAS.
- J. Zhang, M. Mine, D. Zhu and M. Matsuo, Carbon, 2009, 47, 1311 CrossRef CAS.
- J. K. Yuan, Z. M. Dang, S. H. Yao, J. W. Zha, T. Zhou, S. T. Li and J. B. Bai, J. Mater. Chem., 2010, 20, 2441 RSC.
- C. W. Nan, Y. Shen and J. Ma, Annu. Rev. Mater. Res., 2010, 40, 131 CrossRef CAS.
- Q. Li, Q. Z. Xue, L. H. Hao, X. L. Gao and Q. B. Zheng, Compos. Sci. Technol., 2008, 68, 2290 CrossRef CAS.
- B. K. Zhu, S. H. Xie, Z. K. Xu and Y. Y. Xu, Compos. Sci. Technol., 2006, 66, 548 CrossRef CAS.
- Z. M. Dang, Y. H. Lin and C. W. Nan, Adv. Mater., 2003, 15, 1625 CrossRef CAS.
- Y. Shen, Y. H. Lin and C. W. Nan, Adv. Funct. Mater., 2007, 17, 2405 CrossRef CAS.
- Z. M. Dang, Y. Q. Lin, H. P. Xu, C. Y. Shi, S. T. Li and J. B. Bai, Adv. Funct. Mater., 2008, 18, 1509 CrossRef CAS.
- M. Xiao, L. Y. Sun, J. J. Liu, Y. Li and K. C. Gong, Polymer, 2002, 43, 2245 CrossRef CAS.
- S. M. Ke, H. T. Huang, L. Ren and Y. J. Wang, J. Appl. Phys., 2009, 105, 096103 CrossRef.
- Z. B. Qiu, M. Komura, T. Ikehara and T. Nishi, Polymer, 2003, 44, 7781 CrossRef CAS.
- G. R. Saad, Macromol. Biosci., 2001, 9, 387 CrossRef.
- N. Hirai, Y. Maeno, H. Tamura, D. Kaneko, T. Tanaka, Y. Ohki, Y. Tajitsu, M. Kohtoh and S. Okabe, PROCEEDINGS OF THE 2004 IEEE INTERNATIONAL CONFERENCE ON SOLID DIELECTRICS, 2004, 1–87 Search PubMed.
- H. J. Tai, Polymer, 2007, 48, 4558 CrossRef CAS.
- J. K. Duan, S. X. Shao, Ya-Li, L. F. Wang, P. K. Jiang and B. P. Liu, Iran. Polym. J., 2012, 21, 109 CrossRef.
- S. M. Ke, Y. Yang, L. Ren, Y. J. Wang, Y. Y. Li and H. T. Huang, Compos. Sci. Technol., 2012, 2, 370 CrossRef.
- Y. F. Shih, L. S. Chen and R. J. Jeng, Polymer, 2008, 49, 4602 CrossRef CAS.
- L. Yu, S. M. Ke, Y. H. Zhang, B. Shen, A. Z. Zhang and H. T. Huang, J. Mater. Res., 2011, 19, 2493 CrossRef.
- K. Kalaitzidou, H. Fukushima and L. T. Drzal, Carbon, 2007, 45, 1446 CrossRef CAS.
- A. Celzardt, E. McRae and J. F. Mareche, J. Phys. Chem. Solids, 1996, 57, 715 CrossRef.
- J. K. Yuan, S. H. Yao, Z. M. Dang, A. Sylvestre, M. Genestoux and J. B. Bai, J. Phys. Chem. C, 2011, 115, 5515 CAS.
- S. C. Tjong and S. P. Bao, J. Polym. Sci., Part B: Polym. Phys., 2004, 42, 2878 CrossRef CAS.
- N. T. Tunga, T. V. Khai, H. Lee and D. Sohn, Synth. Met., 2011, 161, 177 CrossRef.
- E. J. Garboczi, K. A. Synder and J. F. Douglas, Phys. Rev. E: Stat. Phys., Plasmas, Fluids, Relat. Interdiscip. Top., 1995, 52, 819 CrossRef CAS.
- G. H. Chen, W. G. Weng and D. J. Wu, Carbon, 2004, 42, 753 CrossRef CAS.
Footnotes |
† Electronic Supplementary Information (ESI) available. See DOI: 10.1039/c2ra20956j/ |
‡ Li Yu and Yihe Zhang designed all experiments. Li Yu, Wangshu Tong, Jiwu Shang, Bo Shen, and Fengzhu Lv contributed to this work in experiments, analysing results and drafting the manuscript. Paul K. Chu helped with revisions. |
|
This journal is © The Royal Society of Chemistry 2012 |
Click here to see how this site uses Cookies. View our privacy policy here.