DOI:
10.1039/C3LC51065D
(Technical Innovation)
Lab Chip, 2014,
14, 178-181
A chip-to-world connector with a built-in reservoir for simple small-volume sample injection†
Received
19th September 2013
, Accepted 28th October 2013
First published on 29th October 2013
Abstract
We present a novel connector that allows for easy handling and injection of sample volumes between 1 and 20 μl. All tubing connections between external pumps and the microfluidic device are established before the sample is introduced into a sealable reservoir built into the connector. This approach allows for multiple injections of small sample volumes without the need to dismantle the chip-tubing assembly. We demonstrate that the connector reservoir seal can withstand pressures of up to 6 bar, that opening or closing the reservoir does not dislocate the sample by more than 35 nl, and that the connector can be used for injecting samples into both miscible and immiscible carrier fluids.
1. Introduction
One of the most common practical challenges encountered when working with microfluidic systems is the realization of a robust interface between the device and the outside world. A variety of connection systems have been developed or adapted for use with chip-based microfluidic devices.1,2 These include small hollow steel cylinders connecting plastic tubing to input holes punched into elastomeric substrates,3 pressure-seal chromatography connectors,4,5 D-subminiature connectors,6 fit-to-flow interconnects,7 gaskets and manifolds,8–10 or simply direct insertion of tubing straight into the device.11 However, regardless of the connection system, the handling of fluid volumes of the order of 20 microlitres or less using standard connectors and tubing remains a challenge. This is because in order to efficiently inject such a small volume into a microfluidic device, one cannot simply load the sample into a syringe or pressurised vessel and then transfer it through several millimetres or centimetres of tubing. Such an approach is constrained by the accumulated dead volume of tubing, connectors, containers, and syringes, meaning that either the sample volume must be significantly larger than what is actually injected, or that a carrier fluid must be used to push the sample through.
A common procedure for introducing small-volume samples into a microfluidic channel involves loading the sample into the device-end of narrow bore tubing, which is then inserted into the chip.12,13 Unfortunately, this approach often results in the introduction of gas bubbles, or premature injection of the sample into the channels due to pressure fluctuations during insertion. In the worst case, the sample may be completely lost if the end of the tubing suffers accidental vibration prior to connection. An alternative approach is to pipette a droplet of sample directly over the channel inlet and draw it into the microfluidic system by applying vacuum at the outlet. However, this method also introduces bubbles into the device if any air is trapped between the sample and the inlet, and also greatly limits flow control possibilities due to the fact that suction must be applied at the outlets and at least one inlet must be left open to the atmosphere. It goes without saying that handling multiple small samples becomes exceptionally difficult in situations where this approach is taken. Furthermore, while the sample stands open to air over the inlet, it is vulnerable to contamination, evaporation, or dislocation. It should be noted that an extremely effective approach to handling small volume samples is to integrate sample reservoirs within the chip substrate itself, and implement integrated pumping systems, such as those based on “Quake valves” or electrokinetic flow.14–19 This approach certainly solves many of the aforementioned problems, but at a cost of introducing significant complexity to chip design, fabrication, and operation. Moreover, such techniques require dedicated peripheral equipment around the fluid system.
Herein we propose a new approach to fluidic interconnection (Fig. 1) that provides a simple and direct interface between external tubing and a microfluidic device, is compatible with conventional syringe and pressure pumps, and allows the user to introduce samples by pipetting into a sealable reservoir built into the connector. The connector has three openings: one that connects to the chip, one to interface with tubing and external pumps, and one for introducing sample. All tubing and pump connections are made prior to sample introduction, de-coupling the process of sample loading from fluidic network assembly. The sample reservoir is sealed by a sliding cap, which minimises pressure oscillations during opening and closing and thus avoids premature sample injection. Importantly, if the reservoir is capped while overflowing, air bubbles are not introduced into the chamber. Finally, it should be noted that the resealable sliding cap can be reopened for the introduction of additional sample without requiring any tubing disconnection. Fig. 2 schematically illustrates the process of sample introduction using the connector. This new connector makes injection of sub-20 μl volumes into microfluidic devices a very simple operation, thus eliminating some of the practical complications associated with handling small samples and making microfluidic technology more accessible.
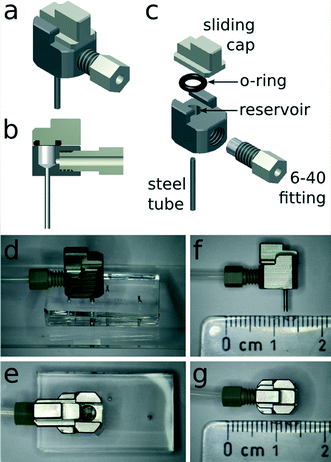 |
| Fig. 1 (a) A schematic of the connector design and (b) its cross-section. (c) An exploded view of the connector showing the sliding cap, o-ring, reservoir, 6-40 fitting for tubing connection, and the steel tube that inserts into the microfluidic device. (d) The connector integrated with a microfluidic device and (e) a top view of the open reservoir. (f and g) The connector next to a scale, showing its dimensions: 8 mm long (without fitting), 7 mm wide, and 10 mm tall (without steel tube). | |
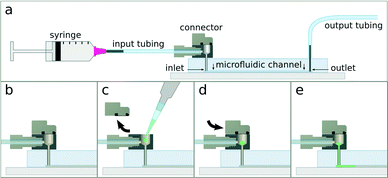 |
| Fig. 2 Schematic of connector operation. (a) Connect all tubing, pumps, and microfluidic device according to experiment. (b) Fill the device with a carrier fluid. (c) Open the connector lid, and pipette the sample into the reservoir. (d) Close the connector lid. (e) Pump the sample into the microfluidic channels. | |
2. Interconnect fabrication
The connector is manufactured using standard mechanical workshop milling techniques (AutoDesk Inventor 2013 technical 3D drawing available in ESI†). The main body of the connector and the sliding cap are milled from steel or polyether ether ketone (PEEK). Hollow steel pin connectors (Instech Laboratories, USA) with a 1 mm outer diameter are used to link the built-in reservoir to the microfluidic channel inlet. The pins are fixed into a 0.98 mm hole drilled through the connector into the reservoir. In the current study, we use either hollow steel pin connectors or Vici 6-40 threaded PEEK fittings (Vici AG, Switzerland) to interface with external tubing, though the design may be modified for compatibility with other commercially available connector systems. When a Vici 6-40 fitting is used to connect 1/16th inch outer diameter tubing, a 4 mm hole is drilled and tapped with 40 threads per inch. The reservoir inside the connector has a maximum volumetric capacity of 20 μl. It is closed by a manually-operated sliding cap, which is also milled from steel or PEEK, whilst the seal is provided by a 1 mm thick Viton rubber O-ring (Georg Rutz AG, Switzerland) with a 2.5 mm inner diameter. The O-ring is stretched and inserted into a 1 mm wide groove with a 3 mm inner diameter and a depth of 0.8 mm milled into the bottom of the sliding cap. Since the connector can be fabricated from a variety of materials, the surface characteristics of the reservoir and internal vias can be tailored to different requirements. Even though stainless steel is generally compatible with many chemical and biological applications, its surface can, for example, be electroplated with gold or other metals or grafted with PEG for improved biocompatibility.20 Moreover, due to the flexible design and simple manufacturing process, multiple inputs can be integrated into the same connector block, allowing several input flow streams to be handled simultaneously. In the current work, we show the use of up to three inputs (Fig. 3).
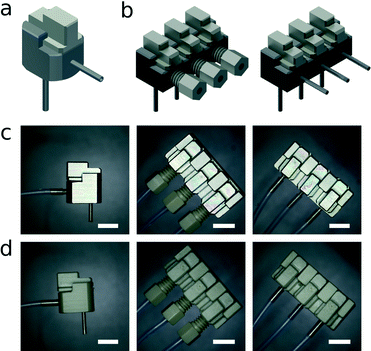 |
| Fig. 3 Different connector embodiments. (a) A connector with a steel tube input interface instead of a 6-40 fitting. (b) 3-way connector blocks with three independent reservoirs, inputs, and outputs. (c) Stainless steel versions and (d) PEEK versions of single and 3-way connectors. The scale bars all represent 5 mm. | |
3. Interconnect testing and operation
The pressure tolerance of the connector was tested by connecting it to sealed tubing at one end, and to a compressed air source at the other. Air pressure was then increased while the connector was submerged in water and monitored for the emergence of air bubbles. For repeated use testing, the cap was completely removed and reinserted before the pressure tolerance was reassessed. It was found that the seals within the connector were able to withstand at least 6 bar of pressure without leaking. The interface between the connector and a 4 mm thick PDMS elastomer (Sylgard 184, Dow Corning, USA) microfluidic device, with inlet cored by a 0.89 mm diameter hole puncher, was tested in a similar manner. It was found that the connection could withstand at least 4 bar of pressure before the microfluidic device delaminated from the substrate. However, the robustness of the connection will most likely be different depending on the thickness of the elastomer substrate, material elasticity, and diameter and quality of the tool used to core the inlets. This is well beyond the requirements of most microfluidic applications, which typically operate in pressure regimes of 2 bar or less. It was also found that even after 250 cycles of opening and closing the reservoir, the sliding cap seal did not leak under 6 bar of pressure. This open/close cycle test was repeated with five different O-rings, with similar results, demonstrating that the quality of the seal provided by the connector assembly is well suited for most microfluidic applications.
The occurrence of sample dislocation during the opening and closing of the reservoir was also investigated. The connector reservoir and tubing were completely filled with mineral oil, clamped down, and connected to a graduated 5 μl glass capillary (minimum graduations of 15 mm per 1 μl) via flexible silicone tubing. As the capillary was connected, oil flowed into it, forming an oil–air (O/A) interface inside. The reservoir was then opened and the loose end of the capillary raised in order to balance gravitational and capillary forces acting on the fluid. An illustration of the process is provided in Fig. 4. When the O/A interface was stationary, the sliding cap was closed and opened repeatedly (10 cycles), and the position of the O/A interface observed during each operation. The distance moved by the O/A interface during opening or closing was never more than 500 μm, corresponding to a maximum sample displacement smaller than 35 nl. These data show that the connector is effective at preventing untimely injection of the sample into the microchannels, since the dead volume inside the connector pin is far greater than 35 nl. Importantly, this means that the interconnect is ideally suited to applications where injection timing and sequence are important. It should be noted that typical disturbances such as bending of the tubing normally dislocate volumes greater than 35 nl.
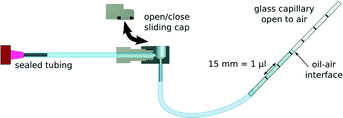 |
| Fig. 4 A schematic of the sample displacement test setup. The connector is linked to a 5 μl graduated glass capillary via flexible silicone tubing at the output, and to sealed plastic tubing at the input. The system is filled with oil such that an oil–air interface is formed inside the glass capillary, and the capillary raised so that there is no movement of fluid while the reservoir is left open. To measure sample displacement, the position of this interface is observed during opening and closing of the sliding cap. For clarity, the clamping system used to prevent unwanted movement of the connector and tubing is not shown. | |
Finally, to demonstrate sample injection, we inserted the connector into a simple straight-channel silicone elastomer microfluidic chip. The whole system, including tubing, channels, and connector reservoir, was flooded with carrier fluid. A 5 μl sample of aqueous food colouring was then pipetted into the reservoir, the cap closed, and the sample injected into the channel via a syringe pump at 10 μl min−1. As depicted in Fig. 5(a), sample injection was completed in precisely 30 seconds when mineral oil containing 2% Span80 (Sigma, USA) was used as a carrier fluid, which is to be expected for a 5 μl sample flowing at 10 μl min−1. When water was used as the carrier fluid, however, both the leading and trailing ends of the sample plug were diffuse into the carrier fluid. As shown in Fig. 5(b), the injection lasted much longer than 30 seconds due to sample dilution. Additional videos demonstrating the injection of nematode worms and yeast cells are available in the ESI.†
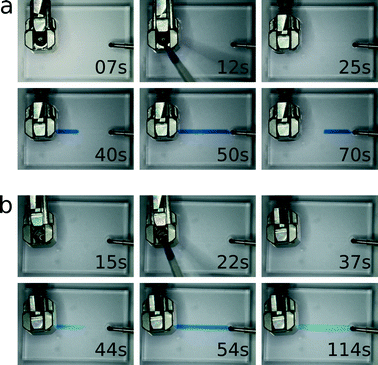 |
| Fig. 5 A timed sequence of images taken of the connector mounted on a simple straight-channel silicone elastomer microfluidic chip and being used to inject a 5 μl sample of food colouring at 10 μl min−1. The sample is injected with mineral oil containing 2% Span80 (a) and water (b) as carrier fluids. It takes exactly thirty seconds to inject the entire sample when using an oil carrier, while the start and end of the sample plug are both diffuse when using a water carrier. Movie files are available in the ESI.† | |
4. Conclusions
We have demonstrated a robust chip-to-world connector that allows on-demand injection of small-volume samples (1–20 μl) into microfluidic devices while still allowing for external pumps to be connected via conventional tubing. Although in this work the connector was tested with a PDMS elastomeric device, it may also be used with other, more rigid materials such as glass. In those cases, the interface between the connector and the channel inlet would need to be sealed with epoxy or another compatible sealing agent. The connector allows for sample loading to be performed after all tubing assemblies have been made, thus eliminating the risk of sample loss or premature injection. The connector is inexpensive to manufacture, while its simple operation and reusability allow for multiple samples to be injected sequentially. Due to its flexible design, connector blocks with multiple tubing–reservoir–inlet interfaces can be manufactured and operated independently.
References
- B. Gray, D. Jaeggi, N. Mourlas, B. van Drienhuizen, K. Williams, N. Maluf and G. Kovacs, Sens. Actuators, A, 1999, 77, 57–65 CrossRef CAS.
- C. K. Fredrickson and Z. H. Fan, Lab Chip, 2004, 4, 526–533 RSC.
- A. M. Christensen, D. A. Chang-Yen and B. K. Gale, J. Micromech. Microeng., 2005, 15, 928 CrossRef.
- V. Nittis, R. Fortt, C. H. Legge and A. J. de Mello, Lab Chip, 2001, 1, 148–152 RSC.
- B. J. Kirby, D. S. Reichmuth, R. F. Renzi, T. J. Shepodd and B. J. Wiedenman, Lab Chip, 2005, 5, 184–190 RSC.
- A. Scott, A. K. Au, E. Vinckenbosch and A. Folch, Lab Chip, 2013, 13, 2036–2039 RSC.
- A. Chen and T. Pan, Lab Chip, 2011, 11, 727–732 RSC.
- D. Snakenborg, G. Perozziello, O. Geschke and J. P. Kutter, J. Micromech. Microeng., 2007, 17, 98 CrossRef.
- S. Miserendino and Y.-C. Tai, Sens. Actuators, A, 2008, 143, 7–13 CrossRef CAS PubMed.
- G. A. Cooksey, A. L. Plant and J. Atencia, Lab Chip, 2009, 9, 1298–1300 RSC.
- C.-H. Chiou and G.-B. Lee, J. Micromech. Microeng., 2004, 14, 1484 CrossRef CAS.
- D. N. Adamson, D. Mustafi, J. X. J. Zhang, B. Zheng and R. F. Ismagilov, Lab Chip, 2006, 6, 1178–1186 RSC.
- W.-A. C. Bauer, M. Fischlechner, C. Abell and W. T. S. Huck, Lab Chip, 2010, 10, 1814–1819 RSC.
- M. A. Unger, H.-P. Chou, T. Thorsen, A. Scherer and S. R. Quake, Science, 2000, 288, 113–116 CrossRef CAS.
- J. Liu, C. Hansen and S. R. Quake, Anal. Chem., 2003, 75, 4718–4723 CrossRef CAS.
- S. Kondapalli and B. Kirby, Microfluid. Nanofluid., 2009, 7, 275–281 CrossRef CAS.
- G. A. Cooksey, C. G. Sip and A. Folch, Lab Chip, 2009, 9, 417–426 RSC.
- S. Zeng, C.-H. Chen, J. C. Mikkelsen Jr and J. G. Santiago, Sens. Actuators, B, 2001, 79, 107–114 CrossRef CAS.
- J. M. Edwards, IV, M. N. Hamblin, H. V. Fuentes, B. A. Peeni, M. L. Lee, A. T. Woolley and A. R. Hawkins, Biomicrofluidics, 2007, 1, 014101 CrossRef PubMed.
- F. Zhang, E. Kang, K. Neoh, P. Wang and K. Tan, Biomaterials, 2001, 22, 1541–1548 CrossRef CAS.
Footnote |
† Electronic supplementary information (ESI) available. See DOI: 10.1039/c3lc51065d |
|
This journal is © The Royal Society of Chemistry 2014 |
Click here to see how this site uses Cookies. View our privacy policy here.