Honeycomb-shaped surface topography induces differentiation of human mesenchymal stem cells (hMSCs): uniform porous polymer scaffolds prepared by the breath figure technique†‡
Received
19th August 2013
, Accepted 25th September 2013
First published on 14th October 2013
Abstract
Polystyrene honeycomb scaffolds with different pore sizes were successfully fabricated by casting a polymer solution under humid conditions in order to investigate the effect of porous microtopography on hMSC differentiation. We have used honeycomb scaffolds to achieve the microtopography-induced differentiation of hMSCs. Honeycomb scaffolds led hMSCs to osteospecific and myospecific differentiations depending on the size of pores. This selective differentiation suggested that surface microtopography alone may be effective for using hMSCs in regenerative medicine and tissue engineering.
Introduction
Cell behaviors, including cellular adhesion, proliferation, migration, and differentiation, are regulated by the interactions between cells and their microenvironments. Therefore, the chemical, mechanical, and topological properties of scaffold surfaces are important for regulating the cell behavior.1–4 Recently, there have been some reports that surface softness strongly affects cell differentiation.5,6 The recent development of microfabrication technologies allows the creation of two- or three-dimensional microtopographic features, such as grooves, pits, posts, and pores from a submicrometer scale to a scale of tens of micrometers, in order to model the in vivo topographic microenvironments of cells.7–10 The adhesion, spreading, alignment, and migration of differentiated cells can be promoted or restricted depending on the height, width, and spacing of these micrometer scale topographic features. This phenomenon is known as contact guidance.11–13
It is vital to design the chemical,14 mechanical,15 and topographical properties16 of next-generation tissue engineering scaffolds to make them suitable for stem cells, and to tailor the proliferation, functionalization, and differentiation of induced pluripotent stem cells. The topographical properties of scaffolds have attracted intense interest, because of their great potential for controlling stem cells without hazardous chemicals and biomolecules. Preliminary studies of stem cells cultured on artificial scaffolds with microtopographic features have been conducted using non-woven fiber matrices prepared from conventional textiles or electrospun fibers.17 The width and alignment of the fibers affected the proliferation and shapes of the stem cells, which are related to the functionalization and differentiation of stem cells. To investigate the topological effect further, cellular- and subcellular-scale line-and-space patterns have been used for culturing stem cells.18 Human mesenchymal stem cells (hMSCs) are multipotent stem cells19,20 which can differentiate into stromal lineages, including adipogenic (fat), chondrogenic (cartilage), fibroblastic (connective tissue), osteoblastic (bone), and myoblastic (muscle) cell lineages, and also show myogenic markers. Other cellular-scale scaffolds have been fabricated by photolithography,21 laser microfabrication,22 and colloidal templating.23 Recently, Dalby et al. have reported that subcellular scale porous substrates can control the differentiation of hMSCs with the symmetry of the pore arrangement. However, little is known about the size effects of cellular- or subcellular-scale porous microtopographic features on the proliferation, functionalization, and differentiation of stem cells.
We have previously reported that honeycomb-patterned porous polymer films (honeycomb films) can be prepared by casting a polymer solution under humid conditions.24 Microporous films with hexagonally arranged pores ranging from the submicrometer to the micrometer scale, which are respectively the same as the cellular and subcellular scales, can be prepared using this technique.25,26 Honeycomb films have a unique double-layered structure with spherical pores formed by the template water droplets. Due to their simple preparation process and unique microstructures, many studies about the formation and applications of honeycomb films have been reported.27–29 Additionally, a wide variety of polymers, including non-cytotoxic and biodegradable polymers, can be used. We have already cultured fibroblasts, endothelial cells, and cardiac myocytes on our honeycomb scaffolds, and investigated the effects of the microtopography and elasticity of the scaffolds on the cell functionality and proliferation.30–32
In this study, we fabricated honeycomb scaffolds with different pore and frame sizes by casting a polymer solution under humid conditions in order to investigate the effect of porous microtopography on hMSC differentiation. The cell morphology (cell area and elongation) and the actin cytoskeletons on honeycomb scaffolds were quantified and the expressions of the myoblast phenotype marker, MyoD1, and the osteoblast phenotype marker, osteopontin, were also observed.
Experimental
Preparation of polystyrene honeycomb scaffolds
Polystyrene (PS; Mw = 280 kg mol−1) was purchased from Aldrich. The amphiphilic copolymer, poly(N-dodecyl acrylamide-co-6-acrylamidehexanoic acid) (polymer 1, Fig. 1a), was synthesized according to the literature (Mw = 84 kg mol−1, Mw/Mn = 4.94).32 Dioleoylphosphatidylethanolamine (DOPE) and chloroform were purchased from Wako Chemical Industries Ltd (Japan). PS (180 mg) and polymer 1 (18 mg) or DOPE (1.8 mg) were dissolved in chloroform (40 mL). The chloroform solution was cast under a flow of humid air (10 L min−1) to obtain PS honeycomb scaffolds. As shown in a previous report,33 pore sizes can be controlled by changing the water condensation time. In this case, we changed the solvent evaporation time, which was almost equal to the water condensation time. The surface structures of the PS honeycomb scaffolds were observed by using a field emission-scanning electron microscope (FE-SEM; S-5200, Hitachi, Japan) after the samples were prepared by osmium sputtering.
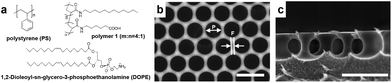 |
| Fig. 1 Structures of the chemicals used (a), SEM (b), and cross-sectional SEM images of a PS honeycomb scaffold (c). (Bar: 10 μm.) | |
Cell culture
The honeycomb substrates were washed in 1-propanol and ethanol to remove polymer 1 and DOPE, respectively. The substrates were immersed in MEM alpha (Gibco BRL, Grand Island, NY) supplemented with 10% fetal bovine serum (FBS; Gibco BRL) overnight. The hMSCs were purchased from Lonza Corporation (Basel, Switzerland). The cells were seeded at a density of 1 × 104 cells per cm2 on the honeycomb substrates, and cultured with MEM alpha containing 10% FBS for 24 h under 5% CO2 in a humidified incubator at 37 °C. All the experiments with the hMSCs were conducted with cultures at passages 4–6.
Analysis
The cell morphology and differentiation were characterized using a fluorescence microscope (FSX 100, Olympus, Japan). The cells were fixed with 4% paraformaldehyde (Wako) for 20 min, blocked in 10% goat serum (Invitrogen, Carlsbad, CA), and permeabilized with 0.1% Triton-X 100 (MP Biomedicals, Irvine, CA) at room temperature for 45 min. The cells were incubated with anti-MyoD1 antibody (Abcam, Cambridge, UK) and anti-osteopontin (OPN) antibody (Santa Cruz Biotechnology Inc., Dallas, TX) for 1 h, and then with Alexa488-anti-mouse IgG (Invitrogen) for 1 h at 37 °C. To visualize the cell morphology, the actin filaments were labeled with rhodamine-phalloidin (Cytoskeleton Inc., Denver, CO). The samples were mounted on glass slides and observed with a 40× objective lens (NA = 0.95). The microscopic images of cell morphology visualized with fluorescent phalloidin were quantitatively analyzed using ImageJ software (National Institute of Health, Bethesda, MD). ImageJ used automated detection of individual cell outlines (phalloidin stained cells) of which approximately 20 cells were analysed on each material from three material replicates. Cell elongation was defined as the ratio of the maximal to the minimal cell length.
Results and discussion
Microtopography of honeycomb scaffolds
The SEM images showed that the scaffold had uniform-sized pores in a hexagonal arrangement (Fig. 1b). A cross-sectional SEM image of this honeycomb scaffold is shown in Fig. 1c. The pores were interconnected, and the bottom polymer layer and the top porous frames were connected with pillars. The average pore sizes (P) and frame widths (F) of the honeycomb scaffolds are shown in Table 1. For polymer 1, which is an amphiphile, three types of honeycomb scaffolds (HC1, HC2, and HC3) with different P and F parameters were prepared (Fig. 2a–c). The pore size of the honeycomb scaffolds depends on the size of the template water droplets, which can be controlled using the preparation conditions such as the humidity of the atmosphere and the casting volume.33 The honeycomb scaffolds using the DOPE amphiphile had 4.8 μm pores and 0.8 μm polymer frames (HC4, Fig. 2d), which was similar to the pore size of the scaffold shown in Fig. 2c, although the polymer frame was thinner. It has been reported that the width of the polymer frame decreases as the value of the interfacial tension between the water and the chloroform solution of the amphiphilic molecules decreases.34,35 The chloroform solution of DOPE greatly decreased the interfacial tension between the chloroform solution and the water compared with the polymer 1 solution; therefore, the width of the frame of the honeycomb scaffolds prepared with DOPE was thinner than those of polymer 1.
 |
| Fig. 2 SEM images of the HC1 (a), HC2 (b), HC3 (c), and HC4 (d) scaffolds. (Bar: 15 μm.) | |
Table 1 Scaffolds used in this experiment (P: average pore size, F: average frame width)
Substrate |
Amphiphile |
P (μm) |
F (μm) |
Glass |
— |
— |
— |
HC1 |
Polymer 1 |
1.6 |
0.4 |
HC2 |
Polymer 1 |
3.2 |
0.6 |
HC3 |
Polymer 1 |
4.7 |
1.6 |
HC4 |
DOPE |
4.8 |
0.8 |
During the formation of the honeycomb scaffolds, the amphiphiles stabilized the condensed water droplets. Because the amphiphiles condensed on the surface of pores, the honeycomb substrates were washed in 1-propanol and ethanol to remove amphiphiles before the cells were cultured, in order to exclude the effect of the different chemical properties of PS, polymer 1, and DOPE. Since only monomolecular layers of amphiphile were formed at the surface of pores, this extraction process did not affect the surface topography of honeycomb scaffolds (see ESI, S1‡).
Cell morphologies on honeycomb scaffolds
The hMSCs were cultured on four different substrates with low seeding densities in order to avoid concealing the cell–substrate interactions with cell–cell interactions. The fluorescence images of hMSCs cultured on a glass substrate and various honeycomb scaffolds after 24 h (upper column) and 2 weeks (bottom column) are shown in Fig. 3. After the cells were cultured for 24 h, the initial adhesion of the cells was observed, and the hMSCs on the glass substrate appeared to spread well and form highly organized actin stress fibers (Fig. 3a). However, the hMSCs on the HC1 and HC2 honeycomb scaffolds were much smaller than those on the glass substrate and formed more polygonal cell shapes (Fig. 3b and c). Fig. 3d and e show that the hMSCs on HC3 and HC4 were very elongated and had lamellipodial extensions. After 2 weeks, the hMSCs cultured on the glass substrate reached confluence (Fig. 3f), whereas the hMSCs cultured on the HC1 and HC2 honeycomb scaffolds formed discrete spots of intense cell aggregation approximately 200 μm in size (Fig. 3g and h). The hMSCs on the HC3 and HC4 honeycomb scaffolds showed increased cell densities and maintained their elongated shapes (Fig. 3i and j).
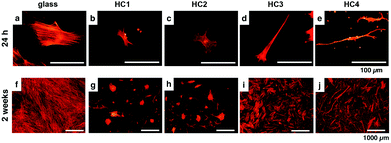 |
| Fig. 3 Fluorescence microscopic observation of cell morphology visualized with fluorescence-labeled phalloidin after 24 h (upper column) and 2 weeks (bottom column) culture on the (a), (f) glass, (b), (g) HC1, (c), (h) HC2, (d), (i) HC3, and (e), (j) HC4 scaffolds. Larger images were available in ESI (Fig. S2–1‡). | |
To understand the relationship between the microtopography and the hMSC behavior, we quantitatively analyzed the adhesive cell numbers, areas, and aspect ratios of hMSCs after they were cultured for 24 h (Fig. 4). Fig. 4a and b show that the numbers and areas of hMSCs adhered to the honeycomb scaffolds were fewer and smaller than those on the glass substrate, respectively. Moreover, the numbers and areas of the hMSCs adhered to the honeycomb scaffolds decreased as the pore size of the honeycomb scaffolds decreased. Furthermore, the hMSCs became polygonal and the aspect ratios of the hMSCs decreased as the pore sizes decreased (Fig. 4c). Because the width of the polymer frame also decreased as the pore size decreased, the surface area available for the cells to attach to was small for honeycomb scaffolds with smaller pores. When the width of the polymer frame decreased further (HC1 and HC2), limited numbers of cells attached themselves to the scaffold surfaces by adopting polygonal morphologies. This size effect was observed on scaffolds with subcellular scale features.36 Very elongated hMSCs were observed on the HC3 and HC4 scaffolds, which had a similar pore size to the hMSCs. Furthermore, the hMSCs on the HC4 scaffold, which had a similar pore size to the HC3 scaffold and thinner polymer frames, were more elongated than the hMSCs on the HC3 scaffold, because of the limited adhesion surface areas. Cell shapes are changed by the size of the focal adhesion, by which cells adhere onto the substrates. Since the size of the focal adhesion is in the range of several μm, the size of pores and polymer frames should be 10 μm and several μm, respectively. Because the cell shapes and the differentiation of stem cells are strongly related, the polygonal and elongated morphologies of the cells imply that the hMSCs underwent differentiation.37
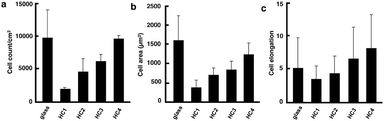 |
| Fig. 4 Plots of adhesive cell count (a), area (b), and elongation (c) after culturing for 24 h on honeycomb scaffolds. | |
Differentiation of hMSCs on honeycomb scaffolds
The preferential differentiation of hMSCs on the honeycomb scaffolds was confirmed by the immunofluorescent staining with the osteogenic marker, OPN, and a myogenic marker, MyoD1. The expression of osteogenic and myogenic protein was detected in hMSCs cultured for 1 and 2 weeks. Fig. 5 shows the immunofluorescent images of hMSCs on glass, HC1, HC2, and HC3 substrates stained with DAPI (upper column) and OPN (bottom column), respectively. The nuclei of cells were imaged with DAPI (Fig. 5a–d), and green OPN fluorescence was only observed on hMSCs with polygonal shapes (Fig. 3b and c) which were cultured on the HC1 and HC2 substrates (Fig. 5f and g). Engler et al. reported that hMSCs, whose shapes were controlled by changing the scaffold elasticity, differentiated into osteoblasts, myoblasts, and neurons from polygonal, elongated, and very elongated morphologies, respectively.38,39 Furthermore, McBeath et al. also reported that the initial low cell seeding density led to osteoblastic differentiation.40 The initial low cell density and polygonal shapes of the hMSCs on the HC1 and HC2 scaffolds, which had subcellular scale pores and thin polymer frames, mean that the hMSCs underwent osteospecific differentiation.
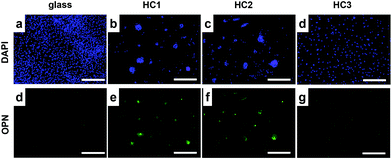 |
| Fig. 5 Osteopontin staining of hMSCs after culturing for 1 week. Upper column: DAPI (blue). Bottom column: osteopontin (green). (a), (f) Glass, (b), (g) HC1, (c), (h) HC2, (d), (i) HC3, and (e), (j) HC4 scaffolds. (Bar: 500 μm.) | |
Fig. 6 shows the immunofluorescent images of hMSCs cultured on the glass, HC2, HC3, and HC4 scaffolds stained with DAPI (upper column) and MyoD1 (bottom column), respectively. The green fluorescence from MyoD1 was clearly observed for the hMSCs on HC4, which had very elongated shapes, whereas no fluorescence was observed for the hMSCs on the glass, HC2, and HC3 substrates. This indicates that cellular scale pores and thin polymer films led to myospecific differentiation.
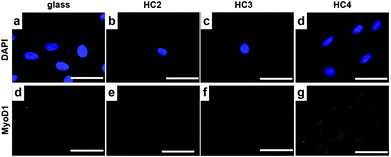 |
| Fig. 6 MyoD1 staining of hMSCs after culturing for 1 week. Upper column: DAPI (blue). Bottom column: MyoD1 (green). (a), (f) Glass, (b), (g) HC1, (c), (h) HC2, (d), (i) HC3, and (e), (j) HC4 scaffolds. (Bar: 50 μm.) | |
The chemical properties and surface elasticity of the substrate surfaces alter the adhesion formation in stem cells and other cell types.41–43 These changes in cell shape influence the cytoskeletal tension, which is generated by the cytoskeleton contraction at focal adhesions between the cytoskeleton and the substrate. These changes in the cytoskeletal tension have an effect on the mechanotransduction pathways of Rho A, which is an important small G-protein involved in cell signaling and cytoskeletal organization, in stem cells in response to surface elasticity.44,45 The changes in initial cell shape caused by the microtopography of the honeycomb scaffolds may influence the cell gene expression and the stem cell differentiation through the same mechanotransduction pathways as altering the chemical properties and elasticity of the scaffolds.
Conclusions
We have used honeycomb scaffolds to achieve the microtopography-induced differentiation of hMSCs without chemical induction using hazardous supplements, such as dexamethasone or ascorbate. The hMSCs were cultured on the honeycomb scaffolds in order to investigate the effect of the pore size and frame size of the honeycomb scaffold on the cell behavior. The honeycomb scaffolds exerted a strong influence on the cell morphology and differentiation. When both P and F were smaller than the cell size, hMSC differentiated to osteospecific differentiation. On the other hand, myospecific differentiation was observed when P was the same as the cell size and F was smaller than the cell size. To reveal molecular mechanism of these differentiations, further investigations including comprehensive gene expressions are required, but this selective differentiation strongly suggested that surface microtopography alone may be effective for using hMSCs in regenerative medicine and tissue engineering. These findings may be a milestone in controlling stem cells for therapeutic uses.
Notes and references
- G. A. Dunn and A. F. Brown, J. Cell Sci., 1986, 83, 313–340 CAS.
- P. Clark, P. Connoly, A. S. G. Curtis, J. A. T. Dow and C. D. Wilkinson, J. Cell Sci., 1991, 99, 73–77 Search PubMed.
- X. F. Walboomersa, H. J. E. Croesb, L. A. Ginselb and J. A. Jansena, Biomaterials, 1998, 19, 1861–1868 CrossRef.
- R. G. Flemming, C. J. Murphy, G. A. Abrams, S. L. Goodman and P. F. Nealy, Biomaterials, 1999, 20, 573–588 CrossRef CAS PubMed.
- A. J. Engler, S. Sen, H. L. Sweeney and D. E. Discher, Cell, 2006, 126(4), 677 CrossRef CAS PubMed.
- A. J. Engler, M. A. Griffin, S. Sen, C. G. Bonnemann, H. L. Sweeney and D. E. Discher, J. Cell Biol., 2004, 166, 877 CrossRef CAS PubMed.
- G. Mahmud, C. J. Campbell, K. J. M. Bishop, Y. A. Komarova, O. Chaga, S. Soh, K. Kandere-Grzybowska and B. A. Grzybowski, Nat. Phys., 2009, 5, 1–7 CrossRef.
- T. Tzvetkova-Chevolleau, A. Stéphanou, D. Fuard, J. Ohayon, P. Schiavone and P. Tracqui, Biomaterials, 2008, 29, 1541–1551 CrossRef CAS PubMed.
- W.-T. Su, Y.-F. Liao, C.-Y. Lin and L.-T. Li, J. Biomed. Mater. Res. A, 2010, 93, 1463–1469 Search PubMed.
- M. J. Poellmann, P. A. Harrell, W. P. King and A. J. Wagoner Johnson, Acta Biomater., 2010, 6, 3514–3523 CrossRef CAS PubMed.
- A. W. Holle and A. J. Engler, Curr. Opin. Biotechnol., 2011, 22, 648–654 CrossRef CAS PubMed.
- C. K. Choi, M. T. Breckenridge and C. S. Chen, Trends Cell Biol., 2010, 20, 705–714 CrossRef CAS PubMed.
- A. M. Ross, Z. Jiang, M. Bastmeyer and J. Lahann, Small, 2012, 8, 336–355 CrossRef CAS PubMed.
- S. B. Carter, Nature, 1965, 208, 1183 CrossRef CAS PubMed.
- M. J. Dalby, N. Gadegaard, R. Tare, A. Andar, M. O. Riehle, P. Herzyk, C. D. W. Wilkinson and R. O. C. Oreffo, Nat. Mater., 2007, 6, 997–1003 CrossRef CAS PubMed.
- E. K. Purcell, Y. Naim, A. Yang, M. K. Leach, J. M. Velkey, R. K. Duncan and J. M. Corey, Biomacromolecules, 2012, 13, 3427–3438 CrossRef CAS PubMed.
- M. A. Bucaro, Y. Vasquez, B. D. Hatton and J. Aizenberg, ACS Nano., 2012, 6(7), 6222–6230 CrossRef CAS PubMed.
- S. Meucci, I. Tonazzini, F. Beltram and M. Cecchini, Soft Matter, 2012, 8, 1109–1119 RSC.
- P. Bianco and P. G. Robey, Nature, 2001, 414, 118–121 CrossRef CAS PubMed.
- R. O. C. Oreffo, C. Cooper, C. Mason and M. Clements, Stem Cell Rev., 2005, 1, 169–178 CrossRef CAS PubMed.
- A. Mata, E. J. Kim, C. A. Boehm, A. J. Flleischman, G. F. Muschler and S. Roy, Biomaterials, 2009, 30, 4610–4617 CrossRef CAS PubMed.
- A. Ovsianikov, A. Deiwick, S. V. Vlieberghe, P. Dubruel, L. Möller, G. Dräger and B. Chichkov, Biomacromolecules, 2011, 12, 851–858 CrossRef CAS PubMed.
- Y.-C. Kuo and K.-H. Chiu, Biomaterials, 2011, 32, 819–831 CrossRef CAS PubMed.
- N. Maruyama, T. Koito, T. Sawadaishi, O. Karthaus, K. Ijiro, N. Nishi, S. Tokura, S. Nishimura and M. Shimomura, Supramol. Sci., 1998, 5, 331 CrossRef CAS.
- G. Widawski, M. Rawiso and B. François, Nature, 1994, 369(6479), 387–389 CrossRef CAS.
- H. Yabu and M. Shimomura, Chem. Mater., 2005, 17(21), 5231–5234 CrossRef CAS.
- L. A. Connal, R. Vestberg, C. J. Hawker and G. G. Qiao, Adv. Funct. Mater., 2008, 18(22), 3706 CrossRef CAS.
- J. S. Park, S. H. Lee, T. H. Han and S. O. Kim, Adv. Funct. Mater., 2007, 17(14), 2315 CrossRef CAS.
- D. Beattie, K. H. Wong, C. Williams, L. A. Poole-Warren, T. P. Davis, C. Barner-Kowollik and M. H. Stenzel, Biomacromolecules, 2006, 7(4), 1072 CrossRef CAS PubMed.
- Y. Fukuhira, E. Kitazono, T. Hayashi, H. Kaneko, M. Tanaka, M. Shimomura and Y. Sumi, Biomaterials, 2006, 27, 1797–1802 CrossRef CAS PubMed.
- T. Nishikawa, M. Nonomura, K. Arai, J. Hayashi, T. Sawadaishi, Y. Nishiura, M. Hara and M. Shimomura, Langmuir, 2003, 19, 6193 CrossRef CAS.
- T. Kawano, Y. Nakamichi, S. Fujinami, K. Nakajima, H. Yabu and M. Shimomura, Biomacromolecules, 2013, 14(4), 1208–1213 CrossRef CAS PubMed.
- H. Yabu and M. Shimomura, Langmuir, 2005, 21(5), 1709–1711 CrossRef CAS PubMed.
- H. Yabu, M. Tanaka, K. Ijiro and M. Shimomura, Langmuir, 2003, 19(15), 6297–6300 CrossRef CAS.
- M. Kojima, Y. Hirai, H. Yabu and M. Shimomura, Polym. J., 2009, 41, 667–671 CrossRef CAS.
- Y. Fukuhira, H. Yabu, K. Ijiro and M. Shimomura, Soft Matter, 2009, 5(10), 2037–2041 RSC.
- M. Massumi, M. Abasi, H. Babaloo, P. Terraf, M. Safi, M. Saeed, J. Barzin, M. Zandi and M. Soleimani, Tissue Eng. Part A, 2012, 18(5–6), 609–620 CrossRef CAS PubMed.
- A. J. Engler, S. Sen, H. L. Sweeney and D. E. Discher, Cell, 2006, 126, 677–689 CrossRef CAS PubMed.
- A. J. Engler, M. A. Griffin, S. Sen, C. G. Bonnemann, H. L. Sweeney and D. E. Discher, J. Cell Biol., 2004, 166, 877–887 CrossRef CAS PubMed.
- R. McBeath, D. M. Pirone, C. M. Nelson, K. Bhadriraju and C. S. Chen, Dev. Cell, 2004, 6(4), 483–495 CrossRef CAS PubMed.
- N. Xia, C. K. Thodeti, T. P. Hunt, Q. Xu, M. Ho, G. M. Whitesides, R. Westervelt and D. E. Ingber, FASEB J., 2008, 22, 1649–1659 CrossRef CAS PubMed.
- W.-H. Guo, M. T. Frey, N. A. Burnham and Y.-L. Wang, Biophys. J., 2006, 90, 2213–2220 CrossRef CAS PubMed.
- T. Kawano and S. Kidoaki, Biomaterials, 2011, 32, 2725–2733 CrossRef CAS PubMed.
- J. T. Parsons, A. R. Horwitz and M. A. Schwartz, Nat. Rev. Mol. Cell Biol., 2010, 11, 633–643 CrossRef CAS PubMed.
- B. Geiger, J. P. Spatz and A. D. Bershadsky, Nat. Rev. Mol. Cell Biol., 2009, 10, 21–33 CrossRef CAS PubMed.
Footnotes |
† This work has been partially supported by Grant-in-Aid for Challenging Exploratory Research (No. 23651100). |
‡ Electronic supplementary information (ESI) available. See DOI: 10.1039/c3bm60195a |
|
This journal is © The Royal Society of Chemistry 2014 |
Click here to see how this site uses Cookies. View our privacy policy here.