DOI:
10.1039/C2MT20151H
(Paper)
Metallomics, 2013,
5, 43-51
Localization of copper and copper transporters in the human brain†
Received
26th July 2012
, Accepted 3rd October 2012
First published on 3rd October 2012
Abstract
Disturbances in brain copper result in rare and severe neurological disorders and may play a role in the pathogenesis and progression of multiple neurodegenerative diseases. Our current understanding of mammalian brain copper transport is based on model systems outside the central nervous system and no data are available regarding copper transport systems in the human brain. To address this deficit, we quantified regional copper concentrations and examined the distribution and cellular localization of the copper transport proteins Copper transporter 1, Atox1, ATP7A, and ATP7B in multiple regions of the human brain using inductively coupled plasma-mass spectrometry, Western blot and immunohistochemistry. We identified significant relationships between copper transporter levels and brain copper concentrations, supporting a role for these proteins in copper transport in the human brain. Interestingly, the substantia nigra contained twice as much copper than that in other brain regions, suggesting an important role for copper in this brain region. Furthermore, ATP7A levels were significantly greater in the cerebellum, compared with other brain regions, supporting an important role for ATP7A in cerebellar neuronal health. This study provides novel data regarding copper regulation in the human brain, critical to understand the mechanisms by which brain copper levels can be altered, leading to neurological disease.
Introduction
Copper is an essential micronutrient and transition metal required for various cellular functions including cellular respiration, free-radical defense, neurotransmitter synthesis, neuronal myelination, and iron metabolism. In excess of cellular requirements, however, copper is toxic because it can participate in reactions that generate free radicals, thus contributing to oxidative stress.1 Neuronal health and function thus depends on cellular mechanisms that regulate copper uptake, distribution, utilisation, storage, detoxification and efflux in the brain.
Compromised copper transport in the human brain is manifested as severe and well-characterized neurodegenerative disorders, including Menkes disease2 and Wilson's disease.3 A growing body of evidence suggests that, in addition to these disorders, disturbances in the distribution of brain copper levels are associated with other neurodegenerative diseases,4 including Alzheimer's disease,5,6 amyotrophic lateral sclerosis (ALS),7,8 Parkinson's disease,9,10 Huntington's disease,10 and prion diseases.11 Alterations in brain copper levels enhance cell death mechanisms in these disorders, including protein aggregation,12–14 oxidative stress5,15 and mitochondrial dysfunction,16 but the homeostatic control of copper in the human brain has not been determined.
To address this deficit, we describe here the associations between regional copper concentrations with the levels and cellular localization of major copper transport proteins Copper transporter 1 (Ctr1), Atox1, and the ATPases, ATP7A and ATP7B. An understanding of copper transport in the normal adult human brain will be essential for understanding diseases where brain copper levels are altered and for the development of more effective treatments for these disorders.
Experimental
Brain tissues
Consent to use autopsy material for research purposes was obtained for all human brain samples. Tissues were received from New South Wales Tissue Resource Centre at the University of Sydney and the Sydney Brain Bank at Neuroscience Research Australia. Autopsy was performed within 48 hours of death and, to prevent metal contamination, brain tissues were dissected using polytetrafluoroethylene-coated blades (ProSciTech, Queensland, Australia). Fresh tissues were frozen immediately in liquid nitrogen, then stored at −80 °C prior to analysis. Fixed tissues were stored in formalin. Ten human brains from subjects free of neurological disorders and neuropathological abnormalities were investigated. The details of these cases are given in Table 1. Brain regions investigated included the cerebellum, body of caudate, putamen, visual cortex, anterior cingulate cortex, and substantia nigra. Substantia nigra tissue was provided from only five of these ten human brains, as described in Table 1.
Table 1 Case details
Case |
Age (years) |
Sex |
Post-mortem interval (hours) |
Brain pH |
Cause of death |
All cases had no significant neuropathology at autopsy. Substantia nigra tissue was provided from cases marked with an asterisk. |
1* |
41 |
Male |
48 |
6.96 |
Ischaemic heart disease |
2* |
49 |
Male |
47 |
6.5 |
Coronary atherosclerosis |
3* |
65 |
Male |
14.5 |
6.79 |
Ischaemic heart disease |
4 |
79 |
Male |
8 |
6.65 |
Pulmonary embolism |
5 |
84 |
Female |
6 |
6.51 |
Respiratory arrest |
6 |
85 |
Female |
23 |
6.44 |
Pneumonia |
7* |
92 |
Female |
5 |
6.08 |
Pancytopaenia |
8 |
93 |
Female |
21 |
6.96 |
Cardiac failure |
9 |
97 |
Female |
16 |
Not available |
Pneumonia |
10* |
102 |
Female |
5 |
5.92 |
Acute renal failure |
Materials
Primary antibodies used for detection of copper transport proteins by Western blotting, immunohistochemistry (IHC), and immunofluorescence (IF) are detailed in Table 2. Specificity of each antibody was confirmed by including no primary controls for Western blotting, IHC, and IF. Western blot assay was performed on brain tissue following preadsorption of the Atox1 antibody with the antigen against which it was raised, and the ATPase antibodies have been well characterized in previous reports.17,18 Horseradish peroxidase-conjugated antibodies used for detection of proteins by Western blotting include: mouse anti-goat IgG, goat anti-mouse IgG, and donkey anti-sheep/goat IgG (all Millipore, USA). Secondary antibodies used for IHC include; biotinylated horse anti-goat IgG (H+L) and biotinylated rabbit anti-sheep IgG (H+L) (both Vector Laboratories Inc., CA, USA). Vectastain ABC Kit Elite was used for IHC (Vector Laboratories Inc., CA). Donkey anti-goat Alexa Fluor® 594 IgG (H+L) and donkey anti-rabbit Alexa Fluor® 488 IgG (H+L), were purchased from Invitrogen (Eugene, Oregon, USA), for detection of Ctr1 and TH by IF, respectively.
Table 2 Primary antibodies used in this study
Antigen |
Immunogen |
Source |
Ctr1 |
Raised against a peptide mapping within amino acids 100–150 of CTR1 of human origin (accession number O15431). |
Santa Cruz Biotechnology, Inc. (CA, USA); CTR1 (G-15): Cat. # sc-18473; goat polyclonal. |
Atox1 |
ATOX1 (NP_004036, 1 a.a.–68 a.a.) partial recombinant protein with GST tag. |
Abnova (Taiwan); ATOX1, clone 2E6; Cat. # H00000475-M01; mouse monoclonal. |
ATP7A |
Raised against the first 590 N-terminal amino acids of the human MNK protein. |
Provided by Julian Mercer, Deakin University, Melbourne, Australia; R17; sheep polyclonal.18 |
ATP7B |
Raised against a 36-kDa fusion protein with a His6 tag at the N-terminus of the protein comprising amino acids 1–199 (N-terminal), 1309–1315 and 1376–1465 (C-terminal) of the human ATP7B protein. |
Provided by Julian Mercer, Deakin University, Melbourne, Australia; NC36; sheep polyclonal.17 |
β-actin |
Raised against amino acids 50–70 of purified chicken gizzard actin. |
Millipore (Billerica, MA, USA); anti-actin, clone C4; Cat. # MAB1501; mouse monoclonal. |
TH |
Raised against amino acids 1–196 of TH of human origin. |
Santa Cruz Biotechnology, Inc. (CA, USA); TH (H-196): sc-14007; rabbit polyclonal. |
Whole tissue metal content
Samples of frozen brain (34–70 mg) were subjected to closed-vessel microwave digestion (Milestone MLS1200) in 3 mL of concentrated nitric acid and 1 mL 35% hydrogen peroxide (both Seastar Chemicals, British Columbia, Canada). Digests were diluted w/w to ca. 50 g with 1% nitric acid, and total metal concentrations were determined using an Agilent Technologies 7500cs inductively coupled plasma mass spectrometer (ICP-MS) (Forrest Hill, Victoria, Australia). Measured masses (m/z) included 55, 57, 63, 64, 65, 66 and 82, representing 55Mn, 57Fe, 63Cu, 64Zn, 65Cu, 66Zn, and 82Se isotopes. Potential polyatomic interferences were removed using a He collision gas, specifically 40Ar16OH+ on 57Fe, 23Na40Ar+ on 63Cu, and 32S2+ and 32SO2+ on 64Zn. Routine tuning of the ICP-MS prior to use with a standard solution of 7Li, 59Co, 89Y, 140Ce and 205Tl in a 1% HNO3/HCl matrix. Matrix-based (m/z 94/59, 59Co35Cl+/59Co+) and spectral (m/z 156/140, 140Ce16O+/140Ce+) interference formation was maintained at <1% at a He flow rate of 6 mL min−1. Reported Cu and Zn concentrations are the average of m/z 63 and 65; and 64 and 66, respectively, with both metals conserving natural isotopic abundance ratios. Analytical performance was determined by measuring the detection limits (DL) and background equivalent concentration (BEC) for each measured mass (Table S1, ESI†). Mean elemental concentrations are shown in μg g−1 wet weight ± standard error of the mean (SEM).
Preparation of brain tissue homogenate
Tissue homogenates were prepared in 15 volumes of buffer solution (10 mM Tris–HCl, 1% SDS, pH 7.5), protease inhibitor cocktail (Sigma) and leupeptin (25 μm final concentration) using a hand-held electronic homogenizer with a polycarbonate probe (OmniTH, Kelly Scientific, Australia). Homogenates were centrifuged at 15
700g for 10 min at 4 °C, supernatants collected and stored at −80 °C. Pierce® BCA Protein Assay Kit (Thermo Scientific) was used to determine sample protein concentration.
SDS-PAGE and Western blot analysis
The following protein amounts were loaded onto 4–12% Bis–Tris Criterion pre-cast gels (Bio-Rad); Ctr1: 40 μg, Atox1: 80 μg, ATP7A: 120 μg, ATP7B: 120 μg, and separated by SDS-PAGE in MES (Atox1) or MOPS (Ctr1, ATP7A, ATP7B) buffer (Bio-Rad), according to manufacturer's instructions (Bio-Rad). Separated proteins were transferred to Immobilon-PSQ PVDF (Millipore) (Ctr1, Atox1, ATP7A) or nitrocellulose (Millipore) (ATP7B) membranes. Following transfer, membranes were blocked in either 5% skim milk (Ctr1, ATP7A, and ATP7B), or 1% casein (Atox1), in PBS containing 0.1% Tween® 20 and incubated with appropriate primary antibodies diluted in PBS containing 0.1% Tween® 20 and 1% skim milk (Ctr1, ATP7A, and ATP7B) or 1% casein (Atox1) at the following concentrations: Ctr1 (1:200), Atox1 (1:250), ATP7A (1:750), and ATP7B (1:500) overnight at 4 °C. Following incubation with horseradish peroxidase-conjugated mouse anti-goat IgG (1:10000), goat anti-mouse IgG (1:2000), or donkey anti-sheep/goat IgG (1:2000), protein signals were obtained using an ECL Western blotting detection system (Bio-Rad) as per the manufacturer's instruction's, and developed using the Chemi-Doc XRS (Bio-Rad). Signal intensities were quantified by densitometry using Quantity One® software (Bio-Rad) and normalized to β-actin (1:10000) levels.
Immunohistochemistry
Formalin-fixed slide-mounted paraffin-embedded sections (7 μm) were antigen retrieved by the following methods: Tris–EDTA buffer (0.01 M Tris, 1 mM EDTA, pH 9.0) at 95 °C for 20 min (Ctr1), citrate buffer (pH 6.0) microwave irradiation for 15 min (ATP7A and ATP7B). Non-specific peroxidase was blocked with 1% H2O2 (Fronine Laboratory Supplies) in 50% ethanol for 30 min at room temperature. Non-specific antigen sites were blocked with a mixture of 1% bovine serum albumin (BSA; Sigma) and 5% normal horse serum (NHS; Australis) (Ctr1), Animal Free Blocker™ (Vector) (ATP7A), or 3% BSA (Sigma) (ATP7B), for 20 min at room temp. Primary antibodies for IHC were used at the following dilutions: Ctr1 (1:100), ATP7A (1:300), ATP7B (1:1000). Primary antibodies for Ctr1, ATP7A and ATP7B were detected using biotinylated IgG antibodies (Vector) (1:200), followed by Vector Elite Kit tertiary antibody complex (Vector) (1:100), and visualized using 3′3′-diaminobenzidine (DAB; Sigma) with a cresyl violet counterstain (Sigma-Aldrich Co.). As prominent expression of Ctr1 and ATP7A proteins have previously been reported in mouse choroid plexus using IHC,19,20 the choroid plexus of a single additional case was used as a positive control for all IHC experiments. Images were taken using a Zeiss AxioCam HRc microscope.
Immunofluorescence
Due to the inherent difficulty to separate positive DAB staining from neuromelanin, IF staining was performed on midbrain tissue sections to examine the cellular localization of Ctr1, ATP7A, and ATP7B in substantia nigra neurons. Formalin-fixed free-floating midbrain sections (50 μm; Ctr1) and formalin-fixed slide-mounted paraffin-embedded sections (7 μm; ATP7A and ATP7B) were antigen retrieved in Tris–EDTA buffer (0.01 M Tris, 1 mM EDTA, pH 9.0) at 95 °C for 25 min (Ctr1), or citrate buffer (pH 6.0) with microwave irradiation for 15 min (ATP7A and ATP7B), and permeabilized with 50% ethanol. Non-specific antigen sites were blocked in 10% NHS (Australis; Ctr1), or 0.25% Casein (Sigma; ATP7A and ATP7B) for 1 hour at room temperature and midbrain sections double-stained for the dopaminergic cell marker tyrosine hydroxylase (TH; 1:1000) and for Ctr1 (1:25), ATP7A (1:100), or ATP7B (1:400). Primary antibodies were detected using secondary IgG antibodies conjugated to Alexa Fluor® 594 or Alexa Fluor® 488 fluorophores (Invitrogen), respectively and images were taken under high magnification, using a Nikon D-Eclipse C1 Si scanning confocal microscope.
Statistical analyses
Statistical analysis was performed using PASW v18 (SPSS inc.). Univariate analysis of variance followed by Bonferoni's t-test was used to test for relationships between copper transporter or copper levels and tissue variables including brain region, brain pH, post-mortem delay, and sex. Linear regression was used to test for relationships between copper transporter levels, copper levels, and tissue variables with age. The significance level was set at 0.05.
Results and discussion
Regional metal levels in the human brain
In addition to total copper levels, regional metal levels determined by ICP-MS are shown in Table 3. Analytical figures of merit (see Table S1, ESI†) demonstrate adequate sensitivity for determining metal concentrations in tissue digests. With the exception of selenium, metal levels varied in a regional manner unique for each metal and were unaffected by post-mortem delay, brain pH, or sex. Copper levels in all brain regions analyzed were remarkably similar, varying between 4 and 5 μg of copper per g of wet weight tissue, with the exception of the substantia nigra, which contained copper levels more than two-fold higher than that in all other brain regions analysed (p<0.001 for every brain region; Table 3). Zinc levels were slightly higher than copper levels, as previously reported,21 and were significantly greater in the substantia nigra, compared to the putamen (p=0.04), visual cortex (p=0.026) and anterior cingulate cortex (p=0.038) (Table 3). Consistent with previous reports,9,21 iron was the most abundant metal in all regions and levels were greatest in the substantia nigra, followed by the body of caudate and putamen (Table 3). Iron levels were significantly higher in the substantia nigra and body of caudate compared with the visual cortex (p=0.006, p=0.029), anterior cingulate cortex (p=0.001, p=0.006), and the cerebellum (p<0.001, p=0.002), respectively. These data suggests an increased requirement for, or storage of copper, zinc, and iron in the substantia nigra. This brain region, associated with high levels of oxidative stress, may require high levels of copper and zinc for the function of Cu/Zn superoxide dismutase, a copper- and zinc-dependent enzyme critical for antioxidant defense.22 Iron is required for TH activity, the rate-limiting step in dopamine synthesis.23 Further, some studies suggest that these metals are also required for neuromelanin synthesis.24–27 Manganese and selenium levels were markedly lower than copper, zinc, and iron levels and, while manganese levels were greatest in the body of caudate and lowest in the anterior cingulate cortex (p=0.028), selenium levels did not differ between brain regions (Table 3). Copper, iron and zinc levels did not significantly change with age, but levels of manganese and selenium significantly increased with age (p=0.043, and p=0.003, respectively). Selenium accumulated uniformly in all brain regions, whereas the age-related increase in manganese primarily resulted from an increase in the body of caudate (p=0.031).
Table 3 Regional differences in metal levels, determined by ICP-MS
Brain region |
n
|
Metal ion levels (μg g−1 wet tissue weight) |
Copper |
Iron |
Zinc |
Manganese |
Selenium |
Values are mean ± SEM. Brain regions where metal levels were significantly greater than other regions are marked with an asterisk. Levels of copper in the substantia nigra were greater than that in all other brain regions (p<0.001). Iron levels were higher in the substantia nigra and body of caudate compared with the visual cortex (p=0.006, p=0.029), anterior cingulate cortex (p=0.001, p=0.006), and cerebellum (p<0.001, p=0.002), respectively. Levels of zinc in the substantia nigra were greater than that in the putamen (p=0.04), visual cortex (p=0.026), and anterior cingulate cortex (p=0.038). Levels of manganese in the body of caudate were greater than that in the anterior cingulate cortex (p=0.028). |
Visual cortex |
10 |
4.14 ± 0.34 |
51.61 ± 3.19 |
11.01 ± 0.88 |
0.15 ± 0.04 |
0.14 ± 0.03 |
Anterior cingulate cortex |
10 |
4.04 ± 0.27 |
42.51 ± 2.61 |
11.24 ± 0.67 |
0.09 ± 0.04 |
0.09 ± 0.03 |
Body of caudate |
10 |
5.09 ± 0.70 |
108.01 ± 20.60* |
12.44 ± 0.94 |
0.31 ± 0.06* |
0.07 ± 0.03 |
Putamen |
10 |
4.47 ± 0.47 |
78.15 ± 16.85 |
11.26 ± 0.50 |
0.22 ± 0.05 |
0.12 ± 0.05 |
Substantia nigra |
5 |
11.40 ± 2.50* |
131.97 ± 15.57* |
16.81 ± 3.56* |
0.20 ± 0.05 |
0.07 ± 0.04 |
Cerebellum |
9 |
4.85 ± 0.57 |
33.23 ± 3.71 |
11.84 ± 0.58 |
0.19 ± 0.05 |
0.18 ± 0.05 |
Copper transporter protein levels
Western blotting detected Ctr1 (28 kDa), Atox1 (7 kDa), ATP7A (173 kDa), and ATP7B (165 kDa) in all brain regions (Fig. 1). Regional levels of Ctr1, Atox1 and ATP7B did not differ, but levels of ATP7A in the cerebellum were significantly greater than that in all other brain regions investigated (p=0.004), with the exception of the substantia nigra (Table 4). Regression analyses identified significant relationships between both Ctr1 and Atox1 protein levels and brain copper levels (p=0.035 and p=0.002, respectively; Fig. 2). There was no relationship between ATP7A, or ATP7B levels and brain copper levels, nor were levels of any of the copper transporter proteins associated with each other or with age.
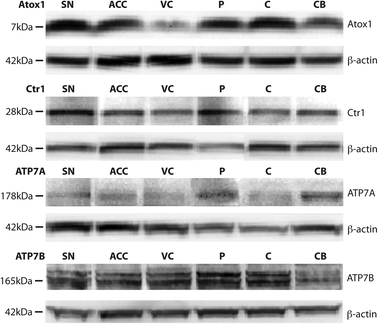 |
| Fig. 1 Representative Western blots demonstrating the presence of the major copper transport proteins Copper transporter 1 (Ctr1), Atox1, ATP7A, and ATP7B in multiple regions in the human brain, including the substantia nigra (SN), anterior cingulate cortex (ACC), visual cortex (VC), putamen (P), body of caudate (C), and cerebellum (CB). β-actin was used as a protein loading control. | |
Table 4 Regional differences in copper transporter levels, determined by Western blot
Brain region |
n
|
Copper transporter levels (Relative to control) |
Ctr1 |
Atox1 |
ATP7A |
ATP7B |
Copper transporter protein levels were semi-quantified by densitometry analysis of Western blot bands. Copper transporter levels were normalized to β-actin levels and expressed relative to an internal standard control. Values are mean ± SEM. Levels of ATP7A in the cerebellum were greater than that in all other brain regions (p<0.001)*. |
Visual cortex |
10 |
0.65 ± 0.07 |
1.08 ± 0.21 |
0.67 ± 0.12 |
0.58 ± 0.19 |
Anterior cingulate cortex |
10 |
0.68 ± 0.09 |
1.34 ± 0.25 |
0.88 ± 0.18 |
0.47 ± 0.19 |
Body of caudate |
10 |
0.74 ± 0.08 |
1.26 ± 0.20 |
0.72 ± 0.15 |
0.84 ± 0.32 |
Putamen |
10 |
0.71 ± 0.12 |
1.39 ± 0.22 |
0.70 ± 0.28 |
0.61 ± 0.20 |
Substantia nigra |
5 |
0.73 ± 0.16 |
2.05 ± 0.60 |
1.00 ± 0.22 |
0.33 ± 0.15 |
Cerebellum |
10 |
0.68 ± 0.07 |
0.92 ± 0.12 |
2.00 ± 0.45* |
0.78 ± 0.27 |
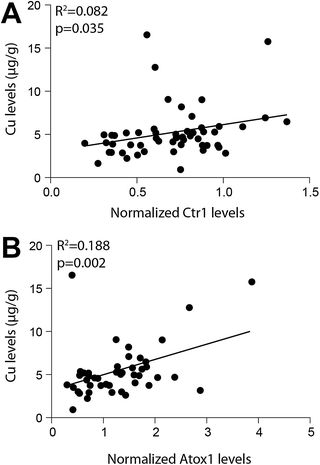 |
| Fig. 2 Regression analyses demonstrated a significant association between both normalized Copper transporter 1 (Ctr1) levels and Atox1 levels with copper (Cu) levels in the human brain. Copper transporter levels were quantified by densitometry performed on Western blots and normalized to β-actin levels (used as a loading control). Cu levels were determined by inductively coupled plasma-mass spectrometry. | |
Copper transporter cellular localization
IHC of copper transporter proteins demonstrated expression of Ctr1, ATP7A, and ATP7B in all brain regions in distinct cell populations (Fig. 3). Although we detected quantifiable amounts of Atox1 by Western blot, consistent with studies in human cell lines and liver,28 this protein was not able to be visualized using IHC on fixed paraffin-embedded tissue. There is only one report, where Atox1 protein has been detected by IHC, using an in-house Atox1 antibody and minimal fixation.29 Atox1 is a small soluble cytoplasmic, 7 kDa protein, and is not membrane bound. Therefore, it is likely that this soluble protein is lost during tissue fixation where soluble solutions are exchanged between internal and external tissue compartments. Many small water-soluble transmitters, for example, are not visible using IHC in fixed tissues.30–32
 |
| Fig. 3 The cellular localization of copper transport proteins Copper transporter 1 (Ctr1), ATP7A, and ATP7B in the human brain. 7 μm paraffin embedded human brain sections were probed for copper transporters, Ctr1, ATP7A, and ATP7B using peroxidase immunohistochemistry (brown) with cresyl violet counterstain. Regions of positive staining are dark brown. Scale bar in L applies to A-L. Scale bar in O applies to M-O. Insets show localization of copper transport proteins in the choroid plexus and caudate putamen at higher magnification. Arrows in A-C insets highlight more concentrated positive staining on the apical (A and C) and basolateral (B) membranes. | |
Intense cytoplasmic staining for Ctr1 protein, particularly concentrated to the apical surface, was observed in the polarized epithelial cells of the choroid plexus (Fig. 3A). Apical and basolateral membranes of the choroid plexus were determined based on morphology, as described in previous studies.33–36 Ctr1 staining in the visual cortex, anterior cingulate cortex, and caudate and putamen (Fig. 3D, G and M), however, revealed a punctate distribution in the cytoplasm, which differed to that seen in the choroid plexus. While Ctr1 staining in the visual cortex, anterior cingulate cortex, and caudate and putamen was primarily neuronal (Fig. 3D, G and M), in the cerebellum there was a striking lack of expression of Ctr1 in Purkinje neurons (Fig. 3J). Rather, Ctr1 expression was restricted to Bergmann glia (Fig. 3J). In the anterior cingulate cortex, Ctr1 staining of the proximal fibers was very weak compared to staining in neuronal cell bodies (Fig. 3G). Ctr1 staining of the striosomes in the caudate and putamen was also very weak (Fig. 3M). Substantia nigra pars compacta (SNpc) dopaminergic neurons exhibited robust Ctr1 staining in a circular pattern around the neuromelanin granules (Fig. 4A). The non-pigmented substantia nigra pars reticulata (SNpr), was predominantly negative for Ctr1.
 |
| Fig. 4 Representative high magnification photomicrographs of the cellular location of Copper transporter 1 (Ctr1), ATP7A, and ATP7B in the human substantia nigra pars compacta. Midbrain sections dual stained for the dopaminergic marker, tyrosine hydroxylase (green), and Ctr1 (A), ATP7A (B), or ATP7B (C) (red). Yellow regions represent co-localisation of TH (green) with Ctr1, ATP7A, or ATP7B (red). Insets: No Ctr1 primary control (A), and no ATP7A and ATP7B primary control (C). Arrows highlight regions of positive staining. | |
Cytoplasmic and apical membrane staining for ATP7A was observed in the polarized epithelial cells of the choroid plexus, but was most concentrated at the basolateral surface (Fig. 3B). In contrast, ATP7B protein was expressed predominantly in the membrane of these epithelial cells and was most concentrated on the apical surface (Fig. 3C). In all other brain regions investigated, the staining pattern for ATP7A was very similar to ATP7B. ATP7A and ATP7B staining in the visual cortex and anterior cingulate cortex (Fig. 3E, F, H and I) was localized to the cytoplasm. Unlike Ctr1 staining in these brain regions, ATP7A and ATP7B staining was not punctate. Most notably, while Ctr1 was strongly expressed only in neuronal cell bodies in the anterior cingulate cortex, ATP7A and ATP7B were strongly expressed in both neuronal cell bodies and proximal fibers in this brain region (Fig. 3H and I), and, unlike Ctr1, ATP7A and ATP7B, staining was clearly positive in the striosomes of the caudate and putamen (Fig. 3N and O). Furthermore, strong staining for the ATPases was observed in cerebellar Purkinje neurons, and not in Bergmann glia (Fig. 3K and L). In contrast to Ctr1, staining for ATP7A was not specifically localized to neuromelanin in the SNpc, but was most strongly expressed in the cytoplasm (Fig. 4B). ATP7B was present in the cytoplasm and associated with neuromelanin (Fig. 4C). Both ATP7A and ATP7B were not present in the nucleus or axons (Fig. 4B and C).
Ctr1,19 ATP7A,20,37,38 and ATP7B37,38 protein and mRNA39 have been previously reported in rodent brain, while Atox1 protein has been identified only in human cell lines and human liver.28 Here we report the presence of the copper transporter proteins Ctr1, Atox1, ATP7A, and ATP7B in the human brain.
Few studies have analysed regional variations in copper levels in the normal human brain.9,21,40,41 Consistent with previous reports,9,40,42 copper levels were highest in the human substantia nigra, suggesting an increased requirement for, or storage of, copper in this brain region.
We demonstrate here that regional levels of the major copper transport protein Ctr1 are associated with regional differences in copper levels. While descriptive in nature, these data are consistent with data from experimental animal models reporting elevated brain Ctr1 protein levels in Cu deficient mice,19,43 and support a role for this protein in regulating copper levels in the human brain. Western blot analyses demonstrated that the primary form of Ctr1 in the human brain is the monomeric (28 kDa) protein,44,45 previously reported in mouse choroid plexus.43
Ctr1 was observed to have a cytoplasmic, vesicular distribution, in agreement with findings in human placental tissue46 and a number of human carcinoma cell lines.45 It has been hypothesized that Ctr1 can exist as both intracellular and plasma membrane pools of protein in a state of pseudo-equilibrium, which change in response to variations in extracellular copper levels.47,48 Our data suggest that, in the normal human brain with adequate cellular copper, Ctr1 exists primarily as an internalized protein pool, rather than as an active membrane-bound transporter. This is consistent with in vitro data from HEK cells over-expressing human Ctr1 which showed that the protein is internalized from the plasma membrane in response to extracellular copper levels within or above the normal physiological range, but recycled to the plasma membrane when extracellular copper levels are depleted.47
While Ctr1 was localized to the cytoplasm in non-pigmented midbrain neurons, in pigmented neurons Ctr1 was associated with neuromelanin granules (Fig. 4A) and may regulate the acquisition of copper by this pigment.49 In support of this hypothesis, Drosophila expressing a mutant form of the high affinity copper transporter protein, Ctr1B, exhibit decreased larval copper accumulation and body pigmentation defects.50
Like Ctr1, levels of Atox1 protein were associated with regional copper levels, suggesting this protein also plays a role in regulating brain copper. These findings are in agreement with a previous report of Atox1 mRNA in human brain51 and the expression pattern of Atox1 mRNA in the rat brain, where the highest levels of Atox1 mRNA are found in neuronal populations that sequester copper.52
With the exception of the polarized epithelial cells of the choroid plexus, ATP7A and ATP7B were predominantly localized to the cytoplasm, consistent with their proposed localization to the trans-Golgi network.53–55 In addition to neuronal cytoplasmic staining, strong expression of ATP7A and ATP7B proteins, but not of Ctr1, was observed in the cerebellum, anterior cingulate cortex, and caudate putamen in the proximal fibers. A role for ATP7A in proximal fiber health is supported by studies in mouse models of Menkes’ disease, a lethal infantile neurodegenerative disorder of copper metabolism caused by mutations in ATP7A. In these animals, axons in the cerebral cortex, cerebellum, and hippocampus remain undeveloped, and cortical dendrites are swollen,38,56 but this pathology is avoided by ATP7A gene therapy at the choroid plexus, plus copper treatment.56 Interestingly, ATP7A and ATP7B were not expressed in the axons of dopaminergic neurons in the substantia nigra, suggesting that in the normal adult brain these axons do not actively require ATP7A expression. This concept of a stage-specific role for axonal ATP7A is supported by studies in the mouse brain where ATP7A protein expression in the dendrites of Purkinje neurons was demonstrated to increase into adulthood, but was lost in the optic nerve axons of postnatal mice.20
Interestingly, neither ATP7A nor ATP7B protein levels were significantly associated with brain copper levels. This finding is in agreement with previous studies demonstrating that changes in copper concentration have no effect on steady state protein levels of ATP7A in vitro53 or ATP7B in vivo,54 but that copper levels influenced the subcellular localization of these proteins.53,54 These data suggest that, in the adult human brain, changes in copper levels may be regulated by changes in the cellular localization of the ATPase proteins, rather than changes in their level of expression.
While Ctr1, ATP7A and ATP7B proteins were observed to be localized to the cytoplasm in most brain regions, the polarized epithelial cells of the choroid plexus exhibited unique patterns of staining, suggesting that copper is transported in the choroid plexus in a way that is distinct from other brain regions. ATP7A was localized to the cytoplasm and the cell membrane, where unlike ATP7B, it was noticeably present at the basolateral surface, consistent with its localization in polarized murine intestinal epithelial cells57 and kidney.58 ATP7B was predominantly localized to the cell membrane and was particularly concentrated at the apical surface, consistent with its localization in human breast epithelial cells59 and in agreement with in vitro data from polarized hepatocytes.60 Although Ctr1 was predominantly cytoplasmic, it too was more concentrated at the apical surface. The observed localization for ATP7A and ATP7B suggests a role for ATP7A in mediating copper efflux across the basolateral membrane into capillaries, and suggests that ATP7B may mediate the transfer of copper into the CSF. This transfer may occur via a mechanism similar to that seen in mammary epithelial cells, where ATP7A is proposed to play a role in removing excess copper, while ATP7B transports copper across the apical surface and is thought to be the major means of copper secretion into milk during lactation.61
In selected brain regions the ATPases and Ctr1 were expressed in different cell types. In the cerebellum, for example, Ctr1 was strongly expressed in Bergmann glia but not detected in Purkinje neurons, whereas the ATPases demonstrated an inverse pattern of expression. Cell-type specific expression has previously been demonstrated in rat dorsal root ganglion62 and human placental tissue,46 where cells that strongly expressed ATP7A, expressed lower levels of Ctr1. Increased Ctr1 expression in mammary tissue during pregnancy and lactation,19 and in duodenal and brain tissue from copper deficient mice,19 suggest that Ctr1 expression may depend on the immediate copper requirement of the cell. Further, changes in the intensity of Ctr1 staining and the cell-type specific expression of the ATPases have been demonstrated in mouse brain tissue at different stages of development.19,37 It has been suggested that cells which strongly express Ctr1 have a high requirement for copper potentially to meet cuproenzyme requirements,63–65 while cells with low Ctr1 expression and high ATP7A may be sensitive to copper and require a high copper efflux capacity.62 ATP7A expression was greatest in the cerebellum where staining was localised to the Purkinje neurons, a finding also reported in the mouse brain.20 Higher levels of ATP7A may be required in the cerebellum to maintain normal function, as the cerebellar Purkinje neurons are particularly sensitive to copper changes in Menkes’ disease.66–68
Conclusions
In this first comprehensive study of copper transporter proteins in the human brain, we have described the presence of Ctr1, Atox1, ATP7A, and ATP7B in multiple human brain regions and identified significant relationships between brain copper and copper transporter protein levels. An understanding of cellular copper transport in the human brain will be critical to understand the mechanisms of disrupted brain copper in multiple disorders where brain copper levels are altered.
Disclosure
The authors report no conflicts of interest.
Acknowledgements
We wish to thank Vanessa Krapp for technical assistance and Heidi Cartwright for figure preparation. Tissues were received from the New South Wales Tissue Resource Centre at the University of Sydney, supported by the National Health and Medical Research Council of Australia, Schizophrenia Research Institute and the National Institute of Alcohol Abuse and Alcoholism (NIH (NIAAA) R24AA012725), and from the Sydney Brain Bank, which is supported by Neuroscience Research Australia, the University of New South Wales and the National Health and Medical Research Council of Australia. This work was supported by a project grant from the National Health and Medical Research Council of Australia (to K.L.D and J.F.B.M), and a seed grant from Parkinson's New South Wales (to K.L.D). KLD and GMH were recipients of Research Fellowships from the National Health and Medical Research Council of Australia, and DH was a recipient of a Research Fellowship from the Australian Research Council.
References
- G. J. Brewer, The risks of free copper in the body and the development of useful anticopper drugs, Curr. Opin. Clin. Nutr. Metab. Care, 2008, 11, 727–732 CrossRef CAS.
- J. F. Mercer, J. Livingston, B. Hall, J. A. Paynter, C. Begy, S. Chandrasekharappa, P. Lockhart, A. Grimes, M. Bhave and D. Siemieniak,
et al., Isolation of a partial candidate gene for Menkes disease by positional cloning, Nat. Genet., 1993, 3, 20–25 CrossRef CAS.
- N. Gouider-Khouja, Wilson's disease, Parkinsonism Relat. Disord., 2009, 15(Suppl 3), S126–S129 CrossRef.
- M. W. Bourassa and L. M. Miller, Metal imaging in neurodegenerative diseases, Metallomics, 2012, 4, 721–738 RSC.
- M. A. Deibel, W. D. Ehmann and W. R. Markesbery, Copper, iron, and zinc imbalances in severely degenerated brain regions in Alzheimer's disease: possible relation to oxidative stress, J. Neurol. Sci., 1996, 143, 137–142 CrossRef CAS.
- M. A. Lovell, J. D. Robertson, W. J. Teesdale, J. L. Campbell and W. R. Markesbery, Copper, iron and zinc in Alzheimer's disease senile plaques, J. Neurol. Sci., 1998, 158, 47–52 CrossRef CAS.
- H. M. Kurlander and B. M. Patten, Metals in spinal cord tissue of patients dying of motor neuron disease, Ann. Neurol., 1979, 6, 21–24 CrossRef CAS.
- B. Tomik, J. Chwiej, M. Szczerbowska-Boruchowska, M. Lankosz, S. Wojcik, D. Adamek, G. Falkenberg, S. Bohic, A. Simionovici, Z. Stegowski and A. Szczudlik, Implementation of X-ray fluorescence microscopy for investigation of elemental abnormalities in amyotrophic lateral sclerosis, Neurochem. Res., 2006, 31, 321–331 CrossRef CAS.
- D. T. Dexter, F. R. Wells, A. J. Lees, F. Agid, Y. Agid, P. Jenner and C. D. Marsden, Increased nigral iron content and alterations in other metal ions occurring in brain in Parkinson's disease, J. Neurochem., 1989, 52, 1830–1836 CrossRef CAS.
- D. A. Loeffler, P. A. LeWitt, P. L. Juneau, A. A. Sima, H. U. Nguyen, A. J. DeMaggio, C. M. Brickman, G. J. Brewer, R. D. Dick, M. D. Troyer and L. Kanaley, Increased regional brain concentrations of ceruloplasmin in neurodegenerative disorders, Brain Res., 1996, 738, 265–274 CrossRef CAS.
- B. S. Wong, S. G. Chen, M. Colucci, Z. Xie, T. Pan, T. Liu, R. Li, P. Gambetti, M. S. Sy and D. R. Brown, Aberrant metal binding by prion protein in human prion disease, J. Neurochem., 2001, 78, 1400–1408 CrossRef CAS.
- W. S. Eum and J. H. Kang, Release of copper ions from the familial amyotrophic lateral sclerosis-associated Cu, Zn-superoxide dismutase mutants, Mol. Cells, 1999, 9, 110–114 CAS.
- X. Huang, M. P. Cuajungco, C. S. Atwood, M. A. Hartshorn, J. D. Tyndall, G. R. Hanson, K. C. Stokes, M. Leopold, G. Multhaup, L. E. Goldstein, R. C. Scarpa, A. J. Saunders, J. Lim, R. D. Moir, C. Glabe, E. F. Bowden, C. L. Masters, D. P. Fairlie, R. E. Tanzi and A. I. Bush, Cu(II) potentiation of alzheimer Aβ neurotoxicity. Correlation with cell-free hydrogen peroxide production and metal reduction, J. Biol. Chem., 1999, 274, 37111–37116 CrossRef CAS.
- X. Wang, D. Moualla, J. A. Wright and D. R. Brown, Copper binding regulates intracellular alpha-synuclein localisation, aggregation and toxicity, J. Neurochem., 2010, 113, 704–714 CrossRef CAS.
- L. Rossi, E. Marchese, M. F. Lombardo, G. Rotilio and M. R. Ciriolo, Increased susceptibility of copper-deficient neuroblastoma cells to oxidative stress-mediated apoptosis, Free Radicals Biol. Med., 2001, 30, 1177–1187 CrossRef CAS.
- J. R. Prohaska and T. L. Smith, Effect of dietary or genetic copper deficiency on brain catecholamines, trace metals and enzymes in mice and rats, J. Nutr., 1982, 112, 1706–1717 CAS.
- M. A. Cater, S. La Fontaine and J. F. Mercer, Copper binding to the N-terminal metal-binding sites or the CPC motif is not essential for copper-induced trafficking of the human Wilson protein (ATP7B), Biochem. J., 2007, 401, 143–153 CrossRef CAS.
- B. X. Ke, R. M. Llanos, M. Wright, Y. Deal and J. F. Mercer, Alteration of copper physiology in mice overexpressing the human Menkes protein ATP7A, Am. J. Physiol., 2006, 290, R1460–R1467 CAS.
- Y. M. Kuo, A. A. Gybina, J. W. Pyatskowit, J. Gitschier and J. R. Prohaska, Copper transport protein (Ctr1) levels in mice are tissue specific and dependent on copper status, J. Nutr., 2006, 136, 21–26 CAS.
- M. J. Niciu, X. M. Ma, R. El Meskini, G. V. Ronnett, R. E. Mains and B. A. Eipper, Developmental changes in the expression of ATP7A during a critical period in postnatal neurodevelopment, Neuroscience, 2006, 139, 947–964 CrossRef CAS.
- M. T. Rajan, K. S. Jagannatha Rao, B. M. Mamatha, R. V. Rao, P. Shanmugavelu, R. B. Menon and M. V. Pavithran, Quantification of trace elements in normal human brain by inductively coupled plasma atomic emission spectrometry, J. Neurol. Sci., 1997, 146, 153–166 CrossRef CAS.
- H. Saggu, J. Cooksey, D. Dexter, F. R. Wells, A. Lees, P. Jenner and C. D. Marsden, A selective increase in particulate superoxide dismutase activity in parkinsonian substantia nigra, J. Neurochem., 1989, 53, 692–697 CrossRef CAS.
- A. J. Ramsey, P. J. Hillas and P. F. Fitzpatrick, Characterization of the active site iron in tyrosine hydroxylase. Redox states of the iron, J. Biol. Chem., 1996, 271, 24395–24400 CrossRef CAS.
- Y. Izumi, H. Sawada, N. Yamamoto, T. Kume, H. Katsuki, S. Shimohama and A. Akaike, Iron accelerates the conversion of dopamine-oxidized intermediates into melanin and provides protection in SH-SY5Y cells, J. Neurosci. Res., 2005, 82, 126–137 CrossRef CAS.
- D. Sulzer, J. Bogulavsky, K. E. Larsen, G. Behr, E. Karatekin, M. H. Kleinman, N. Turro, D. Krantz, R. H. Edwards, L. A. Greene and L. Zecca, Neuromelanin biosynthesis is driven by excess cytosolic catecholamines not accumulated by synaptic vesicles, Proc. Natl. Acad. Sci. U. S. A., 2000, 97, 11869–11874 CrossRef CAS.
- L. Zecca, R. Pietra, C. Goj, C. Mecacci, D. Radice and E. Sabbioni, Iron and other metals in neuromelanin, substantia nigra, and putamen of human brain, J. Neurochem., 1994, 62, 1097–1101 CrossRef CAS.
- L. Zecca, D. Tampellini, A. Gatti, R. Crippa, M. Eisner, D. Sulzer, S. Ito, R. Fariello and M. Gallorini, The neuromelanin of human substantia nigra and its interaction with metals, J. Neural Transm., 2002, 109, 663–672 CrossRef CAS.
- I. Hamza, M. Schaefer, L. W. Klomp and J. D. Gitlin, Interaction of the copper chaperone HAH1 with the Wilson disease protein is essential for copper homeostasis, Proc. Natl. Acad. Sci. U. S. A., 1999, 96, 13363–13368 CrossRef CAS.
- S. D. Moore, K. E. Helmle, L. M. Prat and D. W. Cox, Tissue localization of the copper chaperone ATOX1 and its potential role in disease, Mamm. Genome, 2002, 13, 563–568 CrossRef CAS.
- B. M. Riederer, R. Porchet, R. A. Marugg and L. I. Binder, Solubility of cytoskeletal proteins in immunohistochemistry and the influence of fixation, J. Histochem. Cytochem., 1993, 41, 609–616 CrossRef CAS.
- M. A. Melan and G. Sluder, Redistribution and differential extraction of soluble proteins in permeabilized cultured cells. Implications for immunofluorescence microscopy, J. Cell Sci., 1992, 101(Pt 4), 731–743 Search PubMed.
- A. Pekki and P. Tuohimaa, New freeze-dry and vapor fixation method for immunohistochemistry of soluble proteins: subcellular location of the progesterone receptor, J. Histochem. Cytochem., 1989, 37, 1207–1213 CrossRef CAS.
- J. Praetorius, L. N. Nejsum and S. Nielsen, A SCL4A10 gene product maps selectively to the basolateral plasma membrane of choroid plexus epithelial cells, Am. J. Physiol., 2004, 286, C601–C610 CrossRef CAS.
- S. L. Alper, A. Stuart-Tilley, C. F. Simmons, D. Brown and D. Drenckhahn, The fodrin-ankyrin cytoskeleton of choroid plexus preferentially colocalizes with apical Na + K(+)-ATPase rather than with basolateral anion exchanger AE2, J. Clin. Invest., 1994, 93, 1430–1438 CrossRef CAS.
- R. H. Angeletti, P. M. Novikoff, S. R. Juvvadi, J. M. Fritschy, P. J. Meier and A. W. Wolkoff, The choroid plexus epithelium is the site of the organic anion transport protein in the brain, Proc. Natl. Acad. Sci. U. S. A., 1997, 94, 283–286 CrossRef CAS.
- P. M. Quinton, E. M. Wright and J. M. Tormey, Localization of sodium pumps in the choroid plexus epithelium, J. Cell Biol., 1973, 58, 724–730 CrossRef CAS.
- N. Barnes, R. Tsivkovskii, N. Tsivkovskaia and S. Lutsenko, The copper-transporting ATPases, Menkes and Wilson disease proteins, have distinct roles in adult and developing cerebellum, J. Biol. Chem., 2005, 280, 9640–9645 CrossRef CAS.
- M. J. Niciu, X. M. Ma, R. El Meskini, J. S. Pachter, R. E. Mains and B. A. Eipper, Altered ATP7A expression and other compensatory responses in a murine model of Menkes disease, Neurobiol. Dis., 2007, 27, 278–291 CrossRef CAS.
- B. S. Choi and W. Zheng, Copper transport to the brain by the blood–brain barrier and blood–CSF barrier, Brain Res., 2009, 1248, 14–21 CrossRef CAS.
- W. J. Goldberg and N. Allen, Determination of Cu, Mn, Fe, and Ca in six regions of normal human brain, by atomic absorption spectroscopy, Clin. Chem., 1981, 27, 562–564 CAS.
- W. W. Harrison, M. G. Netsky and M. D. Brown, Trace elements in human brain: copper, zinc, iron, and magnesium, Clin. Chim. Acta, 1968, 21, 55–60 CrossRef CAS.
- L. Zecca, F. A. Zucca, M. Toscani, F. Adorni, G. Giaveri, E. Rizzio and M. Gallorini, Iron, Copper and their proteins in substantia nigra of human brain during aging, J. Radioanal. Nucl. Chem., 2005, 263, 5 CrossRef.
- A. A. Gybina and J. R. Prohaska, Variable response of selected cuproproteins in rat choroid plexus and cerebellum following perinatal copper deficiency, Genes Nutr., 2006, 1, 51–59 CrossRef CAS.
- J. F. Eisses and J. H. Kaplan, Molecular characterization of hCTR1, the human copper uptake protein, J. Biol. Chem., 2002, 277, 29162–29171 CrossRef CAS.
- A. E. Klomp, B. B. Tops, I. E. Van Denberg, R. Berger and L. W. Klomp, Biochemical characterization and subcellular localization of human copper transporter 1 (hCTR1), Biochem. J., 2002, 364, 497–505 CrossRef CAS.
- B. Hardman, U. Manuelpillai, E. M. Wallace, J. F. Monty, D. R. Kramer, Y. M. Kuo, J. F. Mercer and M. L. Ackland, Expression, localisation and hormone regulation of the human copper transporter hCTR1 in placenta and choriocarcinoma Jeg-3 cells, Placenta, 2006, 27, 968–977 CrossRef CAS.
- S. A. Molloy and J. H. Kaplan, Copper-dependent recycling of hCTR1, the human high affinity copper transporter, J. Biol. Chem., 2009, 284, 29704–29713 CrossRef CAS.
- M. J. Petris, K. Smith, J. Lee and D. J. Thiele, Copper-stimulated endocytosis and degradation of the human copper transporter, hCtr1, J. Biol. Chem., 2003, 278, 9639–9646 CrossRef CAS.
- S. Bohic, K. Murphy, W. Paulus, P. Cloetens, M. Salome, J. Susini and K. Double, Intracellular chemical imaging of the developmental phases of human neuromelanin using synchrotron X-ray microspectroscopy, Anal. Chem., 2008, 80, 9557–9566 CrossRef CAS.
- H. Zhou, K. M. Cadigan and D. J. Thiele, A copper-regulated transporter required for copper acquisition, pigmentation, and specific stages of development in Drosophila melanogaster, J. Biol. Chem., 2003, 278, 48210–48218 CrossRef CAS.
- L. W. Klomp, S. J. Lin, D. S. Yuan, R. D. Klausner, V. C. Culotta and J. D. Gitlin, Identification and functional expression of HAH1, a novel human gene involved in copper homeostasis, J. Biol. Chem., 1997, 272, 9221–9226 CrossRef CAS.
- G. S. Naeve, A. M. Vana, J. R. Eggold, G. S. Kelner, R. Maki, E. B. Desouza and A. C. Foster, Expression profile of the copper homeostasis gene, rAtox1, in the rat brain, Neuroscience, 1999, 93, 1179–1187 CrossRef CAS.
- M. J. Petris, J. F. Mercer, J. G. Culvenor, P. Lockhart, P. A. Gleeson and J. Camakaris, Ligand-regulated transport of the Menkes copper P-type ATPase efflux pump from the Golgi apparatus to the plasma membrane: a novel mechanism of regulated trafficking, EMBO J., 1996, 15, 6084–6095 CAS.
- M. Schaefer, R. G. Hopkins, M. L. Failla and J. D. Gitlin, Hepatocyte-specific localization and copper-dependent trafficking of the Wilson's disease protein in the liver, Am. J. Physiol., 1999, 276, G639–G646 CAS.
- T. Yamaguchi, A. Yamamoto, A. Furuno, K. Hatsuzawa, K. Tani, M. Himeno and M. Tagaya, Possible involvement of heterotrimeric G proteins in the organization of the Golgi apparatus, J. Biol. Chem., 1997, 272, 25260–25266 CrossRef CAS.
- A. Donsante, L. Yi, P. M. Zerfas, L. R. Brinster, P. Sullivan, D. S. Goldstein, J. Prohaska, J. A. Centeno, E. Rushing and S. G. Kaler, ATP7A gene addition to the choroid plexus results in long-term rescue of the lethal copper transport defect in a Menkes disease mouse model, Mol. Ther., 2011, 19, 2114–2123 CrossRef CAS.
- J. F. Monty, R. M. Llanos, J. F. Mercer and D. R. Kramer, Copper exposure induces trafficking of the menkes protein in intestinal epithelium of ATP7A transgenic mice, J. Nutr., 2005, 135, 2762–2766 CAS.
- A. Grimes, C. J. Hearn, P. Lockhart, D. F. Newgreen and J. F. Mercer, Molecular basis of the brindled mouse mutant (Mo(br)): a murine model of Menkes disease, Hum. Mol. Genet., 1997, 6, 1037–1042 CrossRef CAS.
- A. Michalczyk, E. Bastow, M. Greenough, J. Camakaris, D. Freestone, P. Taylor, M. Linder, J. Mercer and M. L. Ackland, ATP7B expression in human breast epithelial cells is mediated by lactational hormones, J. Histochem. Cytochem., 2008, 56, 389–399 CrossRef CAS.
- H. Roelofsen, H. Wolters, M. J. Van Luyn, N. Miura, F. Kuipers and R. J. Vonk, Copper-induced apical trafficking of ATP7B in polarized hepatoma cells provides a mechanism for biliary copper excretion, Gastroenterology, 2000, 119, 782–793 CrossRef CAS.
- R. M. Llanos, A. A. Michalczyk, D. J. Freestone, S. Currie, M. C. Linder, M. L. Ackland and J. F. Mercer, Copper transport during lactation in transgenic mice expressing the human ATP7A protein, Biochem. Biophys. Res. Commun., 2008, 372, 613–617 CrossRef CAS.
- V. Ip, J. J. Liu, J. F. Mercer and M. J. McKeage, Differential expression of ATP7A, ATP7B and CTR1 in adult rat dorsal root ganglion tissue, Mol. Pain, 2010, 6, 53 CrossRef.
- J. Lee, M. M. Pena, Y. Nose and D. J. Thiele, Biochemical characterization of the human copper transporter Ctr1, J. Biol. Chem., 2002, 277, 4380–4387 CrossRef CAS.
- J. Lee, J. R. Prohaska and D. J. Thiele, Essential role for mammalian copper transporter Ctr1 in copper homeostasis and embryonic development, Proc. Natl. Acad. Sci. U. S. A., 2001, 98, 6842–6847 CrossRef CAS.
- H. Kim, H. Y. Son, S. M. Bailey and J. Lee, Deletion of hepatic Ctr1 reveals its function in copper acquisition and compensatory mechanisms for copper homeostasis, Am. J. Physiol., 2009, 296, G356–G364 CrossRef CAS.
- J. H. Menkes, M. Alter, G. K. Steigleder, D. R. Weakley and J. H. Sung, A sex-linked recessive disorder with retardation of growth, peculiar hair, and focal cerebral and cerebellar degeneration, Pediatrics, 1962, 29, 764–779 CAS.
- D. Troost, A. van Rossum, W. Straks and J. Willemse, Menkes' kinky hair disease. II. A clinicopathological report of three cases, Brain Dev., 1982, 4, 115–126 CrossRef CAS.
- P. C. Liu, Y. W. Chen, J. A. Centeno, M. Quezado, K. Lem and S. G. Kaler, Downregulation of myelination, energy, and translational genes in Menkes disease brain, Mol. Genet. Metab., 2005, 85, 291–300 CrossRef CAS.
Footnote |
† Electronic supplementary information (ESI) available. See DOI: 10.1039/c2mt20151h |
|
This journal is © The Royal Society of Chemistry 2013 |
Click here to see how this site uses Cookies. View our privacy policy here.