DOI:
10.1039/C2RA21319B
(Paper)
RSC Adv., 2012,
2, 11053-11060
A new hydrogel from an amino acid-based perylene bisimide and its semiconducting, photo-switching behaviour†
Received
29th June 2012
, Accepted 16th September 2012
First published on 9th October 2012
Abstract
A new, stable, semiconducting, photo-responsive, amino acid (L-tyrosine) based perylene bisimide derivative (PBI-Y) was found to form pH-sensitive hydrogels. This gelator molecule (PBI-Y) has undergone hydrogelation at 50 mM phosphate buffer at pH 5.00–9.00. Different minimum gelation concentration values (MGC) were recorded in different pHs at the same phosphate buffer strength (50 mM). The hydrogel was also characterized using different techniques, including X-ray diffraction analysis, rheology and FT-IR study. The fluorescence and cyclic voltammetric responses of the PBI-Y hydrogelator can be tuned by changing the pH. The PBI-Y hydrogelator has shown self-assembled nanofibrillar network structures, as it has been evident from the field emission scanning electron microscopic (FE-SEM), as well as transmission electron microscopic analyses (TEM). This PBI-Y hydrogel has also shown interesting semiconducting behaviour. This PBI-Y hydrogelator-based photoconductor was found to exhibit excellent photo-switching behaviour with a high photo-response value. This holds a future promise for the creation of PBI-conjugated functional soft-materials-based photodetectors and photovoltaics.
A Introduction
Stimuli-responsive smart materials can exhibit dramatic changes in their supramolecular organization in response to the external stimuli, including light, pH, temperature, electricity, chemicals, mechanical stress and others.1 These supramolecular smart functional materials are endowed with a fascinating property of reversibility between integrated and disintegrated phases and this is dictated by various non-covalent interactions, including hydrogen bonding, van der Waals, π–π stacking, solvophobic and electrostatic interactions in suitable solvents.2 In recent years, significant research interest has been dedicated towards functional organic materials consisting of π-conjugated molecular building blocks due to their potential applications in organic electronics and photonics.3 These π-conjugated organic materials have been extensively applied in light harvesting materials,4 light emitting diodes,5 field-effect transistors6 and photovoltaic cells.7 Among several π-conjugated organic building blocks, perylene bisimides are unique due to their interesting photo-physical properties and they have a large π-surface to form functional aggregated species.8 Being motivated by the fascinating properties of perylene bisimide-based molecules, we are curious to develop a new type of hydrogelator consisting of a protein amino acid-based perylene bisimide molecule with an aim to use this functional biomaterial as a photo-switching and semiconducting smart material. Hydrogels9 belong to a special type of soft materials in which self-assembling molecular building blocks form a fibrous network structure with a lot of void spaces that are occupied by a large volume of solvent molecules. Amino acid-10 and short peptide-based11 hydrogelators are fascinating candidates for developing low molecular weight functional gels. Molecules including amino acid- and dipeptide-based derivatives have revolutionized the field of novel biomaterials and they have been used in various potential applications, including cell growth, tissue engineering, light harvesting materials, drug delivery vehicles and other fields.12 There are a few reports on conducting gel-based π-systems.13 Ajayaghosh and co-workers have reported an oligo(thienylenevinylene) based-organogelator with high conductivity.13a,b Oligothiophene-based nanorods and nanotapes with high charge carrier mobility have also been reported.13c Zang and co-workers have made a pioneering contribution by making perylenebisimide-based photoconducting nanobelts, nanofibrils and nanoribons.13d–f However, a perylenebisimide-containing hydrogel-based photo-switching biomaterial is yet to be explored. Development of low-molecular mass perylene bisimide derivatives has recently become a fascinating area of research due to their relevance and potential applications in organic electronics and other fields.14 However, the aromatic perylene core in perylene bisimides has been restricted to use in aqueous systems owing to the strong hydrophobic nature of those compounds. There are relatively few examples of water-soluble perylene bisimide compounds.15 There are several reports on the design and development of perylene bisimide-based organogelators in the literature.5,8,12b,c,e,16 Würthner and co-workers have made a significant contribution to the synthesis and development of perylene bisimide-based organogelators.8,16a,b Shinkai and co-workers have demonstrated the synthesis and development of a visible-light-harvesting cholesterol-based perylene bisimide derivative that has undergone organogelation mainly in aromatic organic solvents.16c Moreover, Rybtchinski and co-workers have reported perylene bisimide-based multiple stimuli-responsive, robust and photo-responsive gels.16d A perylene bisimide derivative-based two component hydrogelator has been reported recently.17 To the best of our knowledge, there is no report on an amino acid-containing perylene based gelator. Our main goal is to make a water-soluble amphiphilic molecule that has a perylene bisimide containing an amino acid residue and also to study the self-assembly behaviour of this compound.
A new perylene bisimide derivative containing a L-tyrosine moiety (PBI-Y) has been found to form a pH-responsive supramolecular hydrogel between the pH range 5.00–9.00. Interestingly, the PBI-Y-based gel has shown semiconducting, as well as photo-switching behaviour. The PBI-Y gel-based conductor has been successfully fabricated to examine its photo-response to both visible light and white light. It is worth mentioning that the photocurrent increases sharply upon exposure to both kind of lights (i.e. ‘ON’ state) and decreases after the withdrawal of the source light (i.e. ‘OFF’ state). These “ON” and ‘OFF’ conditions under the action of light make it an excellent material for a photo-switch, further exhibiting a promising application of such a device for the development of photo-detectors and photovoltaic cells.
B Experimental section
General methods and materials
3,4:9,10-perylenetetracarboxyldianhydride was purchased from Sigma-Aldrich chemicals. Sodium dihydrogen phosphate and disodium hydrogen phosphate salts were purchased from Merck. L-tyrosine, imidazole and others were purchased from SRL local chemicals.
Synthesis of [N,N′-di(L-tyrosine)-perylene-3,4:9,10-tetracarboxylic acid bisimide]-(PBI-Y).
In a 100 mL round bottom flask, L-tyrosine 0.36 g (2 mmol), 3,4:9,10-perylenetetracarboxyldianhydride (PTCDA) 0.392 g (1 mmol) and 1.36 g imidazole (20 mmol) were mixed. The mixture was then purged with N2 for 10 min before being heated at 120–125 °C for 5 h.18 The heating was continued under a N2 atmosphere. Then, the reaction mixture was cooled to 90 °C. Deionised water was then added under a N2 atmosphere and then it was left for 1 h. The dark red solution was filtered to remove the trace amount of unreacted PTCDA. The solution was then acidified with 2 M HCl aqueous solution until the pH of the mixture reached 2, when the precipitate was collected by suction-filtration and thoroughly washed with deionised water until the filtrate was neutral to obtain a red solid. It was then dried in air until a constant weight was observed.
Yield: 0.509 g (0.71 mmol, 71%). 1H NMR (500 MHz, DMSO-d6, 25 °C): δ = 13.07 (br, 2H; –COOH); 8.03 (s, 4H); 7.79 (s, 4H); 7.08 (d, J = 8 Hz); 6.58 (d, J = 8 Hz); 5.92 (m, 2H); 3.53–3.35 (m, 6H). 13C NMR (125MHz, DMSO-d6, 25 °C): δ = 171.38 (COOH); 162.67 (C
O); 156.42 (C–OH); 133.90, 130.94, 130.64, 128.46, 125.41, 123.66, 122.22, 115.54 (perylene core C); 54.73, 33.98. MALDI-TOF MS: calculated 720.1733 for [M (C42H26N2O10)+2H]+, found 720.4023. Anal. calculated for C42H26N2O10: C 70.19; H 3.65; N, 3.90. Found: C 70.71, H 3.68, N 3.90%.
C Results and discussion
Hydrogelation behaviour and characterization
Though there are several studies regarding the self-aggregation and live cell imaging property of the perylene bisimide-based compounds,19,20 gelation by an amino acid-containing perylene bisimide derivative has not yet been reported. In this study, pH-responsive hydrogel formation using a L-tyrosine-containing perylene bisimide-based derivative has been reported.
The compound PBI-Y was found to form hydrogel within the pH range 5.00–9.00 in 50 mM phosphate buffer solution. The minimum gelation concentration at pH 5.00 was found to be 0.27% w/v (Table S1, ESI†). It was found that the minimum gelation concentration (MGC) increased from 0.27% w/v to 3.8% w/v with an increase in pH from 5.00 to 9.00 (Table S1, ESI†). At first, the hydrogelator PBI-Y was dissolved in 1 mL phosphate buffer of 50 mM at the required pH (from 5.00 to 9.00) using sonication and heating at about 80 °C in a sealed tube. Then, the gel tube was kept at room temperature and the tube's temperature was allowed to come down to room temperature to obtain a gel phase material (Scheme 1). Fig. 1 shows the gel melting temperatures (Tgel) of this new hydrogel at different concentrations of the gelator molecule at pH 5. This plot (concentration vs. temperature) suggests that the Tgel value increases with an enhancement of the concentration of the gelator until a typical concentration is attained. This concentration is known as the “plateau region”,10d suggesting that the hydrogel network formation is basically completed and the saturation point is reached, i.e. Tgel value does not increase with the further increase in gelator concentration.
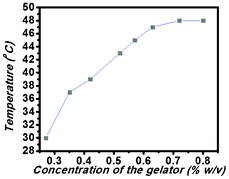 |
| Fig. 1 The Tgel profile of the PBI-Y hydrogel at different concentrations (% w/v). | |
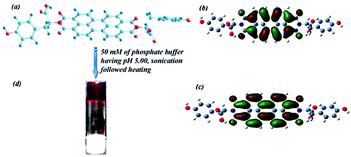 |
| Scheme 1 (a), (b) and (c) are the chemical structure, HOMO and LUMO structures, respectively, of the PBI-Y hydrogelator and (d) shows a photograph of the hydrogel in an inverted glass vial; the arrow indicates the formation of the hydrogel under the specified conditions. | |
A UV-Vis study was carried out to examine the presence of both aromatic moieties (tyrosine and perylene) into the PBI-Y hydrogel. Fig. S4a (see ESI†) shows the presence of a peak at 275 nm for the pure L-tyrosine amino acid in phosphate buffer solution at pH 7. Fig. S4b shows several peaks at 275 nm, 508 nm and 544 nm. A small peak at 275 nm indicates the presence of the tyrosine moiety. Fig. S4c shows the enlarged view of Fig. S4b showing the peak of the tyrosine only (in x-axis the wavelength has been taken from 270 to 285 nm to highlight the tyrosine). Fig. S4d (see ESI†) shows absorption peaks at 472 (the little hump), 508 and 544 nm corresponding to the core perylene moiety of the PBI-Y gelator. UV-Vis absorption of a water-soluble perylene bisimide derivative has also been studied by different research groups previously.21 They have also mentioned the perylene core peaks in the 500–550 nm region. The UV-Vis spectroscopic absorption peak pattern in the gel state of the PBI-Y hydrogelator was investigated using a thin film of gel inbetween two slides of glass. The gel state UV-Vis spectroscopic absorption profile is shown in Fig. 2.
Concentration-dependent UV-Vis studies showed no significant change of these peak positions; only the peak pattern changed with the change in intensity of major peaks. In the solution state (in a dilute solution) the peak at the 544 nm is more intense than that of the other peak at 508 nm. However, in the concentrated solution state (i.e. in the assembled state), the intensity of the peak at 508 nm is more than that of the peak at 544 nm (Fig. S4e, ESI†). pH-Dependent UV-Vis studies were also carried out to investigate the change in peak position in the gel state by varying the pH of the medium. Interestingly, the PBI-Y-based hydrogelator showed a pH-tuneable UV-Vis absorption pattern. At a lower pH of 5.00, the PBI-Y hydrogel showed peaks at 508 and 544 nm. However, upon an increase in pH from pH 5.00 to 9.00, the peak pattern changed and the previous peak at 544 nm red shifted to 610 nm, keeping the 508 nm peak intact. The pH-dependent UV-Vis absorption spectral pattern is shown in the Fig. S5 (see ESI†). This indicates the clear pH tunability of the UV-Vis peak pattern. However, in the temperature-dependent UV-Vis study, the diluted gel showed two major peaks at 508 and 544 nm, with a highly intense peak at 508 nm at 25 °C. At 85 °C, the same diluted gel showed two peaks at 502 nm and 544 nm with almost equal intensities. Interestingly, the peak at 508 nm blue shifted to 502 nm, and peaks at 502 nm and 544 nm have almost equal intensities (Fig. S6, ESI†). This experiment clearly showed that the aggregation behaviour was perturbed to a great extent with an increase in temperature with the gradual destruction of the self-assembly pattern at the elevated temperature range. At the low concentration and at the high temperature, the assembly of the gelator molecule was perturbed significantly, indicating the similar absorption spectra pattern of the gelator molecule in these two above-mentioned conditions.
Fluorescence study
Among different classes of functional π-conjugated molecules, perylene bisimides (PBI) have been widely investigated due to their strong fluorescence.6a These PBI-Y-based molecules are self-assembled using π–π stacking interactions due to the strong intermolecular interactions and they exhibit interesting optical properties.3a The fluorescence spectrum of the amino acid-containing PBI-Y-based hydrogel at pH 5.00 also showed an emission peak at 311 nm due to the presence of the tyrosine moiety22 upon the excitation at 275 nm. Excitation and emission of the tyrosine moiety are distinctly different from those of the PBI-core. Fluorescence emission and excitation spectra of L-tyrosine are shown in Fig. S4f (see ESI†) and the fluorescence emission and excitation spectra of the tyrosine moiety present in PBI-Y hydrogelator are shown in Fig. S4g (see ESI†). The fluorescence emission peak of the pure L-tyrosine appeared at 311 nm (Fig. S4f, see ESI†). The fluorescence emission peak of PBI-Y in the lower region also showed a peak at 311 nm, suggesting the presence of a tyrosine moiety within the PBI-Y-based hydrogelator. Fig. S4h shows the fluorescence emission of both the tyrosine moiety and perylene bisimide core by exciting at 275 nm. Fluorescence emission and fluorescence excitation spectra of the PBI-Y hydrogel are shown in Fig. S7 (see ESI†).
The fluorescence excitation spectrum showed a peak at 507 nm corresponding to the perylene core. However, the fluorescence emission spectrum showed a major peak at 663 nm. This can be due to the formation of excimer complex formed by the PBI moiety.14c Aggregation behaviour of the PBI-Y hydrogelator was also studied using concentration-dependent fluorescence experiments. However, in very dilute conditions the PBI-Y hydrogelator showed peaks at 551 and 593 nm upon the same excitation at 507 nm, which was used for the PBI-Y hydrogel.
The sol-to-gel transition was accompanied by the red shifting of the fluorescence emission peaks (Fig. 3). This red shifting of the PBI-Y hydrogelator from a very dilute solution (2.2 × 10−8 M) state to the gel state (4.37 × 10−3 M) may be due to the formation of an excimer-like structure, suggesting the intermolecular π–π interactions of the PBI-Y π-moieties.14c,23 At an intermediate concentration (1.1 × 10−6 M) between the dilute solution and gel state, the PBI-Y hydrogelator showed a peak at 609 nm (Fig. S8, ESI†). pH-Dependent fluorescence studies24 were also performed to monitor the change in the fluorescence spectrum with a change in pH of the medium in the gel state.
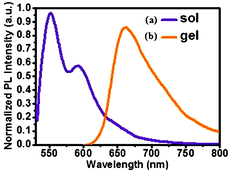 |
| Fig. 3 Fluorescence emission spectra of the PBI-Y gelator in two states (a) solution state, (b) gel state as indicated above. The excitation wavelength was kept fixed at 507 nm for both of these states. The concentration of sol state was 2.2 × 10−8 M and in the gel state was 4.37 × 10−3 M. | |
Remarkably, the fluorescence emission spectrum of the PBI-Y hydrogel changed upon the changing of the pH of the medium (Fig. 4). At pH 5.00 the PBI-Y hydrogel showed a fluorescence emission peak at 663 nm. However, with an increase in the pH from pH 5.00 to 6.00, the emission band at 663 shifted to 666 nm. At pH 7.00 the emission band at 677 nm was observed. At pH 9.00 the PBI-Y hydrogel showed emission peaks at 689 and 701 nm. Thus, the major emission peak was shifted from 663 nm to 689 nm by changing the pH from 5.00 to 9.00. This red shifting may occur due to the ionisation of the phenolic –OH moiety of the tyrosine and the formation of electron rich species tyrosinate, which may donate some charge to the electron deficient system of the centrally located perylene bisimide moiety.14a
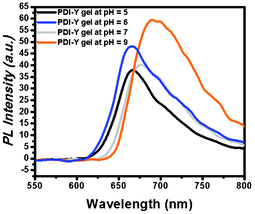 |
| Fig. 4 pH-Dependent fluorescence emission of the hydrogel material keeping the excitation wavelength at 507 nm for all cases. | |
The quantum yield25 of the hydrogel was estimated to be 0.21% with respect to rhodamine B in water (31%). Time-resolved fluorescence was measured to determine the lifetime of the hydrogel and the average decay time for the hydrogel was 0.15 ns (Fig. S9, ESI†).
Morphology
Previously, a perylene bisimide-based organogel nanofibrillar structure has been studied extensively. Rybtchinski and coworkers,16d Würthner and co-workers16a,b and others14b have reported very thin perylene bisimide-based organogel nanofibers. Morphological studies of the PBI-Y hydrogel were carried out using FE-SEM and TEM studies. FE-SEM and TEM images of the xerogel material showed the cross-linked nanofiber network structure. These fibers are several micrometers in length and widths of these gel nanofibers are different in these two (FE-SEM and TEM) different studies (Fig. 5). FE-SEM images of the gel material have revealed the presence of nanofibrillar structures. Widths of these nanofibers are ranging from 21 to 48 nm. However, TEM images of the gel show thinner fibers compared to that of the FE-SEM analysis. Widths of these fibers are within the range of 3–8 nm. The difference in width can be due to the fact that the FE-SEM analysis was carried out by using the diluted xerogel sample with a concentration of 4.4 × 10−5 M, whereas the TEM analysis was carried out by using the very diluted xerogel sample at a concentration of 8.8 × 10−6 M. Fibers are thin and separated from each other in the TEM image, while in the FE-SEM image these thin fibers are bundled together to form a slightly wider fiber. This happens because of the fact that the sample preparation conditions are different for these two microscopic experiments26 (FE-SEM and TEM).
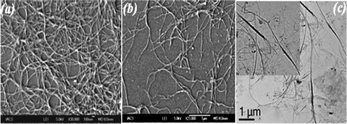 |
| Fig. 5 (a) and (b) are the FE-SEM images at a concentration of 4.4 × 10−5 M, derived from the diluted samples of PBI-Y hydrogel nanofibers and (c) is the TEM image at a concentration of 8.8 × 10−6 M, derived from the more diluted sample of the hydrogel nanofibers. | |
The FT-IR spectra of the PBI-Y hydrogelator in the solid state and the PBI-Y xerogel are shown in the Fig. S10 (see ESI†). The carbonyl stretching frequency of the hydrogelator appeared at 1698 cm−1 in the solid state, whereas in xerogel state of PBI-Y the carbonyl peak appeared at 1646 cm−1. After the gel formation a blue shift of the carbonyl peak was noticed. This can be due to the formation of H-bonding between the carbonyl group and the –OH of the carboxylic acid at pH 5.00.14a Hydrogel formation from the solution state to gel state in D2O is shown in Fig. S10(b) (see †ESI).
To understand the basic structure in gel state, X-diffraction analysis has been carried out. The Fig. 6 shows the X-diffraction pattern of the xerogel as well as in the wet gel state. In case of wet gel condition, the diffraction peak has appeared as a broad band centered at 2θ = 28.93°. The broadness of the peak may be due to the heavy scattering of water molecules. However, in xerogel state a distinct peak at 2θ = 25.17° with the d value of 3.55 Å has been observed suggesting the presence of π–π interactions into the gel state.9j This peak is absent in the powder form of the gelator material in the solid state (Fig. S11, ESI†). So, it can be stated that π–π interaction can be responsible for gelation.
Combining the results of FT-IR, X-ray diffraction and the morphological analyses using TEM, a tentative model can be put forward for the formation of gel nanofibers from the assembling gelator molecular building block. The morphology of the amino acid-containing perylene bisimide-based hydrogel is really interesting as the width of the nanofiber can be correlated with the molecular packing of the gelator molecule to obtain the assembled gel state structure consisting of the network of nanofibers. The B3LYP/6-31+G** energy-minimized structure of the PBI-Y hydrogelator, HOMO and LUMO structures are shown in the Scheme 1. The energies of the highest occupied molecular orbital (HOMO) and the lowest unoccupied molecular orbital (LUMO) are a measure of electron donor and electron acceptor capacity, respectively. HOMO and LUMO can also be reliable measures for reducing and oxidising properties of the corresponding molecule, respectively. Scheme 1(b) and (c) show the electron density distribution in the HOMO and LUMO, which is the main controlling factor for any chemical reaction. The thinner fiber diameter obtained from TEM images is comparable to the face-to-face stacking of a minimum of eight hydrogelator molecules (Scheme S1, ESI†). The molecular conformation used in Scheme 1 was obtained from the conformation of the B3LYP/6-31+G** energy minimized structure using DFT calculations.
The formation of the long thin fiber-like supramolecular structure is not only due to the π–π stacking of the perylene core, but also due to the hydrogen-bonding (see FTIR section) interactions16e (Scheme S1†). So, it can be stated that the gelator molecule was assembled mainly by using π–π stacking and intermolecular H-bonding interactions, leading to the formation of the long and thin rope-like fiber structure in the assembled gel state.
Temperature-dependent 1H NMR study
1H NMR spectroscopy is a very useful tool for examining the π–π stacking interactions within the gel.27 The temperature-dependent 1H NMR study of the hydrogel was performed using D2O as a solvent and it was associated with the shift of the aromatic proton peaks. Two broad peaks at 6.89 ppm and 7.28 ppm appeared due to the aromatic protons of the hydrogelator molecule (Fig. 7). All of these peaks were sharpened and shifted downfield with an increase in temperature. The gelator concentration for the NMR study was fixed at 0.9% w/v, corresponding to Tgel (48 °C). Two new peaks at 6.78 ppm and 7.66 ppm started appearing at 50 °C, with the downfield shift of another two peaks with an increase in temperature (Fig. 7). The shift in the 1H NMR signals at the aromatic region provides direct evidence for the contribution of π–π stacking to the formation of the gel.27 In the hydrogel, aromatic moieties of the core perylene and two tyrosine residues were π–π stacked in such a way that the proton signals appeared broad. The elevated temperature of the hydrogel resulted in the deformation of the π–π stacking. Thus, these aromatic proton signals appeared as sharper peaks at high temperature. The increase in temperature resulted in the appearance of the sol states. Aromatic proton signals in the sol state appeared at 6.78 ppm and 7.66 ppm due to the formation of free aromatic protons, without any π–π stacking interactions. So, it can be stated from the temperature-dependent 1H NMR studies that the π–π stacking of the aromatic moieties is involved during the gel formation and this π–π stacking interaction is absent in the sol state.
Rheological behaviour
Amino acid- and peptide-based molecules are generally biologically active molecules and soft materials made up of these biologically important molecules can be treated as biomaterials with interesting properties. The fundamental mechanical properties of a gel can be expressed using rheology.28 The dynamics and strength of the network structure determine the viscoelastic property of the hydrogel and so it is important to study the hydrogel using rheological experiments. Rheological studies were carried out at a constant oscillatory frequency of 1 Hz at room temperature (25 °C). These studies represent the flow behaviour and viscoelastic properties of the hydrogel. Fig. 8 reveals the rheological properties. The storage modulus (G′) and loss modulus (G′′) were plotted against the angular frequency (ω) of the hydrogel (Fig. 8). If G′ (storage modulus) is proportional to angular frequency (ω), G′′ (loss modulus) is also proportional to angular frequency (ω) and, simultaneously, G′ > G′′ (at a low frequency region), the system is considered to behave as a gel. The storage modulus (G′) and the loss of modulus (G′′) were found to be almost parallel to the angular frequency (ω) applied to the total range done during the experiment. This suggests that both G′ and G′′ are almost independent of the applied angular frequency under the experimental conditions. Fig. 8 shows that the storage modulus (G′) is in the range of 6 × 102 Pa, whereas the loss modulus (G′′) is in the range of 24 Pa initially. This data clearly indicates that the PBI-Y system behaves as a gel.28
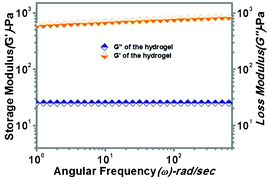 |
| Fig. 8 The storage modulus (G′) and loss modulus (G′′) vs. angular frequency profile for the PBI-Y supramolecular hydrogel at 0.5% w/v. | |
The electrochemical property of the PBI-Y hydrogelator was studied by cyclic voltammetry analysis using a glassy carbon as a working electrode, Pt as a counter electrode, Ag/AgCl (KCl) as a reference electrode and 0.1 (M) NaCl as the supporting electrolyte. A solution of the PBI-Y hydrogelator was prepared in 50 mM phosphate buffer in different pHs from 5.00 to 9.00 (Fig. 9). At pH 5.00 the PBI-Y hydrogelator solution shows irreversible reduction potential at −0.65 and −1.22 V and irreversible oxidation potential at 1.65 and 0.41 V. There is a change in both reduction and oxidation potentials with an increase in pH. At pH 6.00 the irreversible reduction potential at −0.45 and −1.35 V and irreversible oxidation potential at 1.39 and 0.34 V were observed. At pH 9.00 the irreversible reduction potential at −1.01 and −1.61 V and irreversible oxidation potential at 1.57 and 0.51 V were found. The change of reduction potential upon changing pH may be due to the fact that at higher pH (pH = 9.00) the redox-active tyrosine moiety has become tyrosinate.
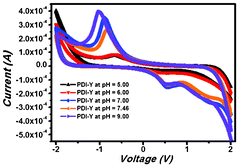 |
| Fig. 9 The pH-dependent cyclic voltammetric study of the hydrogel in the solution state. | |
Thus, the reduction potential was increased to a considerable extent. It is noteworthy that the oxidation behaviour is not so pronounced in each case. However, the reduction behaviour is significant. Previous reports on naphthalene bisimide-based molecules have shown negative values in the cyclic voltammetric study and these results are analogous to our findings.29
Photo-response study
For the photo-response study, a simple conductor device in lateral geometry was fabricated by evaporating two Au contacts of area 1 mm2 separated by a distance of 3 mm on a pellet made of PBI-Y xerogel (the inset in Fig. 10). Then, the required light from a Xenon lamp (model no. 66902; Newport Corp. USA) was shined on the conductor area to measure the photo-response property. The current between the two contacts were measured using a Keithley source meter (model 2401). The dark current shows a perfect straight line that is symmetric in both positive and negative bias conditions, confirming the formation of Ohmic contacts. Under both visible and white light illumination, the current conduction increases, indicating that the PBI-Y xerogel is highly photo-responsive, as shown in Fig. 10. The temporal photo-response curve in Fig. 10 at 5 V bias condition shows that as soon as the light is switched on, the photocurrent increases sharply both for the visible (UV light was filtered) and white light (without filtering the UV light). When the light is switched off after 30 s, the current falls sharply. The current can be reversibly turned ‘ON’ and ‘OFF’ as the light is switched on and off (Fig. 11). The ON/OFF ratio is quite high, such as 18 and 51 for visible and white lights, respectively. This demonstrates an excellent photo-switching phenomenon for such a water-soluble PBI-Y xerogel, which has not yet been reported.
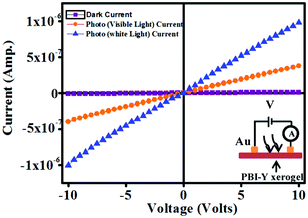 |
| Fig. 10 Plots of I–V curves of a simple conductor device made of PBI-Y xerogel in dark, visible and white light conditions. Inset: A schematic representation of the layout of the circuit diagram for I–V measurements. | |
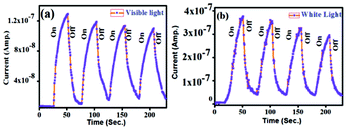 |
| Fig. 11 (a) and (b) Transient photo-response by turning on/off visible and white light illumination of a representative conductor device made of the PBI-Y xerogel, respectively. | |
Conclusions
An amino acid-containing new perylene bisimide-based hydrogelator molecule (PBI-Y) was discovered. The hydrogelator formed a pH-responsive supramolecular fluorescent hydrogel within the pH range 5.00–9.00 in the presence of 50 mM phosphate buffer. Changing the pH can modulate the fluorescence emission behaviour of the hydrogel. The hydrogel was characterized using FE-SEM, TEM, FT-IR, X-ray diffraction and rheological studies. Morphological studies of the gel revealed the formation of a network structure consisting of cross-linked nanofibers. The PBI-Y-based conductor shows excellent photo-switching behaviour towards visible, as well as white light with a high ON/OFF ratio, exhibiting its promising application for the development of photo-detectors and other applications in photo-based electronics.
Acknowledgements
S. R, D. K. M and S. P gratefully acknowledge CSIR, New Delhi, India for financial assistance. We acknowledge DST, India for financial assistance. We thank Mr. Bappaditya Roy of the Department of Polymer Science unit, IACS for rheological measurement. We also thank Mr. Sumit Ray, IACS for recording temperature-dependent 1H NMR data. We also acknowledge Mr. Debasish Mandal (Department of Spectroscopy, IACS) for B3LYP/6-31+G** calculations.
References
-
(a) A. Dawn, T. Shiraki, H. Ichikawa, A. Takada, Y. Takahashi, Y. Tsuchiya, L. T. N. Lien and S. Shinkai, J. Am. Chem. Soc., 2012, 134, 2161–2171 CrossRef CAS;
(b) C. J. Bowerman and B. L. Nilsson, J. Am. Chem. Soc., 2010, 132, 9526–9527 CrossRef CAS;
(c) H. Cui, M. J. Webber and S. I. Stupp, Biopolymers, 2010, 94, 1–18 CrossRef CAS;
(d) K. Rajagopal, M. S. Lamm, L. A. Haines-Butterick, D. J. Pochan and J. P. Schneider, Biomacromolecules, 2009, 10, 2619–2625 CrossRef CAS;
(e) A. Aggeli, M. Bell, L. M. Carrick, C. W. G. Fishwick, R. Harding, P. J. Mawer, S. E. Radford, A. E. Strong and N. Boden, J. Am. Chem. Soc., 2003, 125, 9619–9628 CrossRef CAS;
(f) B. Ozbas, J. Kretsinger, K. Rajagopal, J. P. Schneider and D. J. Pochan, Macromolecules, 2004, 37, 7331–7337 CrossRef CAS;
(g) D. J. Pochan, J. P. Schneider, J. Kretsinger, B. Ozbas, K. Rajagopal and L. Haines, J. Am. Chem. Soc., 2003, 125, 11802–11803 CrossRef CAS;
(h) L. A. Haines, K. Rajagopal, B. Ozbas, D. A. Salick, D. J. Pochan and J. P. Schneider, J. Am. Chem. Soc., 2005, 127, 17025–17029 CrossRef CAS;
(i) Y. Gao, Z. Yang, Y. Kuang, M.-L. Ma, J. Li, F. Zhao and B. Xu, Biopolymers, 2009, 94, 19–31 CrossRef.
-
(a) Y. Li, J. Rodrigues and H. Tomás, Chem. Soc. Rev., 2012, 41, 2193–2221 RSC;
(b) Z. L. Wu and J. P. Gong, NPG Asia Mater., 2011, 3, 57–64 CrossRef;
(c) M. Guvediren, H. D. Lu and J. A. Burdick, Soft Matter, 2012, 8, 260–272 RSC;
(d) S. V. Vlierberghe, P. Dubruel and E. Schacht, Biomacromolecules, 2011, 12, 1387–1408 CrossRef.
-
(a) A. C. Grimsdale and K. Müllen, Angew. Chem., Int. Ed., 2005, 44, 5592–5629 CrossRef CAS;
(b) A. P. H. J. Schenning and E. W. Meijer, Chem. Commun., 2005, 3245–3258 RSC;
(c) F. J. M. Hoeben, P. Jonkheijm, E. W. Meijer and A. P. H. J. Schenning, Chem. Rev., 2005, 105, 1491–1546 CrossRef CAS;
(d) P. E. Keivanidis, V. Kamm, W. Zhang, G. Floudas, F. Laquai, I. McCulloch, D. D. C. Bradley and J. Nelson, Adv. Funct. Mater., 2012, 22, 2318–2326 CrossRef CAS;
(e) C. Li and H. Wonneberger, Adv. Mater., 2012, 24, 613–636 CrossRef CAS;
(f) H. Usta, A. Facchetti and T. J. Marks, Acc. Chem. Res., 2011, 44, 501–510 CrossRef CAS.
-
(a) A. Ajayaghosh, V. K. Praveen, C. Vijayakumar and S. J. George, Angew. Chem., Int. Ed., 2007, 46, 6260–6265 CrossRef CAS;
(b) L. Chen, S. Revel, K. Morris and D. J. Adams, Chem. Commun., 2010, 46, 4267–4269 RSC;
(c) A. Ajayaghosh, V. K. Praveen, S. Srinivasan and R. Varghese, Adv. Mater., 2007, 19, 411–415 CrossRef CAS;
(d) K. V. Rao, K. Jayaramula, T. K. Maji and S. J. George, Angew. Chem., Int. Ed., 2010, 49, 4218–4222 CrossRef CAS;
(e) D. T. McQuade, A. E. Pullen and T. M. Swager, Chem. Rev., 2000, 100, 2537–2574 CrossRef CAS;
(f) T. M. Swager, Acc. Chem. Res., 1998, 31, 201–207 CrossRef CAS;
(g) J.-L. Wang, J. Yan, Z.-M. Tang, Q. Xiao, Y. Ma and J. Pei, J. Am. Chem. Soc., 2008, 130, 9952–9962 CrossRef CAS.
-
(a) A. Donat-Bouillud, I. Lévesque, Y. Tao and M. D'Iorio, Chem. Mater., 2000, 12, 1931–1936 CrossRef CAS;
(b) M. Gross, D. C. Müller, H.-G. Nothofer, U. Scherf, D. Neher, C. Bräuchle and K. Meerholz, Nature, 2000, 405, 661–665 CrossRef CAS.
-
(a) F. Würthner and M. Stolte, Chem. Commun., 2011, 47, 5109–5115 RSC;
(b) R. P. Ortiz, H. Herrera, R. Blanco, H. Huang, A. Facchetti, T. J. Marks, Y. Zheng and J. L. Segura, J. Am. Chem. Soc., 2010, 132, 8440–8452 CrossRef CAS;
(c) A. Facchetti, M.-H. Yoon and T. J. Marks, Adv. Mater., 2005, 17, 1705–1725 CrossRef CAS;
(d) S. A. Ponomarenko, S. Kirchmeyer, A. Elschner, B.-H. Huisman, A. Karbach and D. Drechsler, Adv. Funct. Mater., 2003, 13, 591–596 CrossRef CAS.
-
(a) A. Liscio, G. D. Luca, F. Nolde, V. Palermo, K. Müllen and P. Samori, J. Am. Chem. Soc., 2008, 130, 780–781 CrossRef CAS;
(b) L. Schmidt-Mende, A. Fechtenkötter, K. Müllen, E. Moons, R. H. Friend and J. D. MacKenzie, Science, 2001, 293, 1119–1122 CrossRef CAS;
(c) A. Leliège, P. Blanchard, T. Rousseau and J. Roncali, Org. Lett., 2011, 13, 3098–3101 CrossRef.
-
(a) F. Würthner, B. Hanke, M. Lysetska, G. Lambright and G. S. Harms, Org. Lett., 2005, 7, 967–970 CrossRef;
(b) T. Tang, J. Qu, K. Müllen and S. E. Webber, Langmuir, 2006, 22, 7610–7616 CrossRef CAS;
(c) E. Fron, M. Lor, R. Pilot, G. Schweitzer, H. Dincalp, S. D. Feyter, J. Cremer, P. Bäuerle, K. Müllen, M. V. d. Auweraer and F. C. D. Schryver, Photochem. Photobiol. Sci., 2005, 4, 61–68 RSC;
(d) F. Würthner, Chem. Commun., 2004, 1564–1579 RSC;
(e) F. Würthner, C. Thalacker, A. Sautter, W. Schärtl, W. Ibach and O. Hollricher, Chem.–Eur. J., 2000, 6, 3871–3886 Search PubMed.
-
(a) X. Du, J. Li, Y. Gao, Y. Kuang and B. Xu, Chem. Commun., 2012, 48, 2098–2100 RSC;
(b) X. Li, Y. Kuang and B. Xu, Soft Matter, 2012, 8, 2801–2806 RSC;
(c) F. Zhao, Gao, Y. J. Shi, H. M. Browdy and B. Xu, Langmuir, 2011, 27, 1510–1512 CrossRef CAS;
(d) D. J. Adams, Macromol. Biosci., 2011, 11, 160–173 CrossRef CAS;
(e) D. J. Adams and P. D. Topham, Soft Matter, 2010, 6, 3707–3721 RSC;
(f) G. Cheng, V. Castelletto, C. M. Moulton, G. E. Newby and I. W. Hamley, Langmuir, 2010, 26, 4990–4998 CrossRef CAS;
(g) S. Roy and A. Banerjee, RSC Adv., 2012, 2, 2105–2111 RSC;
(h) S. Bhowmik and U. Maitra, Chem. Commun., 2012, 48, 4624–4626 RSC;
(i) A. Srivastava, S. Ghorai, A. Bhattacharjya and S. Bhattacharya, J. Org. Chem., 2005, 70, 6574–6582 CrossRef CAS;
(j) J. Naskar, G. Palui and A. Banerjee, J. Phys. Chem. B, 2009, 113, 11787–11792 CrossRef CAS.
-
(a) D. M. Ryan, T. M. Doran and B. L. Nilsson, Chem. Commun., 2011, 47, 475–477 RSC;
(b) S. Sutton, N. L. Campbell, A. I. Cooper, M. Kirkland, W. J. Frith and D. J. Adams, Langmuir, 2009, 25, 10285–10291 CrossRef CAS;
(c) J. Shi, Y. Gao, Z. Yang and B. Xu, Beilstein J. Org. Chem., 2011, 7, 167–172 CrossRef CAS;
(d) S. Roy and A. Banerjee, Soft Matter, 2011, 7, 5300–5308 RSC.
-
(a) J. Nanda and A. Banerjee, Soft Matter, 2012, 8, 3380–3386 RSC;
(b) B. Adhikari and A. Banerjee, Soft Matter, 2011, 7, 9259–9266 RSC;
(c) B. Adhikari, G. Palui and A. Banerjee, Soft Matter, 2009, 5, 3452–3460 RSC;
(d) G. Palui, J. Nanda, S. Ray and A. Banerjee, Chem.–Eur. J., 2009, 15, 6902–6909 CrossRef CAS;
(e) B. Adhikari and A. Banerjee, Chem.–Eur. J., 2010, 16, 13698–13705 CrossRef CAS.
-
(a) Z. Yang, K. Xu, L. Wang, H. Gu, H. Wei, M. Zhang and B. Xu, Chem. Commun., 2005, 4414–4416 RSC;
(b) M. Zhou, A. M. Smith, A. K. Das, N. W. Hodson, R. F. Collins, R. V. Ulijn and J. E. Gough, Biomaterials, 2009, 30, 2523–2530 CrossRef CAS;
(c) V. Jayawarna, M. Ali, T. A. Jowitt, A. F. Miller, A. Saiani, J. E. Gough and R. V. Ulijn, Adv. Mater., 2006, 18, 611–614 CrossRef CAS;
(d) V. Jayawarna, S. M. Richardson, A. R. Hirst, N. W. Hodson, A. Saiani, J. E.Gough and R. V. Ulijn, Acta Biomater., 2009, 5, 934–943 CrossRef CAS;
(e) Z. Xie, A. Zhang, L. Ye, X. Wang and Z.-g. Feng, J. Mater. Chem, 2009, 19, 6100–6102 RSC;
(f) N. Amdursky, E. Gazit and G. Rosenman, Adv. Mater., 2010, 22, 2311–2315 CrossRef CAS;
(g) M. Zhou, A. M. Smith, A. K. Das, N. W. Hodson, R. F. Collins, R. V. Ulijn and J. E. Gough, Biomaterials, 2009, 30, 2523–2530 CrossRef CAS.
-
(a) S. Prasanthkumar, A. Saeki, S. Seki and A. Ajayaghosh, J. Am. Chem. Soc., 2010, 132, 8866–8867 CrossRef CAS;
(b) S. S. Babu, S. Prasanthkumar and A. Ajayaghosh, Angew. Chem., Int. Ed., 2012, 51, 1766–1776 CrossRef CAS;
(c) S. Yagai, T. Kinoshita, Y. Kikkawa, T. Karatsu, A. Kitamura, Y. Honsho and S. Seki, Chem.–Eur. J., 2009, 15, 9320–9324 CrossRef CAS;
(d) Y. Che, A. Datar, X. Yang, T. Naddo, J. Zhao and L. Zang, J. Am. Chem. Soc., 2007, 129, 6354–6355 CrossRef CAS;
(e) Y. Che, X. Yang, G. Liu, C. Yu, H. Ji, J. Zuo, J. Zhao and L. Zang, J. Am. Chem. Soc., 2010, 132, 5743–5750 CrossRef CAS;
(f) Y. Che, H. Huang, M. Xu, C. Zhang, B. R. Bunes, X. Yang and L. Zang, J. Am. Chem. Soc., 2011, 133, 1087–1091 CrossRef CAS.
-
(a) Y. Sun, C. He, K. Sun, Y. Li, H. Dong, Z. Wang and Z. Li, Langmuir, 2011, 27, 11364–11371 CrossRef CAS;
(b) K. Sugiyasu, S.-i. Kawano, N. Fujita and S. Shinkai, Chem. Mater., 2008, 20, 2863–2865 CrossRef CAS;
(c) L. Xue, H. Wu, Y. Shi, H. Liu, Y. Chen and X. Li, Soft Matter, 2011, 7, 6213–6221 RSC;
(d) D. González-Rodríguez and A. P. H. J. Schenning, Chem. Mater., 2011, 23, 310–325 CrossRef;
(e) S. Yagai, Y. Monma, N. Kawauchi, T. Karatsu and A. Kitamura, Org. Lett., 2007, 9, 1137–1140 CrossRef CAS.
-
(a) T. Heek, C. Fasting, C. Rest, X. Zhang, F. Würthner and R. Haag, Chem. Commun., 2010, 46, 1884–1886 RSC;
(b) C. D. Schmidt, C. Böttcher and A. Hirsch, Eur. J. Org. Chem., 2009, 5337–5349 CrossRef CAS;
(c) X. Zhang, S. Rehm, M. M. Safont-Sempere and F. Würthner, Nat. Chem., 2009, 1, 623–629 CrossRef CAS;
(d) J. Qu, C. Kohl, M. Pottek and K. Müllen, Angew. Chem., Int. Ed., 2004, 43, 1528–1531 CrossRef CAS;
(e) M. A. Abdalla, J. Bayer, J. O. Rädler and K. Müllen, Angew. Chem., Int. Ed., 2004, 43, 3967–3970 CrossRef CAS;
(f) T. Tang, K. Peneva, K. Müllen and S. E. Webber, J. Phys. Chem. A, 2007, 111, 10609–10614 CrossRef CAS;
(g) K. Peneva, G. Mihov, A. Herrmann, N. Zarrabi, M. Börsch, T. M. Duncan and K. Müllen, J. Am. Chem. Soc., 2008, 130, 5398–5399 CrossRef CAS.
-
(a) X.-Q. Li, X. Zhang, S. Ghosh and F. Würthner, Chem.–Eur. J., 2008, 14, 8074–8078 CrossRef CAS;
(b) F. Würthner, C. Bauer, V. Stepanenko and S. Yagai, Adv. Mater., 2008, 20, 1695–1698 CrossRef;
(c) K. Sugiyasu, N. Fujita and S. Shinkai, Angew. Chem., Int. Ed., 2004, 43, 1229–1233 CrossRef CAS;
(d) E. Krieg, E. Shirman, H. Weissman, E. Shimoni, S. G. Wolf, I. Pinkas and B. Rybtchinski, J. Am. Chem. Soc., 2009, 131, 14365–14373 CrossRef CAS;
(e) H. Wu, L. Xue, Y. Shi, Y. Chen and X. Li, Langmuir, 2011, 27, 3074–3082 CrossRef CAS;
(f) O. Simalou, X. Zhao, R. Lu, P. Xue, X. Yang and X. Zhang, Langmuir, 2009, 25, 11255–11260 CrossRef CAS.
- P. K. Sukul, D. Asthana, P. Mukhopadhyay, D. Summa, L. Muccioli, C. Zannoni, D. Beljonne, A. E. Rowan and S. Malik, Chem. Commun., 2011, 47, 11858–11860 RSC.
- C. Xue, M. Chen and S. Jin, Polymer, 2008, 49, 5314–5321 CrossRef CAS.
- C. Lemouchi, S. Simonov, L. Zorina, C. Gautier, P. Hudhomme and P. Batail, Org. Biomol. Chem., 2011, 9, 8096–8101 CAS.
- B. Gao, H. Li, H. Liu, L. Zhang, Q. Bai and X. Ba, Chem. Commun., 2011, 47, 3894–3896 RSC.
-
(a) T. Heek, C. Fasting, C. Rest, X. Zhang, F. Würthner and R. Haag, Chem. Commun., 2010, 46, 1884–1886 RSC;
(b) C. Backes, F. Hauke and A. Hirsch, Adv. Mater., 2011, 23, 2588–2601 CrossRef CAS.
- T. Härd, V. Hsu, M. H. Sayre, E. P. Geiduschek, K. Appelt and D. R. Kearns, Biochemistry, 1989, 28, 396–406 CrossRef.
- K. Balakrishnan, A. Datar, R. Oitker, H. Chen, J. Zuo and L. Zang, J. Am. Chem. Soc., 2005, 127, 10496–10497 CrossRef CAS.
- A. Bernet, R. Q. Albuquerque, M. Behr, S. T. Hoffmann and H.-W. Schmidt, Soft Matter, 2012, 8, 66–69 RSC.
- D. Magde, G. E. Rojas and P. G. Seybold, Photochem. Photobiol., 1999, 70, 737–744 CrossRef CAS.
- S. Malik, S.-i. Kawano, N. Fujita and S. Shinkai, Tetrahedron, 2007, 63, 7326–7333 CrossRef CAS.
-
(a) Y. Feng, Z.-T. Liu, J. Liu, Y.-M. He, Q.-Y. Zheng and Q.-H. Fan, J. Am. Chem. Soc., 2009, 131, 7950–7951 CrossRef CAS;
(b) K.-P. Tseng, M.-T. kao, T. W. T. Tsai, C.-H. Hsu, J. C. C. Chan, J.-J. Shyue, S.-S. Sun and K.-T. Wong, Chem. Commun., 2012, 48, 3515–3517 RSC;
(c) S. S. Babu, V. K. Praveen, S. Prasanthkumar and A. Ajayaghosh, Chem.–Eur. J., 2008, 14, 9577–9584 CrossRef CAS.
-
(a) P. Terech, D. Pasquier, V. Bordas and C. Rossat, Langmuir, 2000, 16, 4485–4494 CrossRef CAS;
(b) C. Yan and D. J. Pochan, Chem. Soc. Rev., 2010, 39, 3528–3540 RSC;
(c) G. Palui, A. Garai, J. Nanda, A. K. Nandi and A. Banerjee, J. Phys. Chem. B, 2010, 114, 1249–1256 CrossRef CAS;
(d) B. Roy, P. Bairi, A. Saha and A. K. Nandi, Soft Matter, 2011, 7, 8067–8076 RSC.
- C. Thalacker, C. Röger and F. Würthner, J. Org. Chem., 2006, 71, 8098–8105 CrossRef CAS.
|
This journal is © The Royal Society of Chemistry 2012 |
Click here to see how this site uses Cookies. View our privacy policy here.