DOI:
10.1039/C3NR04425D
(Paper)
Nanoscale, 2014,
6, 255-262
Multi-enzyme co-embedded organic–inorganic hybrid nanoflowers: synthesis and application as a colorimetric sensor†
Received
20th August 2013
, Accepted 30th September 2013
First published on 3rd October 2013
Abstract
This study reports a facile method for the synthesis of multi-enzyme co-embedded organic–inorganic hybrid nanoflowers, using glucose oxidase (GOx) and horseradish peroxidase (HRP) as the organic components, and Cu3(PO4)2·3H2O as the inorganic component. The synthesized nanoflowers enable the combination of a two-enzyme cascade reaction in one step, in which the GOx component of the nanoflowers oxidizes glucose to generate H2O2, which then reacts with the adjacent HRP component on the nanoflowers to oxidize the chromogenic substrates, resulting in an apparent color change. Given the close proximity of the two enzyme components in a single nanoflower, this novel sensor greatly reduces the diffusion and decomposition of H2O2, and greatly enhances the sensitivity of glucose detection. Thus, the obtained multi-enzyme co-embedded organic–inorganic hybrid nanoflowers can be unquestionably used as highly sensitive colorimetric sensors for the detection of glucose. Notably, this work presents a very facile route for the synthesis of multi-enzyme co-embedded nanomaterials for the simultaneous catalysis of multi-step cascade enzymatic reactions. Furthermore, it has great potential for application in biotechnology, and biomedical and environmental chemistry.
1. Introduction
The concept of enzyme-embedded nanomaterials, in which enzymes are immobilized in nanostructured materials, is a recent but very fruitful and prolific area of research, as it has been applied to proteomic analysis, antifouling, biofuel cells, and tissue engineering, due to the improved stability of the immobilized enzymes.1–3 Given that a number of multi-step reactions or cascade processes need to be catalyzed by a number of enzymes, multi-enzyme co-embedded nanomaterials have recently received continuous interest,4–8 in which multiple enzymes catalyzing multi-step or cascade reactions are co-immobilized into the same support, such as a sol–gel,9,10 silica particles,11 a polymer,12 DNA13–16 or a protein.17,18 Compared with single-enzyme immobilization, these multi-enzyme co-embedded nanomaterials enable the combination of cascade reactions in one pot, either by running the cascade reactions simultaneously or in a stepwise fashion without isolating the intermediates.7 The major advantage of multi-enzyme co-immobilization is that the active sites of the enzymes are brought into close proximity with one another in the nanomaterials, and such close confinement minimizes the diffusion of intermediates among the enzymes in the consecutive reactions, thereby enhancing the overall reaction efficiency and specificity.6 To date, most multi-enzyme co-immobilizations are achieved through relatively complicated procedures, such as covalent cross-linking,19 encapsulation,20 gene fusion21 and post-translational enzyme conjugation.22 Unfortunately, these methods are either time consuming or may reduce enzymatic activity, which limits the application of these materials.6 Therefore, the development of a facile and efficient method to create multi-enzyme co-embedded nanomaterials without negatively affecting the activity of the enzymes is urgently needed.
Colorimetric sensors have been used to detect a variety of targets including nucleic acids, proteins, small molecules, metal ions, and especially for the detection of glucose, due to their low-cost, simplicity, and ease of measurement with the naked eye.23–30 Conventional colorimetric sensors for the detection of glucose are operated in two separate steps. In the first step, glucose is incubated with glucose oxidase (GOx) which oxidizes glucose to produce hydrogen peroxide (H2O2). In the second step, the as-prepared reaction solution containing H2O2 then reacts with horseradish peroxidase (HRP), or its mimics, and a chromogenic substrate, resulting in a colorimetric change of the solution (Fig. 1a).31–37 However, due to its instability, a large amount of H2O2 can decompose in this two-step enzymatic reaction, resulting in the loss of sensitivity of the assay. Recently, Zare's group reported a method for the creation of hybrid protein–inorganic nanoflowers using various proteins and Cu3(PO4)2·3H2O,38 which were then used to achieve a new and rapid detection of aqueous phenol solutions.39 Herein, we further develop this method and report a very simple and convenient method for the synthesis of new multi-enzyme co-embedded organic–inorganic hybrid nanostructures, GOx&HRP–Cu3(PO4)2·3H2O nanoflowers, by immobilizing GOx and HRP in a one-pot reaction, and subsequently applying the resulting nanoflowers as a colorimetric sensor. Since GOx and HRP are co-immobilized in single GOx&HRP–Cu3(PO4)2·3H2O nanoflowers, the two-step enzymatic assay for glucose detection can be achieved in a single hybrid-enzyme nanoflower. As shown in Scheme 1b, when glucose is added into the system, it reacts with O2 and GOx on the GOx&HRP–Cu3(PO4)2·3H2O nanoflowers to produce H2O2, which is immediately catalyzed by adjacent HRP on the same nanoflower to oxidize the 3,3′,5,5′-tetramethylbenzidine (TMB) in the solution, resulting in a color change from clear to blue. Due to the close proximity of the GOx and the HRP in the GOx&HRP–Cu3(PO4)2·3H2O nanoflowers, the developed method utilizes the synergistic behaviors of GOx and HRP, and reduces the diffusion and decomposition of H2O2. The results suggested that the GOx&HRP–Cu3(PO4)2·3H2O nanoflowers cascade reaction displayed both higher response and sensitivity for the detection of glucose than the corresponding two-step free enzyme assay (Table S1†).
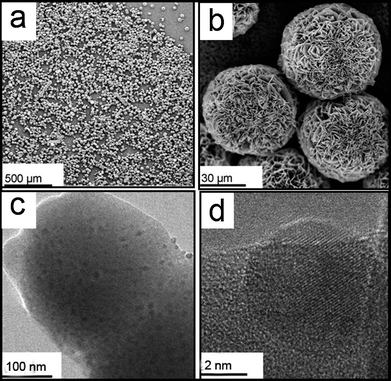 |
| Fig. 1 (a and b) SEM images of GOx&HRP–Cu3(PO4)2·3H2O nanoflowers. (c) TEM image of GOx&HRP–Cu3(PO4)2·3H2O nanoflowers. (d) High-resolution TEM image of GOx&HRP–Cu3(PO4)2·3H2O nanoflowers. | |
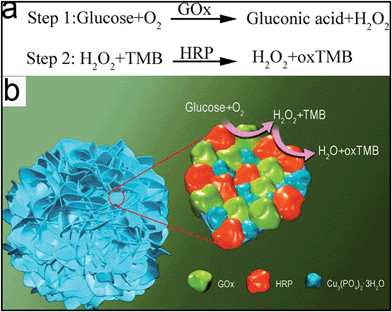 |
| Scheme 1 (a) The mechanism of glucose detection using a two-step enzymatic reaction. (b) The mechanism of glucose detection using GOx&HRP–Cu3(PO4)2·3H2O nanoflowers. | |
2. Experimental
2.1 Materials and methods
Glucose oxidase (GOx), horseradish peroxidase (HRP), and β-D-glucose were purchased from Sigma Aldrich. CuSO4 was purchased from Alfa Aesar. PBS buffer was purchased from Hyclone. All stock solutions were prepared with MilliQ-purified distilled water. White colored sponge was purchased from a supermarket.
The size and the morphology of the nanoflowers were examined using a JEOL JEM-2100F Transmission Electron Microscope (TEM) and a Hitachi S-4300 Scanning Electron Microscope (SEM). For SEM imaging, a suspension of the nanoflowers was filtered and dried on a membrane (pore size: 0.1 μm) and sputter coated with gold. For TEM imaging, a 9 μL suspension of the nanoflowers was added to the TEM grid and dried at room temperature. UV-Vis spectra were recorded on a Hitachi U-3010 UV/Vis spectrophotometer. XRD patterns were obtained using an X-ray diffractometer (Bruker, Germany) using Cu-Kα radiation (λ = 1.5178 Å, 40 kV × 40 mA). The 2θ scanning range was from 5° to 80° with a scanning speed of 0.1° s−1. Photographs of color changes were taken with a Sony digital camera.
2.2 Preparation and characterization of GOx–Cu3(PO4)2·3H2O nanoflowers, HRP–Cu3(PO4)2·3H2O nanoflowers and GOx&HRP–Cu3(PO4)2·3H2O nanoflowers
Synthesis of GOx–Cu3(PO4)2·3H2O nanoflowers: in a typical experiment, 20 μL of aqueous CuSO4 solution (120 mM) in molecular biology grade water was added to 3 mL of PBS (pH = 7.4) containing different concentrations of GOx, followed by incubation at 25 °C for 3 days.
Synthesis of HRP–Cu3(PO4)2·3H2O nanoflowers: in a typical experiment, 20 μL of aqueous CuSO4 solution (120 mM) in molecular biology grade water was added to 3 mL of PBS (pH = 7.4) containing different concentrations of HRP, followed by incubation at 25 °C for 3 days.
Synthesis of GOx&HRP–Cu3(PO4)2·3H2O nanoflowers: in a typical experiment, 20 μL of aqueous CuSO4 solution (120 mM) in molecular biology grade water were added to 3 mL of PBS (pH = 7.4) containing different concentrations of GOx and HRP.
2.3 Treatments of GOx&HRP–Cu3(PO4)2·3H2O nanoflowers
The suspension of protein-incorporated nanoflowers was incubated with 25 μg mL−1 of trypsin at 25 °C for 12 h.
The protein-incorporated nanoflowers were treated with glutaraldehyde (0.8 wt%) in solution for 48 hours, followed by incubation with EDTA (1 wt%).
For calcination, the suspension of protein-incorporated nanoflowers was dried on a silicon wafer and heated at 350 °C for 2 hours.
2.4 Glucose detection with GOx&HRP–Cu3(PO4)2·3H2O nanoflowers
Glucose detection with GOx&HRP–Cu3(PO4)2·3H2O nanoflowers was performed as follows: different concentrations of glucose were added into 2 mL 0.2 M HAc–NaAc buffer (pH = 4.0) containing 2 mg GOx&HRP–Cu3(PO4)2·3H2O nanoflowers and 0.5 mM TMB, which was then incubated at 37 °C for 25 min. Then, the reaction solution was centrifuged at 10
000 rpm for 10 min to remove the GOx&HRP–Cu3(PO4)2·3H2O nanoflowers and the absorbance of the solution was measured.
2.5 Glucose detection with free enzyme, HRP–Cu3(PO4)2·3H2O nanoflowers, GOx–Cu3(PO4)2·3H2O nanoflowers, and a mixture of HRP–Cu3(PO4)2·3H2O nanoflowers and GOx–Cu3(PO4)2·3H2O nanoflowers
Glucose detection with free enzyme was performed as follows: (a) different concentrations of glucose were added to 2 mL PBS (pH = 7.4) solution containing 0.5 mg mL−1 GOx, and the solution was then incubated at 37 °C for 25 min. (b) 100 μL of 10 mM TMB, 20 μL of 10 μg mL−1 HRP, and 1.72 mL of 0.2 M HAc–NaAc buffer (pH = 4.0) were added to the above 160 μL glucose reaction solution. (c) The mixed solution was incubated at 37 °C for 20 min and then kept in an ice-water bath for 10 min to terminate the reaction. (d) The absorbance of the final solution was then measured.
Glucose detection with HRP–Cu3(PO4)2·3H2O nanoflowers was performed as follows: (a) glucose was added to 2 mL PBS (pH = 7.4) solution containing 0.5 mg mL−1 GOx, and the solution was then incubated at 37 °C for 25 min. (b) 100 μL of 10 mM TMB, 2 mg HRP–Cu3(PO4)2·3H2O nanoflowers and 1.72 mL of 0.2 M HAc–NaAc buffer (pH 4.0) were added to the above 160 μL glucose reaction solution. (c) The mixed solution was incubated at 37 °C for 20 min and then kept in an ice-water bath for 10 min to terminate the reaction. (d) The absorbance of the final solution was then measured.
Glucose detection with GOx–Cu3(PO4)2·3H2O nanoflowers was performed as follows: (a) different concentrations of glucose were added to 2 mL PBS (pH = 7.4) solution containing 2 mg GOx–Cu3(PO4)2·3H2O nanoflowers, and the solution was then incubated at 37 °C for 25 min. The reaction solution was centrifuged at 10
000 rpm for 10 min to remove the GOx–Cu3(PO4)2·3H2O nanoflowers. (b) 100 μL of 10 mM TMB, 20 μL of 10 μg mL−1 HRP, and 1.72 mL of 0.2 M HAc–NaAc buffer (pH = 4.0) were added to the above 160 μL glucose reaction solution. (c) The mixed solution was incubated at 37 °C for 20 min and then kept in an ice-water bath for 10 min to terminate the reaction. (d) The absorbance of the final solution was then measured.
Glucose detection with a mixture of HRP–Cu3(PO4)2·3H2O nanoflowers and GOx–Cu3(PO4)2·3H2O nanoflowers was performed as follows: different concentrations of glucose were added to 2 mL 0.2 M HAc–NaAc buffer (pH 4.0) containing 2 mg GOx–Cu3(PO4)2·3H2O, created from PBS solution containing 0.5 mg mL−1 GOx, 2 mg HRP–Cu3(PO4)2·3H2O nanoflowers, created from PBS solution containing 0.1 mg mL−1 HRP and 0.5 mM TMB, and the solution was then incubated at 37 °C for 25 min. Then, the reaction solution was centrifuged at 10
000 rpm for 10 min to remove the nanoflowers and the absorbance of the final solution was measured.
2.6 Paper-based glucose detection using sponge containing GOx&HRP–Cu3(PO4)2·3H2O nanoflowers as a model
Commercially obtained white colored sponge was cut into pieces with a hole-puncher. GOx&HRP–Cu3(PO4)2·3H2O nanoflowers suspended in PBS was injected into the sponge. For glucose detection, the GOx&HRP–Cu3(PO4)2·3H2O nanoflower containing sponge was immersed in an acetate solution containing 0–50 μM glucose and 0.5 mM TMB, and kept at 37 °C for 25 min.
2.7 Urine sample preparation
For the glucose determination of a urine sample from a male diabetic, the collected sample was stored at −20 °C and thawed at room temperature before further preparation. The sample was centrifuged at 12
000 rpm for 10 min, and then, the supernatant was directly used for measurement.
The detection of glucose in urine using GOx&HRP–Cu3(PO4)2·3H2O nanoflowers was performed as follows: 20 μL of as-prepared urine sample was added to 1.8 mL 0.2 M HAc–NaAc buffer (pH 4.0) containing 2 mg GOx&HRP–Cu3(PO4)2·3H2O nanoflowers and 0.5 mM TMB, and the solution was then incubated at 37 °C for 25 min. Then, the reaction solution was centrifuged at 10
000 rpm for 10 min to remove the GOx&HRP–Cu3(PO4)2·3H2O nanoflowers and the absorbance of the final solution was measured.
3. Results and discussion
3.1 Structural characterization
GOx&HRP–Cu3(PO4)2·3H2O nanoflowers were created by adding CuSO4 to phosphate buffered saline (PBS) solution containing GOx and HRP, at pH 7.4 and room temperature. After three days, a precipitate with porous, flower-like structures appeared. Fig. 1a and b show the general morphologies of the GOx&HRP–Cu3(PO4)2·3H2O nanoflowers as imaged by scanning electron microscopy (SEM). In the low-resolution SEM image, most of the GOx&HRP–Cu3(PO4)2·3H2O nanoflowers are uniform spheres with diameters of approximately 30 μM (Fig. 1a). The high-resolution SEM image of the GOx&HRP–Cu3(PO4)2·3H2O nanoflowers shows that they are assembled from hundreds of nanoplates (Fig. 1b). A transmission electron microscope (TEM) image of a single nanoplate is shown in Fig. 1c, and a high-resolution TEM image of the crystal structure of one of the nanoplates is presented in Fig. 1d. The X-ray diffraction pattern of the nanoflower powder fits that of Cu3(PO4)2·3H2O (Fig. S1†). The morphologies of nanoflowers produced from different PBS solutions containing different concentrations of enzymes were also studied. The results showed that the nanoflowers created from the PBS solution containing 0.5 mg mL−1 GOx were rigid and uniform. As the GOx concentration was decreased (0.1, 0.05, 0.01 mg mL−1), the obtained nanoflowers became larger and less uniform (Fig. S2†). This result may be due to the fact that decreasing the concentration of GOx would lead to a corresponding decrease in the number of nucleation sites, resulting in nanoflowers with a greater size and a less uniform structure. When nanoflowers were created from PBS containing 0.5 mg mL−1 GOx but decreasing concentrations of HRP (0.5, 0.1, 0.05, and 0.01 mg mL−1), nanoflowers with similar sizes were obtained (Fig. S3†).
3.2 Growth mechanism
To study the mechanism of formation of the multi-enzyme co-embedded organic–inorganic hybrid nanoflowers, SEM images were recorded as a function of nanoflower growth time. At an early growth stage (2 h, Step 1, Fig. 2b), primary crystals of copper phosphate were formed. At this stage, protein molecules formed complexes with Cu2+ predominantly through coordination of the amide groups in the protein backbone.40 These complexes then provided a location for nucleation of the primary crystals. In the second growth step (8 h to 36 h, Step 2, Fig. 2c–e), protein–Cu2+ crystals combined into large agglomerates that formed the primary petals. The kinetically controlled growth of copper phosphate crystals originated on the surfaces of these agglomerates, causing small petals to appear. In the last stage (48 h to 72 h, step 3, Fig. 2f and g), the growth process of the nanoflowers continued, resulting in the complete formation of multi-layered flower-like structures. In this growth process, the protein induces the nucleation of the copper phosphate crystals to form the scaffold for the petal, and also binds the petals together.23 In the absence of protein, large crystals without nanoflowers were formed (Fig. S4a†). Furthermore, the digestion of protein by trypsin led to the shrinking of the nanoflower structure (Fig. 2h). The loss of the flower structure was believed to have been caused by the removal of protein from the petal. The nanoflowers were further treated with glutaraldehyde, which crosslinked the protein, followed by the addition of ethylenediaminetetraacetic acid (EDTA) to remove the Cu2+. After this treatment, microspheres with an average size of 20 μm and relatively smooth surfaces were obtained (Fig. 2i), indicating that the protein was mainly located in the core of the nanoflowers. Calcination of the nanoflowers at 350 °C led to the loss of the flower structure (Fig. 2j). Furthermore, no nanoflowers were observed (Fig. S4b†) when 0.8 mM copper sulfate was added into the protein-containing phosphate buffer without chloride ions, suggesting that chloride ions are essential for nanoflower formation.
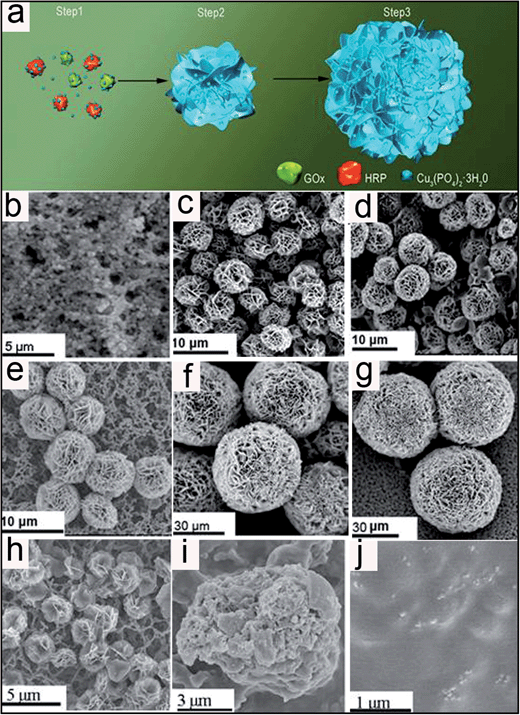 |
| Fig. 2 (a) Proposed growth mechanism of nanoflowers. (b–g) SEM images of GOx&HRP–Cu3(PO4)2·3H2O nanoflowers after growing for (b) 2 h, (c) 8 h, (d) 24 h, (e) 36 h, (f) 48 h, (g) 72 h. (h–j) SEM images of nanoflowers treated with (h) trypsin, (i) glutaraldehyde and EDTA and (j) calcination. | |
3.3 Performance of the glucose sensor
The performances of the GOx&HRP–Cu3(PO4)2·3H2O nanoflowers created from PBS solutions containing different concentrations of GOx and HRP were compared, in order to optimize the glucose detection sensitivity of the GOx&HRP–Cu3(PO4)2·3H2O nanoflowers. The GOx&HRP–Cu3(PO4)2·3H2O nanoflowers produced in a PBS solution containing 0.5 mg mL−1 GOx and 0.1 mg mL−1 HRP showed a higher sensitivity for glucose detection than other nanoflowers, as shown in Fig. S5.† To test the quantitative detection of glucose using the developed method, different concentrations of glucose were added to the acetate buffer (pH = 4.0) containing GOx&HRP–Cu3(PO4)2·3H2O nanoflowers and TMB at 37 °C. Upon increasing the concentration of glucose, the color of the reaction solution gradually changed from clear into dark blue (Fig. 3a). The reaction could be completed within 25 min under the employed conditions (Fig. S6†). These results were further confirmed by UV-Vis spectroscopy. An obvious increase in the absorption peak at 656 nm was clearly observed with increasing glucose concentration, as shown in Fig. 3b. Furthermore, the corresponding effect was evaluated by comparing the A656 values in the presence of different concentrations of glucose for quantitative analysis. A linear relationship was found between A650 and the glucose concentration in the range of 0–50 μM (R = 0.993) (Fig. 3d). The limit of detection (LOD) was 0.2 μM, which is lower than those of previously reported colorimetric glucose sensors31–37 (Table S1.† The LOD was calculated according to the method described by Demchemko, as illustrated in the ESI.†) To illustrate the potential application of GOx&HRP–Cu3(PO4)2·3H2O nanoflowers in glucose test paper, we injected GOx&HRP–Cu3(PO4)2·3H2O nanoflowers into commercially obtained white sponge, which was then immersed in acetate buffer containing TMB and glucose. The nanoflowers containing sponge showed an obvious color change in the presence of 0–50 μM glucose, as shown in Fig. 3c.
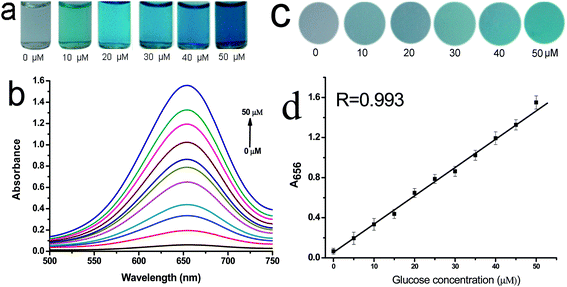 |
| Fig. 3 (a) Colorimetric change of TMB solutions containing GOx&HRP–Cu3(PO4)2·3H2O nanoflowers upon the addition of 0, 10, 20, 30, 40, and 50 μM glucose. (b) UV-Vis absorption spectra of TMB solutions containing GOx&HRP–Cu3(PO4)2·3H2O nanoflowers upon the addition of 0–50 μM glucose. (c) The color change of sponge containing GOx&HRP–Cu3(PO4)2·3H2O nanoflowers after immersion in the acetate buffer solution containing 0–50 μM glucose and 0.5 mM TMB. (d) The plot of A656versus glucose concentration. | |
Furthermore, GOx&HRP–Cu3(PO4)2·3H2O nanoflowers were used to detect the glucose in a urine sample from a male volunteer with diabetes. The urine sample was used in the detection without any complicated pretreatment. The reaction solution exhibited an obvious color change from clear to blue upon the addition of the urine sample (Fig. 4). The UV-Vis spectra indicated that this method is largely free from the complicated sample matrix effect of the urine sample. According to the calibration curve, the concentration of glucose in the urine was determined to be 3.9 mM (70.2 mg dL−1). The GOx&HRP–Cu3(PO4)2·3H2O nanoflowers could detect 0–5 mM glucose (0–90 mg dL−1) in the urine sample. Therefore, this colorimetric method is applicable for the determination of glucose concentration in urine samples.
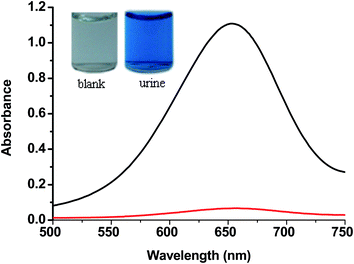 |
| Fig. 4 UV-Vis spectra of the blank solution (black line) and the solution with the urine sample (red line). Inset: visual color change between the blank solution and the solution with the urine sample. | |
To illustrate the advantage of GOx&HRP–Cu3(PO4)2·3H2O nanoflowers, we compared their performance to those of the GOx–Cu3(PO4)2·3H2O nanoflowers, HRP–Cu3(PO4)2·3H2O nanoflowers, and free enzymes. The result suggested that the response of the GOx&HRP–Cu3(PO4)2·3H2O nanoflowers with respect to glucose is about 400% higher than that of free enzymes, GOx–Cu3(PO4)2·3H2O nanoflowers, or HRP–Cu3(PO4)2·3H2O nanoflowers (Fig. 5a). Furthermore, the performance of the GOx&HRP–Cu3(PO4)2·3H2O nanoflowers was compared with that of a mixture of GOx–Cu3(PO4)2·3H2O nanoflowers and HRP–Cu3(PO4)2·3H2O nanoflowers with a mass ratio of 1
:
1, to prove the proposed mechanism of the enhanced performance of the GOx&HRP–Cu3(PO4)2·3H2O nanoflowers in the detection of glucose. In this detection system, given that GOx and HRP were immobilized on separate nanoflowers, glucose was oxidized by GOx in the GOx–Cu3(PO4)2·3H2O nanoflowers to generate H2O2, which then diffused to the HRP–Cu3(PO4)2·3H2O nanoflowers to react with HRP and TMB. The distance between the GOx and HRP was therefore farther than that in the GOx&HRP–Cu3(PO4)2·3H2O nanoflowers; thus it is supposed that the performance of the mixture of GOx–Cu3(PO4)2·3H2O nanoflowers and HRP–Cu3(PO4)2·3H2O nanoflowers should be weaker than that of the GOx&HRP–Cu3(PO4)2·3H2O nanoflowers. As shown in Fig. 5a, the GOx&HRP–Cu3(PO4)2·3H2O nanoflowers showed more than a 60% higher response to the presence of glucose than that of the mixture of GOx–Cu3(PO4)2·3H2O and HRP–Cu3(PO4)2·3H2O nanoflowers. These results suggest that the enhanced response of the GOx&HRP–Cu3(PO4)2·3H2O nanoflowers to glucose probably arises from the close proximity of the GOx and the HRP. In addition, the performances of the GOx&HRP–Cu3(PO4)2·3H2O nanoflowers and free enzymes were compared at different temperatures. GOx&HRP–Cu3(PO4)2·3H2O showed stable performance at temperatures as high as 65 °C, whereas free GOx lost a large part of its reactivity at temperatures higher than 45 °C, suggesting that the immobilization of the enzymes in the nanoflowers improved their stability (Fig. 5b).
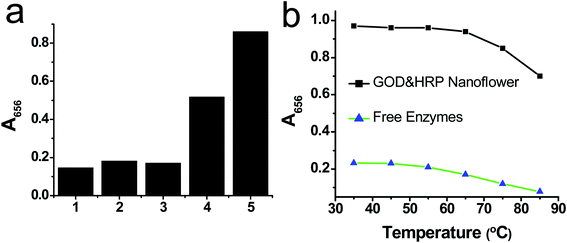 |
| Fig. 5 (a) The performance of (1) free enzymes, (2) GOx–Cu3(PO4)2·3H2O nanoflowers, (3) HRP–Cu3(PO4)2·3H2O nanoflowers, (4) the mixture of GOx–Cu3(PO4)2·3H2O nanoflowers and HRP–Cu3(PO4)2·3H2O nanoflowers, and (5) GOx&HRP nanoflowers in the presence of 30 μM glucose. (b) The performance of GOx&HRP nanoflowers (black points) and free enzymes (blue points) at different temperatures. | |
The selectivity of the GOx&HRP–Cu3(PO4)2·3H2O nanoflowers for glucose was evaluated by monitoring the absorbance change at 656 nm (A656) in the presence of various analytes. The results are shown in Fig. 6. First, the responses of GOx&HRP–Cu3(PO4)2·3H2O nanoflowers to ions such as NaCl, KCl, and CaCl2 were investigated. The absorbance at 656 nm (A656) showed minimal change in the presence of NaCl, KCl, and CaCl2. Thus, GOx&HRP–Cu3(PO4)2·3H2O nanoflowers showed no cross-reactivity with these ions. Subsequently, the response of GOx&HRP–Cu3(PO4)2·3H2O nanoflowers to large biological molecules such as BSA and DNA was evaluated. The results indicated that GOx&HRP–Cu3(PO4)2·3H2O nanoflowers displayed little response to the presence of either BSA or DNA in comparison with glucose. We further monitored the response of GOx&HRP–Cu3(PO4)2·3H2O nanoflowers to other saccharides such as fructose, mannose, maltose, and galactose. The UV-Vis spectra revealed that these molecules did not interfere in the detection of glucose. The selectivity of GOx&HRP–Cu3(PO4)2·3H2O nanoflowers to glucose can be attributed to the high selectivity of GOx to glucose.
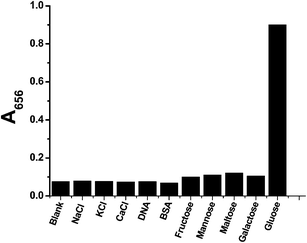 |
| Fig. 6 The selectivity of GOx&HRP–Cu3(PO4)2·3H2O nanoflowers for 30 μM glucose in comparison to 30 μM NaCl, KCl, CaCl2, fructose, mannose, maltose, galactose, and 1 μg L−1 DNA and BSA. | |
4. Conclusions
We have reported a facile method for the synthesis of multi-enzyme co-embedded organic–inorganic hybrid nanoflowers, by simultaneously using GOx and HRP as the organic components and copper(II) ions as the inorganic component. The newly synthesized nanostructures can catalyze the two-enzyme cascade reaction in one step, in which the GOx component of the nanoflowers oxidises glucose to form H2O2, which then reacts with the adjacent HRP component on the nanoflowers to oxidize TMB, resulting in a colorimetric change. Given the close proximity of the two enzyme components in a single nanoflower, this method largely reduces the diffusion and decomposition of H2O2, thereby greatly enhancing the sensitivity of glucose detection. Given the wide variety of possible enzyme combinations and the efficiency of this method, we believe that this work presents a new route for the synthesis of multi-enzyme bionanocatalysts for the simultaneous catalysis of multi-step cascade enzymatic reactions, and furthermore, it has great potential for application in biotechnology, and biomedical and environmental chemistry.
Acknowledgements
This work was supported by the National Basic Research Program of China (Grant no. 2012CB932401) and the NNSF of China (Grant no. 51172244, 11179006 and 61227008).
References and Notes
- C. Y. Chiu, Y. J. Li, L. Y. Ruan, X. C. Ye, C. B. Murray and Y. Huang, Nat. Chem., 2011, 3, 393–399 CrossRef CAS PubMed
.
- S. Mann, Nat. Mater., 2009, 8, 781–792 CrossRef CAS PubMed
.
- L. B. Wang, Y. C. Wang, R. He, A. Zhuang, X. P. Wang, J. Zeng and J. G. Hou, J. Am. Chem. Soc., 2013, 135, 1272–1275 CrossRef CAS PubMed
.
- K. Tauber, M. Fuchs, J. H. Sattler, J. Pitzer, D. Pressnitz, D. Koszelewski, K. Faber, J. Pfeffer, T. Haas and W. Kroutil, Chem. –Eur. J., 2013, 19, 4030–4035 CrossRef CAS PubMed
.
- I. Oroz-Guinea and E. García-Junceda, Curr. Opin. Chem. Biol., 2013, 17, 236–249 CrossRef CAS PubMed
.
- S. Schoffelen and J. C. M. van Hest, Soft Matter, 2012, 8, 1736–1746 RSC
.
- F. Lopez-Gallego and C. Schmidt-Dannert, Curr. Opin. Chem. Biol., 2010, 14, 174–183 CrossRef CAS PubMed
.
- J. H. Schrittwieser, J. Sattler, V. Resch, F. G. Mutti and W. Kroutil, Curr. Opin. Chem. Biol., 2011, 15, 249–256 CrossRef CAS PubMed
.
- A. C. Pierre, Biocatal. Biotransform., 2004, 22, 145–170 CrossRef CAS
.
- L. Betancor and H. R. Luckarift, Trends Biotechnol., 2008, 26, 566–572 CrossRef CAS PubMed
.
- L. Betancor, C. Berne, H. R. Luckarift and J. C. Spain, Chem. Commun., 2006, 3640–3642 RSC
.
- S. M. Kuiper, M. Nallani, D. M. Vriezema, J. L. M. Cornelissen, J. C. M. van Hest, R. J. M. Nolte and A. E. Rowan, Org. Biomol. Chem., 2008, 6, 4315–4318 CAS
.
- J. Muller and C. M. Niemeyer, Biochem. Biophys. Res. Commun., 2008, 377, 62–67 CrossRef PubMed
.
- O. I. Wilner, S. Shimron, Y. Weizmann, Z. G. Wang and I. Willner, Nano Lett., 2009, 9, 2040–2043 CrossRef CAS PubMed
.
- O. I. Wilner, Y. Weizmann, R. Gill, O. Lioubashevski, R. Freeman and I. Willner, Nat. Nanotechnol., 2009, 4, 249–254 CrossRef CAS PubMed
.
- C. J. Delebecque, A. B. Lindner, P. A. Silver and F. A. Aldaye, Science, 2011, 333, 470–474 CrossRef CAS PubMed
.
- J. E. Dueber, G. C. Wu, G. R. Malmirchegini, T. S. Moon, C. J. Petzold, A. V. Ullal, K. L. J. Prather and J. D. Keasling, Nat. Biotechnol., 2009, 27, 753–759 CrossRef CAS PubMed
.
- M. C. Good, J. G. Zalatan and W. A. Lim, Science, 2011, 332, 680–686 CrossRef CAS PubMed
.
- K. M. Manesh, P. Santhosh, S. Uthayakumar, A. I. Gopalan and K. P. Lee, Biosens. Bioelectron., 2010, 25, 1579–1586 CrossRef CAS PubMed
.
- G. Delaittre, I. C. Reynhout, J. L. M. Cornelissen and R. J. M. Nolte, Chem. –Eur. J., 2009, 15, 12600–12603 CrossRef CAS PubMed
.
- Z. M. Fan, K. Wagschal, W. Chen, M. D. Montross, C. C. Lee and L. Yuan, Appl. Environ. Microbiol., 2009, 75, 1754–1757 CrossRef CAS PubMed
.
- H. Hirakawa, N. Kamiya, T. Tanaka and T. I Nagamune, Protein Eng., Des. Sel., 2007, 20, 453–459 CrossRef CAS PubMed
.
- D. Liu, Z. Wang and X. Jiang, Nanoscale, 2011, 3, 1421–1433 RSC
.
- J. Liu and Y. Lu, Chem. Commun., 2007, 4872–4874 RSC
.
- Y. Dong, H. Zhang, Z. Rahman, L. Su, X. Chen, J. Hu and X. Chen, Nanoscale, 2012, 4, 3969–3976 RSC
.
- J. Li, L. Wu, S. Guo, H. Fu, G. Chen and H. Yang, Nanoscale, 2013, 5, 619–623 RSC
.
- M. Mancuso, L. Jiang, E. Cesarman and D. Erickson, Nanoscale, 2013, 5, 1678–1686 RSC
.
- Y. Jiang, H. Zhao, N. N. Zhu, Y. Q. Lin, P. Yu and L. Q. Mao, Angew. Chem., Int. Ed., 2008, 47, 8601–8604 CrossRef CAS PubMed
.
- J. Sun, J. Ge, W. Liu, Z. Fan, H. Zhang and P. Wang, Chem. Commun., 2011, 47, 9888–9890 RSC
.
- J. Sun, J. Ge, W. Liu, X. Wang, Z. Fan, W. Zhao, H. Zhang, P. Wang and S.-T. Lee, Nano Res., 2012, 5, 486–493 CrossRef CAS PubMed
.
- H. Wei and E. Wang, Anal. Chem., 2008, 80, 2250–2254 CrossRef CAS PubMed
.
- J. Mu, Y. Wang, M. Zhao and L. Zhang, Chem. Commun., 2012, 48, 2540–2542 RSC
.
- R. Li, M. Zhen, M. Guan, D. Chen, G. Zhang, J. Ge, P. Gong, C. Wang and C. Shu, Biosens. Bioelectron., 2013, 47, 502–507 CrossRef CAS PubMed
.
- M. Ornatska, E. Sharpe, D. Andreescu and S. Andreescu, Anal. Chem., 2011, 83, 4273–4280 CrossRef CAS PubMed
.
- L. Su, J. Feng, X. Zhou, C. Ren, H. Li and X. Chen, Anal. Chem., 2012, 84, 5753–5758 CrossRef CAS PubMed
.
- Y. Song, K. Qu, C. Zhao, J. Ren and X. Qu, Adv. Mater., 2010, 22, 2206–2210 CrossRef CAS PubMed
.
- X. Wang, K. Qu, B. Xu, J. Ren and X. Qu, Nano Res., 2011, 4, 908–920 CrossRef CAS PubMed
.
- J. Ge, D. J. Lei and R. N. Zare, Nat. Nanotechnol., 2012, 7, 428–432 CrossRef CAS PubMed
.
- L. Zhu, L. Gong, Y. Zhang, R. Wang, J. Ge, Z. Liu and R. N. Zare, Chem.–Asian J., 2013, 8, 2358–2360 CrossRef CAS PubMed
.
- C. Harford and B. Sarkar, Acc. Chem. Res., 1997, 30, 123–130 CrossRef CAS
.
Footnote |
† Electronic supplementary information (ESI) available. See DOI: 10.1039/c3nr04425d |
|
This journal is © The Royal Society of Chemistry 2014 |
Click here to see how this site uses Cookies. View our privacy policy here.