DOI:
10.1039/C3MT00208J
(Paper)
Metallomics, 2014,
6, 96-104
Zinc to cadmium replacement in the prokaryotic zinc-finger domain
Received
31st July 2013
, Accepted 6th November 2013
First published on 7th November 2013
Abstract
Given the similar chemical properties of zinc and cadmium, zinc finger domains have been often proposed as mediators of the toxic and carcinogenic effects exerted by this xenobiotic metal. The effects of zinc replacement by cadmium in different eukaryotic zinc fingers have been reported. In the present work, to evaluate the effects of such substitution in the prokaryotic zinc finger, we report a detailed study of its functional and structural consequences on the Ros DNA binding domain (Ros87). We show that this protein, which bears important structural differences with respect to the eukaryotic domains, appears to structurally tolerate the zinc to cadmium substitution and the presence of cadmium does not affect the DNA binding activity of the protein. Moreover, we show for the first time how zinc to cadmium replacement can also take place in a cellular context. Our findings both complement and extend previous results obtained for different eukaryotic zinc fingers, suggesting that metal substitution in zinc fingers may be of relevance to the toxicity and/or carcinogenicity mechanisms of this metal.
1 Introduction
Structural metal cofactors perform crucial roles in proteins as stabilizing elements of a specific fold in the native state and often as potential nucleation points during folding.1,2 Their binding imposes conformational restrictions on the unfolded protein favouring a specific folding mechanism.3
In the case of the eukaryotic zinc finger domain, protein folding is totally zinc-dependent.4–6 Zinc, in combination with hydrophobic residues, stabilizes the active ββα fold of the domain that consists of less than 30 amino acids.5–7 Some divalent metal ions (such as cadmium, lead, copper, etc.) have been shown to be able to displace the zinc ion from the domain coordination core.8–14 Knowing the important role played by zinc fingers, this has been proposed as a potential mechanism for the toxic and carcinogenic effects exerted by xenobiotic metals.9,13,15–27 Among them cadmium is one of the highly toxic transition metals to human beings, universally identified as a serious environmental pollutant, due to its relatively high mobility in soils and potential toxicity even at low concentrations.28–37 Given the similar chemical properties of zinc and cadmium, effects of zinc replacement by cadmium in different eukaryotic zinc fingers, with special attention to the metal modulation of the DNA binding activity, have been widely studied.13,15,18,21,23,38,39
Bacteria have been shown to be susceptible to metal pollution. In fact, while very low levels of metals are essential for them, bacteria often take up cations up to harmful concentrations.40–42 Some micro-organisms have adapted themselves to deal with the toxicity of increased metal concentrations by developing a variety of resistance mechanisms.40,43–46 These mechanisms include the extracellular precipitation and exclusion of metal ions to the outer surface of bacteria, reduction of metals to less toxic species, and direct efflux of them out of the cell.42,44,47 However, in the majority of the bacteria, heavy metals exert their toxicity via the known mechanisms of inducing oxidative stress and of interfering with proper protein folding and functions. Recently, a novel class of zinc-finger proteins have been found in bacteria.48 The first member of this prokaryotic family to be structurally and functionally characterized has been the protein Ros from A. tumefaciens.49,50 Ros87 NMR structure, a functional mutant of the Ros wild-type obtained by deletion of the first fifty-five amino acids (here named Zn-Ros87), has revealed how the prokaryotic zinc finger bears a novel fold with important structural differences with respect to its eukaryotic counterpart.50 In particular, the Ros87 globular fold has a βββαα topology and it is stabilized by an extended hydrophobic core of 15 amino acids50 (Fig. 1). Although Ros87 shows five possible coordinating residues (namely Cys24, Cys27, His37, His41 and His42), we have demonstrated that Cys24, Cys27, His37 and His42 bind the zinc ion with high affinity; when His42 is mutated in Ala, His41 is able to occupy the fourth position of the zinc coordination sphere.49
 |
| Fig. 1 Ros87 sequence: the coordinating residues are in red; the secondary structure elements are also reported. | |
Here we report a detailed study of the functional and structural effects of zinc to cadmium substitution on this new domain structure. The characterization of the cadmium binding to Ros87, the exploration of how its structural effects are reflected on the protein function and the comparison to what is already known about such effects on the eukaryotic domain are presented and can be useful to clarify the chemistry of cadmium in relation to metal-dependent proteins and to the mechanism of cadmium toxicity.
Moreover, in vitro cadmium substitution of native metals in the binding sites of many proteins has been described.18,23,39 However, although such substitution is very often proposed as the leading molecular mechanism of cadmium toxicity, only a few of these in vitro studies have been confirmed in vivo. For these reasons, in order to further address the relevance of this mechanism, we have verified if such a replacement can take place in a cellular context.
2 Methods
Protein production and purification
Unlabelled and single labelled (15N Zn-Ros87) proteins used for the NMR and UV-Vis experiments were over-expressed and purified as previously reported.49,51
Preparation of 15N Cd-Ros87
Unlabelled and labelled Zn-Ros87 were acidified at pH 2.0 by adding HCl 0.1 M and dialyzed with an aqueous solution containing 4 mM TCEP (Tris(2-carboxyethyl) phosphine) at pH 2.0 to remove the zinc ion. Cd-Ros87 was prepared by adding 1.2 molar equivalents of Cd(II) from a CdCl2 solution to apoRos87 after a second dialysis in aqueous solution containing 4 mM TCEP at pH 6.8.
Cd-Ros87 electromobility shift assay (EMSA) analysis
60 pmol of the purified Cd-Ros87 were incubated for 10 min on ice with 2.5 pmol of the duplex oligonucleotides VirC, 5′-GATTTTATATTTCAATTTTATTGTAATATAATTTCAATTG-3′, or NS (5′-TGGCCAGGGCCGCGCCGTGGCGGGGCCAGGGCGCGGGGCT-3′) as a nonspecific competitor. The experiments were conducted as previously described.49 The gel was then stained with SYBR Green and visualized using a Typhoon Trio ++ scanner (GE Healthcare).
UV-Vis spectroscopy
UV-Vis spectra were recorded on a Nanodrop2000c spectrophotometer from 200 to 350 nm at 295 K. The apoRos87 concentration was calculated by absorbance at 280 nm. ApoRos87 (7.97 μM) was dissolved in 20 μM TCEP at pH 6.8, and CdCl2 (1.0 mM) was added up to a 1.5/1.0 Cd/apoRos87 ratio. The cadmium binding affinity constant was calculated by monitoring the absorbance at 240 nm, corresponding to the S− → Cd(II) ligand-to-metal charge-transfer transition.13,52,53 The calculation of binding constant has also been repeated at 245 nm and 250 nm wavelengths.
NMR spectroscopy
NMR samples contained typically a 1 mM solution of 15N Cd-Ros87 and 4 mM TCEP at pH 6.8 in 550 μl of 90% H2O/10% 2H2O. All the experiments were carried out on a Varian Unity INOVA 500 MHz spectrometer. 1H–15N-HSQC spectra were recorded with 256 complex points for 15N (F1) and 2048 for 1H (F2) while 1H–15N-HSQC J-18 spectra were acquired as previously described.49 All the NMR experiments were processed using Varian software VNMR6.1B and 1H, and 15N chemical shifts were calibrated indirectly by using TMS as external reference. The program XEASY54 was used to analyze and assign the spectra. The structures were visualized using the program MOLMOL.55
1HN and 15N chemical shift perturbations were estimated by using the following equation:56–58
Δδ = [(ΔHN2 + (ΔN/5)2)/2]1/2 |
where Δ
HN and Δ
N are the differences between the
1H and
15N chemical shifts of Zn-Ros87
50 and Cd-Ros87.
The 1H–15N HSQC Cd-Ros87 displacement experiments were performed by adding Zn(II) as ZnCl2 solution to the Cd-Ros87 sample in the following Zn
:
protein concentration ratios: 0.2
:
1, 0.5
:
1, 0.7
:
1, 1
:
1, 1.2
:
1, 1.5
:
1, 2
:
1.
Cd-Ros87cell protein production and purification
For the synthesis of 15N-labeled Cd-Ros87cell protein E. coli BL21(DE3) host strain was used. The cells were grown at 37 °C in the minimal medium (which contained a Zn(II) concentration of (1.36 ± 0.5) mg L−1) until an OD600 value of 0.5 was reached; then, Cd(II), from a CdCl2 solution, at increasing concentrations of 0.05 mM, 0.100 mM, 0.150 mM, 0.200 mM, 0.300 mM and 0.600 mM was added to six vials containing cells. Successively, at the OD600 value of 0.6 (after about 30 min of exposure) the synthesis of Cd-Ros87cell was induced for 75 min adding IPTG 1 mM and the cell growth was monitored by measuring the OD600. Whole cell lysates were loaded and run on 18% SDS PAGE and the separated proteins were visualized by staining the gel with Coomassie Brilliant Blue R250 considering as control whole lysates of untreated cells. The comparison of untreated cells with those treated indicates that up to the concentration of 0.6 mM of Cd(II) the synthesis level of Cd-Ros87cell was unaffected. Then, for the NMR analysis 15N single-labelled Cd-Ros87cell was produced in the presence of 0.6 mM Cd(II) concentration and purified as already reported.49 The NMR samples contained typically a 1 mM solution of 15N Cd-Ros87cell and 4 mM TCEP at pH 6.8 in 550 μl of 90% H2O/10% 2H2O.
ICP-MS
The amount of cadmium and/or zinc contained in Cd-Ros87cell and Cd-Ros87 proteins was measured by inductively coupled argon plasma mass spectrometry (ICP-MS). The recombinant proteins (7 μM) were dialysed with pure water and then acidified (pH 2) by adding HCl 0.1 M to obtain the release of the metal ion. As a blank, a sample without the protein was prepared in the same way.
3 Results
Unstructured apoRos87 binds Cd(II)
The 1H–15N HSQC spectrum of Cd-Ros87 is shown in Fig. 2 (blue peaks); the good dispersion of the cross-peaks in both proton and nitrogen dimensions indicates that upon Cd(II) addition the unstructured apoRos87 folds into a well defined structure with extensive tertiary interactions.51 The superposition of this spectrum on the 1H–15N HSQC of Zn-Ros87 (Fig. 2), recorded under the same conditions, suggests that Cd-Ros87 (blue signals) retains globally the same fold of Zn-Ros87 (pink signals) with only small local rearrangements.
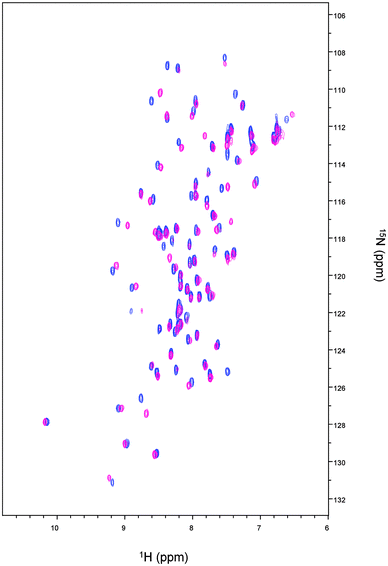 |
| Fig. 2 Superposition of Zn-Ros87 (pink) and Cd-Ros87 (blue) 1H–15N HSQCs spectra acquired at 298 K. | |
We have previously shown that Cys24, Cys27, His37 and His42 are involved in the zinc coordination and that His41 can replace His42 in the coordination sphere, when His42 is mutated to alanine.49,59 To identify which of the two histidines occupying the fourth coordinating position is involved in Cd(II) coordination, a 1H–15N-HSQC J-18 experiment has been acquired (Fig. 3 – blue cross peaks). This experiment allows the coherence transfer from Hε1 and Hδ2 protons to Nε2 and Nδ1 nitrogens through the 2JHN coupling constants.60,61 It shows in the case of Zn-Ros87 (Fig. 3 – pink cross peaks) that His37 and His42 are involved in the zinc coordination both being Nδ1–H tautomers (i.e., with Nδ1 protonated and Nε2 unprotonated) and having both Nε2 and Nδ1 chemical shifts typical of zinc binding histidine side-chains. On the other hand, His41 having a Nδ1 chemical shift that is largely out of the range typical for unprotonated nitrogens of zinc binding histidines is not involved in the coordination of the metal ion.49,61 Interestingly, the superposition of the 1H–15N-HSQC J-18 spectrum of Cd-Ros87 with that of Zn-Ros87 (Fig. 3 – blue and pink signals, respectively) confirms that the histidines 37 and 42 are again involved in metal coordination retaining the same pattern shown by a Nδ1–H tautomer. Interestingly, 15N chemical shifts induced by Cd(II) binding fall into the chemical shift range typical of a zinc binding histidine side-chain.
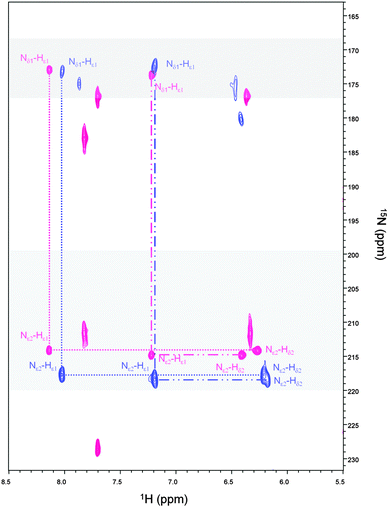 |
| Fig. 3 Superposition of Zn-Ros87 (pink) and Cd-Ros87 (blue) 1H–15N HSQC-J18 spectra acquired at 298 K. The gray rectangles indicate the nitrogen chemical-shift ranges typical for His involved in metal coordination. The assignments of the cross-peaks indicate that His37 and His42 are also involved in the coordination of the cadmium. | |
Cd(II) binding affinity
The affinity of apoRos87 for the zinc ion (Kd = 3.6 (±0.8) × 10−8 M) has been previously indirectly determined via UV-Vis spectroscopy at 290 nm, by monitoring the Co(II) displacement from the Co-Ros87 complex in a quantitative titration with Zn(II).51 Here, given the spectroscopic properties of cadmium complexes, the cadmium binding affinity of Ros87 was directly investigated via UV-Vis spectroscopy by quantitatively titrating the apo-protein with cadmium (Fig. 4)13,53,62 obtaining a comparable Kd value of 1.98 (±0.82) × 10−8 M.51
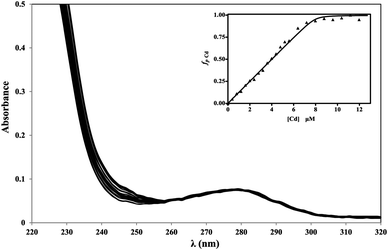 |
| Fig. 4 Cd(II) titration of apoRos87 in 20 μM TCEP pH 6.8 at 295 K. Inset: UV-titration curve at 240 nm. | |
Cd(II) displacement by Zn(II) in Cd-Ros87
On the basis of the obtained Kds, Cd(II) displacement by Zn(II) was monitored by NMR spectroscopy. Cd-Ros87 protein was titrated with ZnCl2 and a series of 1H–15N-HSQC spectra were recorded. The superposition of the spectra recorded in the presence of 0.2 (black peaks) and 1.0 (gold peaks) equivalents of Zn(II) are compared in Fig. 5, which shows how at 0.2 Zn(II) equivalents (black peaks) most of the peaks shift toward the position occupied by the same resonance in the spectrum of Zn-Ros87, according to a fast exchange regime. At 1.0 Zn(II) equivalents (gold peaks) the spectrum is coherent with the magnitude of the obtained Kds. A closer look at all the spectra shows that the peaks corresponding to the residues Leu25, Glu26 (i.e.Fig. 5 circled resonances), Cys27, Gly28, Gly29, Leu32, Ser33 and Leu34, located close to the coordination sphere, are in slow-exchange. In particular, for these residues at 1.0 Zn(II) equivalents weak residual signals for the Cd-Ros87 are still visible. In fact, as the zinc concentration gradually increases, these peaks slowly disappear while the corresponding peaks of the Zn(II) bound complex become more intense.
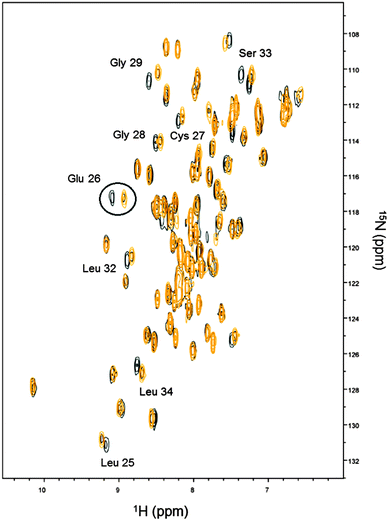 |
| Fig. 5 Titration of Cd-Ros87 with zinc. The figure reports the superposition of the 1H–15N HSQC spectrum recorded in the presence of 0.2 equivalents of zinc (black) with the same spectrum recorded in the presence of 1.0 equivalents (gold). The circled resonances are assigned to Glu26. | |
Mapping of the 1HN and 15N chemical shift variations onto Zn-Ros87 structure
1HN and 15N chemical shift assignment of the HSQC spectrum of Cd-Ros87 was obtained by transferring each previously resonance assignment of Zn-Ros8750 to the nearest resonance in the same spectrum for Cd-Ros87. The experiment described in the previous section guarantees the correct assignment as the fast exchange regime permits us to follow the resonances to their final positions. The chemical shift deviations observed using this method can be considered to reliably indicate changes in the local environment of the originating residues. To assess such changes, the weighted average of the chemical shift deviations obtained comparing the backbone amide 1H and 15N chemical shifts of Cd-Ros87 with those of Zn-Ros87 have been calculated for each residue of the protein globular domain (residue 9–66) and the data plotted out as a function of residue number (Fig. 6). As expected, resonances arising from the residues immediately close to the metal binding site experience the largest chemical shift changes. To visualize the location of these regions, we mapped sites with weighted chemical shift deviations above a threshold value ≥ of 0.4 ppm onto the NMR structure of Zn-Ros87 (Fig. 7). The threshold value was chosen heuristically based on the visual inspection of the data with the intent to include all regions that display significant chemical shift changes. It should be noted that the residues showing the major changes are located in the protein regions ranging from position 24 to 28 and from position 32 to 43. These regions include the β-turn connecting β2 and β3 strands that contain the two coordinating cysteines, the loop that connects β3 and the first α-helix (α-1) and α-1 where the two coordinating histidine are located.50 Other important chemical shift perturbations are observed for residues 51, 53 and 57 that in the globular structure of Zn-Ros87 are in the spatial vicinity of the metal binding site. In particular, Trp53 located in the last turn of the second α-helix in Zn-Ros87 is anchored to Glu26 through a key stabilizing hydrogen bond.50
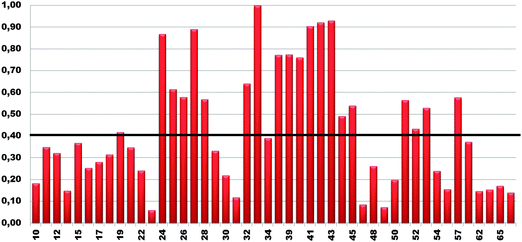 |
| Fig. 6 Normalized weighted average chemical shift differences (Δav/Δmax) for Cd-Ros87 plotted against residue number (residue 10–66 of Zn-Ros87 globular domain) for amide proton and nitrogen resonances. | |
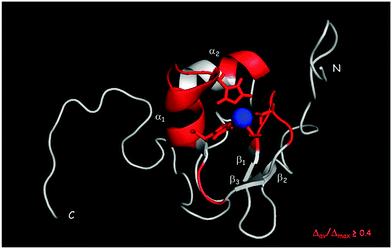 |
| Fig. 7 Normalized weighted average shift differences Δav(HN) ≥ 0.4 mapped onto a ribbon representation of a representative conformer of the Zn-Ros87 structure. | |
Cd-Ros87 binds DNA
Cd-Ros87 DNA binding activity has been tested by EMSA experiment in which the VirC oligonucleotide recognized by Zn-Ros87 is used as a probe (Fig. 8).49 As shown in Fig. 8, Cd-Ros87 (lane 2) is able to bind the same DNA sequence recognized by Zn-Ros87 (lane 1) while is not able to bind an oligonucleotide (NS) containing a different DNA sequence (lane 3). The slight shift differences between the two complexes (lane 1 and 2) could reflect a small difference in their hydrodynamic properties. All together these results confirm that Cd-Ros87 adopts the correct functional fold in the presence of Cd(II) that therefore induces only small local rearrangements in the structure.
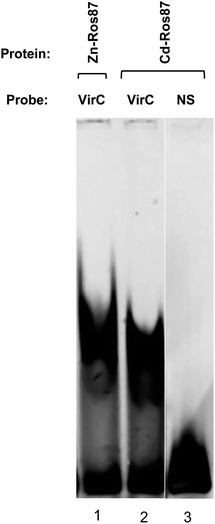 |
| Fig. 8 Cd-Ros87 DNA binding capability was tested using the specific target oligonucleotide VirC (lane 2) and a non specific oligonucleotide (NS – lane 3). Zn-Ros87 was also used as a positive control (lane 1). | |
Cd-Ros87 produced in E. coli cells (Cd-Ros87cell)
Cadmium is generally toxic to E. coli cells.63 Because of its toxicity, initially we tested the effect of Cd(II) both on the cell growth and on their protein synthesis level by exposing the cells to increasing concentrations of CdCl2. As shown in Fig. 9, the protein synthesis level remained unaffected up to a Cd(II) concentration of 0.6 mM. We found that 0.6 mM CdCl2 concentration is not lethal to BL21DE3 E. coli cells and after an initial 3 h bacteriostatic effect cells continue to grow (data not shown).
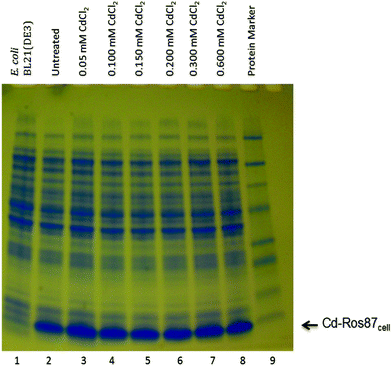 |
| Fig. 9 Effect of Cd(II) on the Ros87 synthesis in E. coli cells Cd(II)-treated and untreated for different doses in culture conditions. Ros87 synthesis was tested by SDS-PAGE analysis of total proteins at different doses of CdCl2 exposure (0.05 mM, lane 3; 0.100 mM, lane 4; 0.150 mM, lane 5; 0.200 mM, lane 6; 0.300 mM, lane 7 and 0.600 mM, lane 8) and compared with untreated cells as the control (lane 2). Lane 1, total proteins of E. coli BL21(DE3) host strain; lane 9, protein marker. | |
The 15N-labeled Ros87 protein produced in the presence of 0.6 mM of Cd(II) (Cd-Ros87cell) was characterized via ICP-MS and NMR. The ICP-MS analysis confirmed that ∼95% of the protein produced in these conditions contained cadmium, while the remaining part retains zinc.
The Cd-Ros87cell1H–15N-HSQC spectrum is reported in Fig. 10A. The pattern of the cross-peaks in the Cd-Ros87cell spectrum is quite different from the spectrum of Zn-Ros87 while closely resembles that obtained for the protein that we have produced in vitro by adding Cd(II) to apoRos87. The presence of Cd(II) in the protein Cd-Ros87cell is further confirmed by the superposition of the 1H–15N-HSQC-J18 spectra of Cd-Ros87 (blue signals) and Cd-Ros87cell (green signals) shown on Fig. 10B. The pattern of cross-peaks observed in the two spectra confirms that histidines 37 and 42 involved in the coordination of cadmium in Cd-Ros87 are still coordinating cadmium in Cd-Ros87cell protein and retain the same pattern of Nδ1–H tautomers.
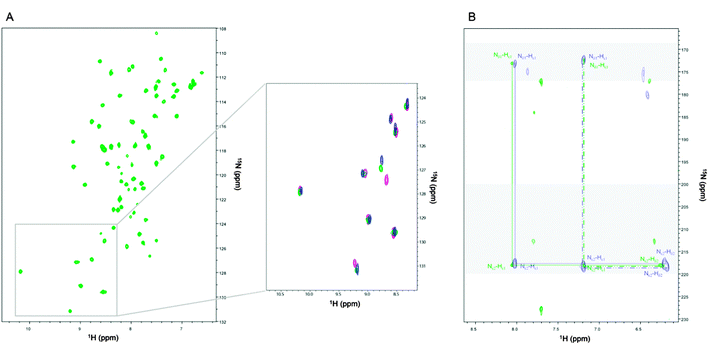 |
| Fig. 10 Panel A reports the 1H–15N HSQC spectrum of Cd-Ros87cell acquired at 298 K. In the inset: region of the spectrum reporting the superposition of Zn-Ros87 (pink), Cd-Ros87 (blue) and Cd-Ros87cell (green peaks). Panel B reports the superposition 1H–15N HSQC-J18 spectra of Cd-Ros87 (blue peaks) and Cd-Ros87cell (green peaks) acquired at 298 K. The gray rectangles indicate the nitrogen chemical-shift ranges typical for His involved in metal coordination. | |
The comparison of the Cd-Ros87cell1H–15N-HSQC spectrum with that of Cd-Ros87 (inset Fig. 10A – green and blue peaks) shows how the chemical shift of the residues that were found to be in slow exchange in the experiment of Cd(II) displacement by Zn(II) is exactly conserved (inset Fig. 10A – pink peaks). The resonances that in the displacement experiment were found in a fast exchange regime show a slight shift that is consistent with the ICP-MS results (Fig. 10A).
Overall, these data show how, during the protein synthesis in the presence of Cd(II) in E. coli, the cadmium ion is capable of substituting the zinc ion in the metal coordination sphere of Ros87.
4 Discussion
Mechanisms of cadmium toxicity are varied and comprehensive knowledge of cadmium biological effects is indeed necessary. Used in many technological applications, cadmium is naturally found in the environment as a mineral. Its industrial use has led to high emissions of cadmium in the environment, making the bio-available cadmium a risk for biological systems.64 Cadmium imitates other metals that are essential to biota, which generally fail to deal with this element.64 The only case of a biological function for cadmium has been recently described in the marine diatom Thalassiosira weissflogii, a unicellular microalga that grows in cadmium rich marine environments, in which cadmium is naturally associated with a zinc-binding carbonic anhydrase.65
Metal-binding proteins must bind their cognate metal ions with appropriate affinity to properly perform their biological function.
The evident similarity in the chemical properties of zinc and cadmium makes it possible for cadmium to replace the zinc ion at the binding sites of zinc finger proteins.35,38,64 The effects of such substitutions, frequently proposed as the leading molecular mechanism of cadmium toxicity, are likely to be fundamentally mediated by associated changes in the physicochemical properties of the proteins that are often reflected in the protein function.64 For these reasons the structural effects of zinc replacement by cadmium in different eukaryotic zinc fingers have been widely studied.15,16,18,21,23,38,39,66
The effects resulting from this substitution on the structure and function of eukaryotic Cys2His2 zinc fingers are dependent upon the specific domain sequence under consideration. In the recently reported structure of Cd(II)-SUP37, the overall domain fold is maintained when Zn(II) is substituted by Cd(II), but changes in the orientation of specific side chains most likely involved in the interaction with DNA are observed, suggesting a decreased binding affinity for its target DNA.13 In the case of Sp1, such substitution appears not to alter the quantitative binding to its cognate DNA and not to perturb its structure,23 while, in the case of Cd-tramtrack a 10-fold decrease in binding affinity for its DNA target and a loss of helical character is observed.67 Finally, Cd(II)-TFIIIA does not specifically bind its cognate DNA.18,21
In the present study, we report the effects of zinc to cadmium substitution on the structure and function of the prokaryotic Cys2His2 zinc-finger domain, included in the DNA binding region (Ros87) of Ros protein from A. tumefaciens.49 Though possessing a similar zinc coordination sphere, this domain is very different from its eukaryotic counterpart. It consists of 58 amino acids arranged in a βββαα topology and forming a globular fold stabilized by a 15 residues hydrophobic core.50
The dispersion of the amide chemical shifts in the 1H–15N-HSQC spectra clearly indicate that, as for Zn(II)-Ros87, Cd(II)-Ros87 populates a highly folded conformation with well-formed α-helix and β-strands structures. In addition, a large fraction of the resonances in the spectra of both zinc bound and cadmium bound proteins are superimposable. Because chemical shifts are extremely sensitive to changes in local environment, this is a strong indication that the two proteins adopt in solution a highly similar structure. Nonetheless, some chemical shift perturbations are evident that reflect a change in the local environment of the corresponding residues. These perturbations can be due to changes in the conformation of either the corresponding residues and/or of spatially proximal residues. The regions experiencing the greatest metal substitution induced perturbations are the β-turn connecting β2 and β3 strands, the loop that connects β3 and the first α-helix (α-1), α-1 and the residues 51, 53 and 57. These regions are all in the spatial vicinity of the metal binding site.50 Interestingly, the turn connecting the β-sheet with the α-helix and the first part of the α-helix have been reported to be the most perturbed regions also in the case of the eukaryotic SUPERMAN zinc finger (Cd(II)-SUP37).13 In that case such structural perturbations caused a global rearrangement of the ββα fold suggesting a detrimental influence on the proper functions of the domain.13 In the Ros87 case, cadmium can functionally substitute zinc as the protein is not influenced in its DNA binding activity. The domain appears to tolerate the metal substitution. Considering that cadmium has a slightly larger ionic radius than zinc, the possibility for it to substitute zinc without affecting the DNA binding capability demonstrates a structural ‘flexibility’ of this new DNA binding domain. Such flexibility is not surprising as a large number of Ros homologues has been found in different bacteria having high sequence identity with Ros protein that excludes the zinc coordination sphere: Ros homologues either show a modified coordination sphere or lose the structural metal nonetheless maintaining the DNA binding activity.68–70 Moreover, Ros87 contains four basic regions involved in DNA recognition, as previously shown. The first is located at the N-terminus (residues 14 and 15), the second is within the first helix (residue 35 and 36) and the other two are contained in the C-terminal disordered tail (residues 70 and 72 and residues 82–84). Overall, cadmium to zinc substitution affects the conformation of only one of the four basic regions,50 allowing the domain to preserve its DNA binding ability.
Substitution of native metals by cadmium is often proposed as a plausible molecular mechanism of cadmium toxicity.71,72 However the experimental evidence that such substitutions could take place in vivo is scarce. For this reason we have verified whether in the case of Ros87 such substitution would take place in a cellular context. We have shown how, given the evident similarity of the Cd(II)-Ros87 dissociation constant with respect to that for Zn2+, the same protein that we have produced in vitro by adding Cd(II) to apoRos87 is obtained in a cellular context by producing the protein in cells exposed to 0.6 mM CdCl2 concentration.
Accordingly, the 1H–15N-HSQC spectrum of the protein produced in E. coli cells treated with Cd(II) is consistent with the presence in solution of 95% Cd-Ros87 and 5% Zn-Ros87 proved via ICP-MS. In fact, the chemical shifts of those resonances that in Cd-Ros87 were found to be in a slow exchange regime in the experiment of Cd(II) displacement by Zn(II) are conserved in Cd-Ros87cell while those found in a fast exchange regime show in Cd-Ros87cell only a slight shift (Fig. 10A).
In eukaryota, proteins containing smaller zinc finger domains are almost always constituted by tandem of at least two successive motifs, whose structure and functions are entirely influenced by the presence and the nature of the metal ion. In prokaryotic proteins containing larger and globular zinc finger domains, mostly single and flanked by several basic regions, the domains appear to be functionally influenced to a lesser extent by the zinc availability and possibly by its substitution with similar metal ions.
Despite the nature of this prokaryotic zinc finger with its globular fold and the fact that an exogenous protein is over-produced in a host prokaryotic organism, a general conclusion can be drawn. The ability of this metal to substitute zinc in this DNA binding domain also in a cellular context suggests that metal substitution in zinc fingers in vivo may be of relevance to the toxicity and/or carcinogenicity of this metal. Future work should be aimed at properly addressing the significance of this mechanism.
Acknowledgements
The authors thank Dr Emilio Cuoco for assistance with the ICP-MS measurements, Mr Maurizio Muselli and Mr Marco Mammucari for the excellent technical assistance. This work was supported by Ministero dell' Istruzione Università e Ricerca (200993WWF9_001 to C.I. and 2010M2JARJ_002 to R.F.) and Programma MERIT RBNE08HWLZ_014 from Ministero dell' Istruzione, dell'Università e della Ricerca (MIUR).
References
- H. J. Coyne, S. Ciofi-Baffoni, L. Banci, I. Bertini, L. Zhang, G. N. George and D. R. Winge, J. Biol. Chem., 2007, 282, 8926–8934 CrossRef CAS PubMed.
- M. Ikeguchi, K. Kuwajima and S. Sugai, J. Biochem., 1986, 99, 1191–1201 CAS.
- P. Wittung-Stafshede, Acc. Chem. Res., 2002, 35, 201–208 CrossRef CAS PubMed.
- S. Chang, X. Jiao, J. P. Hu, Y. Chen and X. H. Tian, Int. J. Mol. Sci., 2010, 11, 4014–4034 CrossRef CAS PubMed.
- W. Li, J. Zhang, J. Wang and W. Wang, J. Am. Chem. Soc., 2008, 130, 892–900 CrossRef CAS PubMed.
- T. Miura, T. Satoh and H. Takeuchi, Biochim. Biophys. Acta, 1998, 1384, 171–179 CrossRef CAS.
- J. M. Berg and H. A. Godwin, Annu. Rev. Biophys. Biomol. Struct., 1997, 26, 357–371 CrossRef CAS PubMed.
- G. S. Makowski and F. W. Sunderman, J. Inorg. Biochem., 1992, 48, 107–119 CrossRef CAS.
- M. R. Basha, W. Wei, M. Brydie, M. Razmiafshari and N. H. Zawia, Int. J. Dev. Neurosci., 2003, 21, 1–12 CrossRef CAS.
- N. H. Zawia, T. Crumpton, M. Brydie, G. R. Reddy and M. Razmiafshari, Neurotoxicology, 2000, 21, 1069–1080 CAS.
- A. N. Besold, S. J. Lee, S. L. Michel, N. L. Sue and H. J. Cymet, J. Biol. Inorg. Chem., 2010, 15, 583–590 CrossRef CAS PubMed.
- S. J. Lee and S. L. Michel, Inorg. Chem., 2010, 49, 1211–1219 CrossRef CAS PubMed.
- G. Malgieri, L. Zaccaro, M. Leone, E. Bucci, S. Esposito, I. Baglivo, A. Del Gatto, L. Russo, R. Scandurra, P. V. Pedone, R. Fattorusso and C. Isernia, Biopolymers, 2011, 95, 801–810 CAS.
- R. C. diTargiani, S. J. Lee, S. Wassink and S. L. Michel, Biochemistry, 2006, 45, 13641–13649 CrossRef CAS PubMed.
- P. F. Predki and B. Sarkar, J. Biol. Chem., 1992, 267, 5842–5846 CAS.
- B. Sarkar, Nutrition, 1995, 11, 646–649 CAS.
- J. S. Hanas and C. G. Gunn, Nucleic Acids Res., 1996, 24, 924–930 CrossRef CAS PubMed.
- D. H. Petering, M. Huang, S. Moteki and C. F. Shaw, Mar. Environ. Res., 2000, 50, 89–92 CrossRef CAS.
- M. Razmiafshari, J. Kao, A. d'Avignon and N. H. Zawia, Toxicol. Appl. Pharmacol., 2001, 172, 1–10 CrossRef CAS PubMed.
- A. Hartwig, M. Asmuss, H. Blessing, S. Hoffmann, G. Jahnke, S. Khandelwal, A. Pelzer and A. Bürkle, Food Chem. Toxicol., 2002, 40, 1179–1184 CrossRef CAS.
- M. Huang, D. Krepkiy, W. Hu and D. H. Petering, J. Inorg. Biochem., 2004, 98, 775–785 CrossRef CAS PubMed.
- M. Matzapetakis, D. Ghosh, T. C. Weng, J. E. Penner-Hahn and V. L. Pecoraro, J. Biol. Inorg. Chem., 2006, 11, 876–890 CrossRef CAS PubMed.
- R. Kothinti, A. Blodgett, N. M. Tabatabai and D. H. Petering, Chem. Res. Toxicol., 2010, 23, 405–412 CrossRef CAS PubMed.
- A. Hartwig, T. Schwerdtle and W. Bal, Methods Mol. Biol., 2010, 649, 399–410 CAS.
- S. M. Quintal, Q. A. dePaula and N. P. Farrell, Metallomics, 2011, 3, 121–139 RSC.
- A. Witkiewicz-Kucharczyk and W. Bal, Toxicol. Lett., 2006, 162, 29–42 CrossRef CAS PubMed.
- A. B. Ghering, L. M. Jenkins, B. L. Schenck, S. Deo, R. A. Mayer, M. J. Pikaart, J. G. Omichinski and H. A. Godwin, J. Am. Chem. Soc., 2005, 127, 3751–3759 CrossRef CAS PubMed.
- A. Hartwig, BioMetals, 2010, 23, 951–960 CrossRef CAS PubMed.
- F. Thévenod, BioMetals, 2010, 23, 857–875 CrossRef PubMed.
- D. Beyersmann and A. Hartwig, Arch. Toxicol., 2008, 82, 493–512 CrossRef CAS PubMed.
- V. H. Liao and J. H. Freedman, J. Biol. Chem., 1998, 273, 31962–31970 CrossRef CAS PubMed.
- M. Waisberg, P. Joseph, B. Hale and D. Beyersmann, Toxicology, 2003, 192, 95–117 CrossRef CAS.
- J. M. Moulis, BioMetals, 2010, 23, 877–896 CrossRef CAS PubMed.
- M. Sugiyama, Cell Biol. Toxicol., 1994, 10, 1–22 CrossRef CAS.
- A. Hartwig and T. Schwerdtle, Toxicol. Lett., 2002, 127, 47–54 CrossRef CAS.
- T. Schwerdtle, F. Ebert, C. Thuy, C. Richter, L. H. Mullenders and A. Hartwig, Chem. Res. Toxicol., 2010, 23, 432–442 CrossRef CAS PubMed.
- T. S. Nawrot, J. A. Staessen, H. A. Roels, E. Munters, A. Cuypers, T. Richart, A. Ruttens, K. Smeets, H. Clijsters and J. Vangronsveld, BioMetals, 2010, 23, 769–782 CrossRef CAS PubMed.
- A. Hartwig, Antioxid. Redox Signaling, 2001, 3, 625–634 CrossRef CAS PubMed.
- D. Krepkiy, F. H. Försterling and D. H. Petering, Chem. Res. Toxicol., 2004, 17, 863–870 CrossRef CAS PubMed.
- S. Silver and L. T. Phung, Annu. Rev. Microbiol., 1996, 50, 753–789 CrossRef CAS PubMed.
- S. Silver, Gene, 1996, 179, 9–19 CrossRef CAS.
- S. Silver, J. Ind. Microbiol. Biotechnol., 1998, 20, 1–12 CrossRef CAS.
- M. R. Bruins, S. Kapil and F. W. Oehme, Ecotoxicol. Environ. Saf, 2000, 45, 198–207 CrossRef CAS PubMed.
- D. H. Nies and S. Silver, J. Ind. Microbiol., 1995, 14, 186–199 CrossRef CAS.
- T. Barkay and B. H. Olson, Appl. Environ. Microbiol., 1986, 52, 403–406 CAS.
- J. S. Parkinson and E. C. Kofoid, Annu. Rev. Genet., 1992, 26, 71–112 CrossRef CAS PubMed.
- L. Diels, Q. Dong, D. van der Lelie, W. Baeyens and M. Mergeay, J. Ind. Microbiol., 1995, 14, 142–153 CrossRef CAS.
- A. Y. Chou, J. Archdeacon and C. I. Kado, Proc. Natl. Acad. Sci. U. S. A., 1998, 95, 5293–5298 CrossRef CAS.
- S. Esposito, I. Baglivo, G. Malgieri, L. Russo, L. Zaccaro, L. D. D'Andrea, M. Mammucari, B. Di Blasio, C. Isernia, R. Fattorusso and P. V. Pedone, Biochemistry, 2006, 45, 10394–10405 CrossRef CAS PubMed.
- G. Malgieri, L. Russo, S. Esposito, I. Baglivo, L. Zaccaro, E. M. Pedone, B. Di Blasio, C. Isernia, P. V. Pedone and R. Fattorusso, Proc. Natl. Acad. Sci. U. S. A., 2007, 104, 17341–17346 CrossRef CAS PubMed.
- M. Palmieri, G. Malgieri, L. Russo, I. Baglivo, S. Esposito, F. Netti, A. Del Gatto, I. de Paola, L. Zaccaro, P. V. Pedone, C. Isernia, D. Milardi and R. Fattorusso, J. Am. Chem. Soc., 2013, 135, 5220–5228 CrossRef CAS PubMed.
- A. Nomura and Y. Sugiura, Inorg. Chem., 2002, 41, 3693–3698 CrossRef CAS PubMed.
- C. Isernia, E. Bucci, M. Leone, L. Zaccaro, P. Di Lello, G. Digilio, S. Esposito, M. Saviano, B. Di Blasio, C. Pedone, P. V. Pedone and R. Fattorusso, ChemBioChem, 2003, 4, 171–180 CrossRef CAS PubMed.
- C. Bartels, T. H. Xia, M. Billeter, P. Güntert and K. Wüthrich, J. Biomol. NMR, 1995, 6, 1–10 CrossRef CAS PubMed.
- R. Koradi, M. Billeter and K. Wüthrich, J. Mol. Graph, 1996, 14, 51–55 CrossRef CAS , 29–32.
- M. P. Foster, D. S. Wuttke, K. R. Clemens, W. Jahnke, I. Radhakrishnan, L. Tennant, M. Reymond, J. Chung and P. E. Wright, J. Biomol. NMR, 1998, 12, 51–71 CrossRef CAS.
- S. Grzesiek, A. Bax, G. M. Clore, A. M. Gronenborn, J. S. Hu, J. Kaufman, I. Palmer, S. J. Stahl and P. T. Wingfield, Nat. Struct. Biol., 1996, 3, 340–345 CrossRef CAS PubMed.
- D. S. Garrett, Y. J. Seok, A. Peterkofsky, G. M. Clore and A. M. Gronenborn, Biochemistry, 1997, 36, 4393–4398 CrossRef CAS PubMed.
- M. Palmieri, L. Russo, G. Malgieri, S. Esposito, I. Baglivo, A. Rivellino, B. M. Farina, I. de Paola, L. Zaccaro, D. Milardi, C. Isernia, P. V. Pedone and R. Fattorusso, J. Inorg. Biochem., 2014, 131, 30–36 CrossRef PubMed.
- R. J. Simpson, E. D. Cram, R. Czolij, J. M. Matthews, M. Crossley and J. P. Mackay, J. Biol. Chem., 2003, 278, 28011–28018 CrossRef CAS PubMed.
- A. Urbani, R. Bazzo, M. C. Nardi, D. O. Cicero, R. De Francesco, C. Steinkühler and G. Barbato, J. Biol. Chem., 1998, 273, 18760–18769 CrossRef CAS PubMed.
- K. Hirose, J. Inclusion Phenom. Macrocyclic Chem., 2001, 39, 193–209 CrossRef CAS.
- S. T. Hossain, I. Mallick and S. K. Mukherjee, Ecotoxicol. Environ. Saf, 2012, 86, 54–59 CrossRef CAS PubMed.
- A. Martelli, E. Rousselet, C. Dycke, A. Bouron and J. M. Moulis, Biochimie, 2006, 88, 1807–1814 CrossRef CAS PubMed.
- T. W. Lane, M. A. Saito, G. N. George, I. J. Pickering, R. C. Prince and F. M. Morel, Nature, 2005, 435, 42 CrossRef CAS PubMed.
- R. K. Kothinti, A. B. Blodgett, D. H. Petering and N. M. Tabatabai, Toxicol. Appl. Pharmacol., 2010, 244, 254–262 CrossRef CAS PubMed.
- G. Roesijadi, R. Bogumil, M. Vasák and J. H. Kägi, J. Biol. Chem., 1998, 273, 17425–17432 CrossRef CAS PubMed.
- I. Baglivo, L. Russo, S. Esposito, G. Malgieri, M. Renda, A. Salluzzo, B. Di Blasio, C. Isernia, R. Fattorusso and P. V. Pedone, Proc. Natl. Acad. Sci. U. S. A., 2009, 106, 6933–6938 CrossRef CAS PubMed.
- F. Netti, G. Malgieri, S. Esposito, M. Palmieri, I. Baglivo, C. Isernia, J. G. Omichinski, P. V. Pedone, N. Lartillot and R. Fattorusso, Mol. Biol. Evol., 2013, 30, 1504–1513 CrossRef CAS PubMed.
- L. Russo, M. Palmieri, I. Baglivo, S. Esposito, C. Isernia, G. Malgieri, P. V. Pedone and R. Fattorusso, Biomol. NMR Assignments, 2010, 4, 55–57 CrossRef CAS PubMed.
- D. K. Blencowe and A. P. Morby, FEMS Microbiol. Rev., 2003, 27, 291–311 CrossRef CAS.
- A. P. Marques, H. Moreira, A. R. Franco, A. O. Rangel and P. M. Castro, Chemosphere, 2013, 92, 74–83 CrossRef CAS PubMed.
|
This journal is © The Royal Society of Chemistry 2014 |
Click here to see how this site uses Cookies. View our privacy policy here.