DOI:
10.1039/C4AN01443J
(Paper)
Analyst, 2015,
140, 197-203
In vivo fluorescence sensing of the salicylate-induced change of zinc ion concentration in the auditory cortex of rat brain†
Received
6th August 2014
, Accepted 12th September 2014
First published on 12th September 2014
Abstract
This study demonstrates a fluorescence method for in vivo sensing of the dynamic change of Zn2+ concentration in auditory cortex microdialysates induced by salicylate with N′-(7-nitro-2,1,3-benzoxadiazole-4-yl)-N,N,N′-tris(pyridine-2-ylmethyl) ethane-1,2-diamine (NBD-TPEA) as a probe. The excellent properties of the NBD-TPEA probe make it possible to achieve a high selectivity for Zn2+ sensing with the co-existence of amino acids and other metal ions as well as the species commonly existing in the cerebral system. To validate the method for in vivo fluorescence sensing of Zn2+ in the rat brain, we pre-mix the microdialysates in vivo sampled from the auditory cortex with the NBD-TPEA probe and then perfuse the mixtures into a fluorescent cuvette for continuous-flow fluorescence detection. The method demonstrated here shows a linear relationship between the signal output and Zn2+ concentration within the concentration range from 0.5 μM to 4 μM, with a detection limit of 156 nM (S/N = 3). The basal level of extracellular Zn2+ in auditory cortex microdialysates is determined to be 0.52 ± 0.082 μM (n = 4). This value is increased by the injection of 100 mg mL−1 of salicylate (1 μL min−1, 5 min, i.p.), reaches a peak at the time point of 90 min, and levels off with time. Such an increase is attenuated by the injection of MK-801, a potent and specific NMDA receptor antagonist, after the pre-injection of 100 mg mL−1 salicylate for 5 min. This study offers a fluorescence method for in vivo sensing of Zn2+ in the rat brain that could be useful for the investigations of chemical processes involved in brain functions.
The understanding of the chemical processes involved in the salicylate-induced physiological and pathological events is of great importance since, as one kind of anti-inflammatory component of aspirin, salicylate has been widely used to treat various ailments.1 However, excessive salicylate would induce a series of diseases, such as allergic reactions, hepatic and renal dysfunction, tinnitus and hearing loss.2 For example, previous attempts have demonstrated that a systemic injection of 250 mg kg−1 of salicylate can significantly reduce the sound evoked field output of the rat cochlea, in which salicylate competes with the contribution of cytoplasmic chloride to nonlinear capacitance of sensory outer hair cells.3 On the other hand, in the salicylate-induced physiological and pathological events, a comprehensive chemical process has been proposed to be involved.4 For instance, the injection of high doses of salicylate evokes excessive glutamate efflux from inner hair cells into the cochlea, which not only results in cochlear excitotoxicity but also leads to temporary hearing threshold shift.5 Moreover, the injection of salicylate could decrease cochlear perilymph ascorbate of guinea pigs, as demonstrated in our previous study,6 suggesting that ascorbate may be involved in the chemical processes of the salicylate-induced tinnitus. In spite of these previous studies, the chemical processes involved in the salicylate-induced physiological and pathological events remain to be further explored and understood.
As one kind of the most abundant transition metal elements that are present in all organs and bodies, Zn2+ has been demonstrated to play critical roles in many physical and pathological processes, especially in brain function activity.7 For instance, in the central nervous system, Zn2+ acts as an important endogenous neuromodulator for glutamate transmission.8 During forebrain ischemia, a considerable amount of Zn2+ was reported to release from synaptic vesicles of glutamatergic neuronal terminal to extracellular fluid, which would cause neuronal death.9 In spite of these attempts, a close survey of literature indicates that there is no report on the role of Zn2+ in the salicylate-induced physiological and pathological events. In this context, a method that is capable of in vivo sensing of the Zn2+ level remains very essential. However, the high chemical complexity inherent in the cerebral system, the requirement of spatial resolution of the investigation on chemical issues in the physiological and pathological events, as well as the less theoretical and instrumental demand of neurotechnologies unfortunately render difficulties in applying the methods reported so far for Zn2+ measurement.10
Very recently, one of our authors has demonstrated that the NBD-TPEA probe displays a high selectivity and sensitivity towards Zn2+ due to its strong affinity of all nitrogen atoms of pyridine and amine towards Zn2+.11 Such a property of the NBD-TPEA probe potentially enables its promising application for effective sensing of Zn2+ in the cerebral system. To explore such potentiality, we sample the brain dialysates with an in vivo microdialysis technique and pre-mix the microdialysates with the fluorescent probe and finally perfuse the mixtures into a cuvette for continuous-flow fluorescence detection, as shown in Scheme 1. The combination of sample pre-mixing with continuous-flow fluorescence detection facilitates signal readout recording in a continuous-flow system, eliminates background contamination, and minimizes fluorescence bleaching, while to some extent warrants the time resolution. The method demonstrated here is highly selective and sensitive and could thus be used for monitoring the dynamic change in the Zn2+ concentration involved in various brain function activities such as salicylate-induced physiological and pathological events.
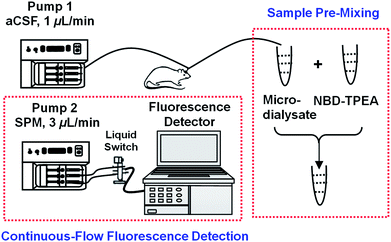 |
| Scheme 1 Schematic illustration of sample pre-mixing and continuous-flow fluorescence detection for in vivo sensing of Zn2+. | |
Experimental
Chemicals and solutions
Dopamine (DA), lactate, glucose, sodium ascorbate (AA), uric acid (UA), 3,4-dihydroxyphenylacetic acid (DOPAC), 5-hydroxytryptamine (5-HT), dizocipline [(+)-5-methyl-10,11-dihydro-5H-dibenzo [a,d] cyclohepten-5,10-imine], maleate (MK-801), and amino acids (99%) were all purchased from Sigma-Aldrich. Sodium salicylate was obtained from Sinopharm Chemical Reagent Co., Ltd. Other chemicals were of at least analytical grade and used as received. Artificial cerebrospinal fluid (aCSF) used as the perfusion solution for in vivo microdialysis and in vitro experiments was prepared by mixing NaCl (126 mM), KCl (2.4 mM), KH2PO4 (0.5 mM), MgCl2 (0.85 mM), NaHCO3 (27.5 mM), Na2SO4 (0.5 mM), and CaCl2 (1.1 mM) in Milli-Q water and the solution pH was adjusted to 7.4. All aqueous solutions were prepared with Milli-Q water (18.2 MΩ cm).
Synthesis of NBD-TPEA
Synthesis of the NBD-TPEA probe was conducted with a procedure reported previously.11 Briefly, N,N,N′-tris(pyridine-2-ylmethyl) ethane-1,2-diamine (TPEA, 2754 mg, 8.26 mmol), K2CO3 (1142 mg, 8.26 mmol), and 4-chloro-7-nitro-2,1,3-benzoxiadiazole (4-ClNBD, 1814 mg, 9.18 mmol) were mixed in 200 mL of tetrahydrofuran (THF) and the mixture was stirred overnight at room temperature. After that, the solvent was collected and evaporated under vacuum. The crude product obtained was chromatographed on silica gel by eluting with acetate/methanol (v/v, 8
:
1) to give an orange oil product with 63% yield.
In vivo microdialysis and fluorescence detection
Surgeries for in vivo microdialysis were performed as reported previously.12 Adult male Sprague-Dawley rats (350–400 g) were purchased from Center of Health Science, Peking University and housed on a 12
:
12 h light–dark scheduler with food and water ad libitum. The animals were anaesthetized with chloral hydrate (345 mg kg−1, i.p.) and positioned onto a stereotaxic frame. The microdialysis guide cannulas were carefully implanted in the auditory cortex (AP = −4.0 mm, L = 6.5 mm from bregma, V = 2.0 mm from the surface of the skull) using standard stereotaxic procedures.13 The guide cannula was in place with three skull screws and dental acrylic. Stainless steel dummy blockers were inserted into the guide cannulas and fixed until the insertion of the microdialysis probe (CMA, dialysis length, 2 mm; diameter, 0.24 mm). Throughout the surgery, the body temperature of the animals was maintained at 37 °C with a heating pad. Immediately after the surgery, the rats were placed into a warm incubator individually until they recovered from anesthesia. The rats were allowed to recover for at least 24 h before in vivo microdialysis sampling. Prior to in vivo fluorescence measurements, the microdialysis probe was implanted in the auditory cortex and was allowed to equilibrate for at least 90 min by continuously perfusing aCSF at 1 μL min−1.
The in vitro fluorescence spectrum was recorded on a Hitachi F-4600 spectrometer (Hitacho Co. Ltd. Japan) with a Xe lamp as the excitation source at room temperature. In vivo continuous-flow fluorescence detection was performed in a RF-20A xs fluorescence detector cell (Shimadzu Co. Ltd., Japan) with a Xe lamp as the excitation source at room temperature. The excitation wavelength was set as 469 nm, and the emission wavelength was set as 550 nm. To detect Zn2+ in the microdialysates in vivo sampled from the auditory cortex, we first mixed 30 μL of auditory cortex microdialysates with 30 μL of NBD-TPEA (10 μM) dissolved in DMSO/aCSF (v/v, 1
:
99, pH 7.4). After 5 min, the mixtures were perfused into a fluorescent cell with a microinjection pump (CMA 100, CMA Microdialysis AB, Stockholm, Sweden) at a perfusion rate of 3 μL min−1 for continuous-flow fluorescence detection. The basal level of Zn2+ in the microdialysates was obtained from the fluorescence response of the mixtures containing microdialysates and the NBD-TPEA probe (normal group). To study the change of the Zn2+ level in the microdialysates induced by salicylate injection, 100 mg mL−1 of sodium salicylate dissolved in phosphate buffer (0.1 M, pH 7.4) was injected into the rats (350 mg kg−1, i.p.). Such a dose was reported to produce an animal model of tinnitus.3a,b The microdialysates were collected, mixed and detected using the same procedures employed for the microdialysates from the normal group (salicylate group). To elucidate the salicylate-induced change of the Zn2+ level, in the separate experiments, we injected 1 mg mL−1 MK-801 dissolved in phosphate buffer (0.1 M, pH 7.4) into the animals (5 mg kg−1, i.p.) after the animals were injected with 100 mg mL−1 salicylate for 5 min, and the microdialysates were thus collected, mixed, and detected by the same method employed for the normal and salicylate groups (Sal/MK-801 group). During the surgery and in vivo microdialysis sampling, the body temperature of the animals was maintained at 37 °C with a heating pad and an anesthetic was supplemented if necessary.
ICP-MS analysis
Inductively coupled plasma mass spectrometry (ICP-MS) was carried out on an Agilent 7700x series ICP-MS instrument. For ICP-MS analysis, 20 μL of the microdialysates was diluted to 2 mL with Milli-Q water. Agilent ICP-MS calibration standards (multi-element) were used for preparing the calibration curves, of which the Zn2+ concentrations were 0, 0.02, 0.1, 0.5, 1, 5, 10, 50 and 100 ppb (μg mL−1), and Cd2+ concentrations were 0, 0.001, 0.01, 0.1, 1 and 10 ppb (μg mL−1). The isotopes detected were 66Zn and 111Cd, and both readings were taken in He gas mode.
Results and discussion
Fluorescent response and selectivity
In the DMSO/aCSF (v/v, 1
:
99, pH 7.4) mixture, NBD-TPEA itself shows a weak fluorescence with λex and λem at 469 nm and 550 nm, respectively, as shown in Fig. 1A. The weak emission could be attributed to the presence of two amine groups at TPEA (Fig. 1B, inset, red dashed square) as reported previously;11 TPEA connects to the 4-amino-7-nitro-2,1,3-benzoxadiazole (ANBD) fluorophore via an ethylene group and thus quenches the emission of the ANBD fluorophore via a space photoinduced electron transfer (PET) process. However, when Zn2+ was added into the mixture of DMSO/aCSF containing NBD-TPEA, the fluorescence emission intensity of NBD-TPEA was largely enhanced, as displayed in Fig. 1B (red curve). Such an enhancement was mainly caused by the PET block effect induced by the coordination of Zn2+ to all N atoms of TPEA, which leads to the recovery of the fluorescence of the ANBD fluorophore.11 In addition to its application for Zn2+ imaging in living cells with excellent biocompatibility and cell permeability,11 this mechanism is envisaged to be particularly useful for in vivo sensing of Zn2+ in the cerebral system, as described below.
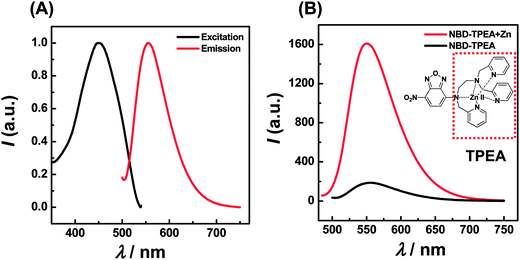 |
| Fig. 1 (A) Excitation (black curve) and emission (red curve) spectra of 10 μM of NBD-TPEA in DMSO/aCSF (v/v, 1 : 99, pH 7.4). (B) Fluorescence emission spectra of 10 μM of NBD-TPEA in the absence (black curve) and presence (red curve) of 10 μM of Zn2+ in DMSO/aCSF (v/v, 1 : 99, pH 7.4). Inset: the proposed binding mode of Zn2+ with NBD-TPEA. | |
To explore such a possibility, we systematically studied the selectivity of the method for Zn2+ sensing over other kinds of metal ions, amino acids, and physiologically important species in the cerebral system. To do this, each kind of the species was separately added into 10 μM of NBD-TPEA in the DMSO/aCSF mixture. As displayed in Fig. 2, no obvious fluorescence enhancement was observed upon the addition of each kind of metal ions (with an exception of the addition of Zn2+ and Cd2+) (A), amino acids (B), and physiologically important species (C). We also studied the effect of metal ions, amino acids and physiologically important species on the Zn2+-enhanced fluorescence intensity of NBD-TPEA and found that negligible signal changes were observed when each kind of metal ions (Fig. S1†), amino acids (Fig. S2†), and physiologically important species (Fig. S3†) was added into the mixture of 10 μM of NBD-TPEA and 10 μM of Zn2+ in DMSO/aCSF. However, the addition of Cd2+ into the same mixture clearly decreases the fluorescence intensity (data not shown), which may be caused by the competitive coordination of Cd2+ and Zn2+ with NBD-TPEA. It should be noted that the interference of Cd2+ was not considered here because of its low level in the brain microdialysates (i.e., at the nanomolar level), as determined by traditional ICP-MS in our study (data not shown). Furthermore, no significant change of the fluorescence response of 10 μM NBD-TPEA in DMSO/aCSF (v/v, 1
:
99, pH 7.4) at 550 nm was observed upon consecutive excitation with 469 nm laser light for 60 min with an interval of 1 min (data not shown), suggesting the good photostability of the NBD-TPEA probe employed in this study. The high selectivity and excellent photostability of the NBD-TPEA probe form a strong basis for in vivo sensing of Zn2+ in the cerebral system.
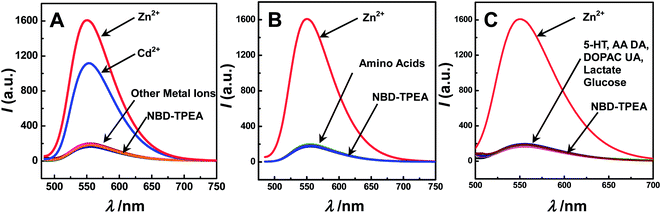 |
| Fig. 2 (A) Fluorescence emission spectra of 10 μM of NBD-TPEA in the absence (black curves) and presence of each kind of metal ions (A), amino acids (B), and physiologically important species (C) in DMSO/aCSF (v/v, 1 : 99, pH 7.4). λex = 469 nm. The concentrations of metal ions were 100 μM for K+, Na+, Ca2+, and Mg2+, and 10 μM for the other metal ions. The concentrations of each kind of amino acids were 100 μM. The concentrations of physiologically important species were 5-HT (10 μM), AA (200 μM), DA (10 μM), DOPAC (10 μM), glucose (10 mM), lactate (1 mM) or UA (80 μM). The black curve overlaps with the curves with the colors rather than blue and red. λex = 469 nm. | |
Fluorescence sensing of Zn2+
In order to validate the selective fluorescence mechanism demonstrated above for in vivo sensing of Zn2+ in rat brain, we premixed the brain microdialysates with the probe and perfused the resulting mixtures into a fluorescent cuvette with a volume as small as 30 μL for continuous-flow fluorescence sensing (Scheme 1). With this method, we obtained a time resolution of 30 min (30 μL, with 1 μL min−1 as a perfusion rate for in vivo microdialysis). Such a time resolution, although remained to be further improved in our future study, could meet the requirement for understanding the chemical process involved in the salicylate-induced physiological and pathological events such as tinnitus since previous attempts have demonstrated that the development of salicylate-induced tinnitus was a slow process.4a,b Moreover, the method demonstrated here also facilitates the fluorescence signal readout recording with less fluorescence bleaching because of the easily switchable properties and continuous-flow feature of the samples in the micro-volume fluorescent cuvette. As displayed in Fig. 3, the method shows a well-defined response toward Zn2+ and the relative fluorescence intensity ((F − F0)/F0) was linear with the concentration of Zn2+ with a dynamic linear range within the concentration range from 0.5 μM to 4 μM ((F − F0)/F0 = 0.8471 C μM−1 + 0.1397, R2 = 0.9844). Here, F0 represents the fluorescence intensity of blank NBD-TPEA solution and F represents the fluorescence intensity of the mixtures of the NBD-TPEA probe and Zn standards (or the microdialysates for in vivo analysis). The detection limit was calculated to be 156 nM (S/N = 3). Remarkably, the use of the relative fluorescence intensity (i.e., (F − F0)/F0) as the signal readout for the calibration eliminates background contamination that may be inherent in in vivo sensing of Zn2+ (possibly all kinds of trace amounts of metal ions) in the rat brain since the contamination may come from labware, reagents, and environmental contributions within the whole operation procedure.14a To achieve the (F − F0)/F0 value, in a conventional fluorescence method, both F and F0 were read out at the maximum fluorescence emission wavelength, which need to be accurately defined under a provided maximum excitation wavelength. However, in the method demonstrated here, the easily switchable and continuous-flow character of the solutions in the cuvette further facilitates the fluorescence signal readout since both the F and F0 values reach their steady-state values in the continuous-flow cell and could be easily defined and read out. Moreover, the continuous-flow character of the solutions containing the fluorescent probe in the cuvette could minimize the fluorescence bleaching as compared with that in the quiescent solution.
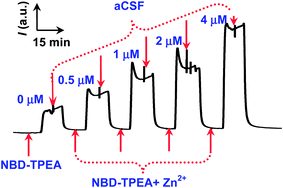 |
| Fig. 3 Typical fluorescence–time response obtained using the method for the Zn2+ standards. 30 μL of different concentrations of Zn2+ in aCSF (pH 7.4) was pre-mixed with 30 μL of NBD-TPEA (10 μM) in DMSO/aCSF (v/v, 1 : 99, pH 7.4) for 5 min, and the resulting mixtures were perfused into a fluorescent cell for continuous-flow fluorescence detection. Flow rate, 3 μL min−1. The final concentrations of Zn2+ are indicated in the figure. | |
Application for selective sensing of salicylate-induced Zn2+ in the rat brain
To demonstrate the application of our method for selectively sensing Zn2+ in the rat brain, we collected the brain microdialysates, pre-mixed the microdialysates with NBD-TPEA in DMSO/aCSF (v/v, 1
:
99, pH 7.4) and perfused the resulting mixture into a fluorescent cuvette for continuous-flow fluorescence detection (Scheme 1). As shown in Fig. S4A† and 4A (black curve), the pre-mixing of 30 μL of the microdialysates from the auditory cortex of the normal group leads to an obvious increase in the fluorescence intensity, suggesting the presence of Zn2+ in the auditory cortex microdialysates. The fluorescence intensity remains almost unchanged as a function of time from 0 min to 150 min for in vivo microdialysis in the auditory cortex, as displayed in Fig. 4A (black curve), suggesting that the basal level of auditory cortex Zn2+ does not change in the normal group in the time scale employed in this study. The basal level of Zn2+ in the auditory cortex microdialysates was determined to be 0.52 ± 0.082 μM (n = 4). To ensure the result obtained by the fluorescence method, we further determined the basal level of Zn2+ by traditional ICP-MS, in which the signal obtained for aCSF (i.e., without addition of microdialysates) was subtracted to minimize the background contamination. We found that the result obtained by our method was almost consistent with the value determined using the traditional ICP-MS method (i.e., 0.64 ± 0.10 μM, n = 4). It should be noted that the basal level of Zn2+ in the brain microdialysates reported so far remains different, ranging from 19 nM to several hundred nanomolar or even higher.14 The difference might be due to the difference in animal models, brain regions, and experimental conditions (for example, the probes and perfusion rates used for in vivo microdialysis) employed for in vivo sensing of Zn2+, as reported previously.14
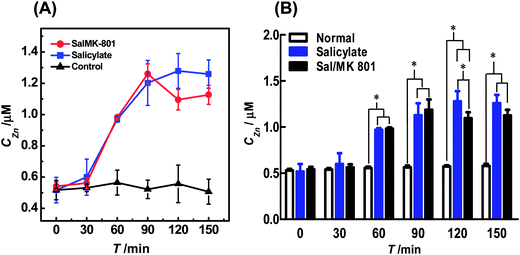 |
| Fig. 4 (A) Dynamic changes of auditory cortex Zn2+ in the normal (black curve), salicylate (blue curve) and Sal/MK-801 (red curve) groups (n = 4). All brain microdialysates (with a perfusion rate of 1 μL min−1) were pre-mixed with 30 μL of NBD-TPEA (10 μM) in DMSO/aCSF (v/v, 1 : 99, pH 7.4) for 5 min, and the resulting mixtures were perfused into a fluorescent cell for continuous-flow fluorescence detection. The perfusion rate for the mixtures was 3 μL min−1. The other conditions were the same as those in Fig. 3. (B) Comparison of the dynamic changes of auditory cortex Zn2+ levels of the normal group (white bars), salicylate group (blue bars), and Sal/MK-801 group (black bars). Data are presented as mean ± SD. The asterisks (*P < 0.01) indicate significant differences among auditory cortex Zn2+ levels of the normal group, salicylate group, and Sal/MK-801 group. | |
To further study the change of the Zn2+ level induced by salicylate, 100 mg mL−1 of sodium salicylate in phosphate buffer (0.1 M, pH 7.4) was intraperitoneally injected into the animals (350 mg kg−1, i.p.). This dose of sodium salicylate has been reported to be able to induce tinnitus.2a,3a The microdialysates were analyzed by the method using the same procedures employed for those sampled from the normal animals. As illustrated in Fig. S4B† and 4A (blue curve), the injection of salicylate into the animals leads to an increase in the level of extracellular Zn2+ in auditory cortex microdialysates from the point of 60 min. The level of Zn2+ reaches its maximum (i.e., 1.20 ± 0.24 μM, n = 4) at the time point of 90 min and then levels off with time after salicylate injection. Previous attempts have demonstrated that the development of salicylate-induced tinnitus was a slow process,4a,b and the results obtained here suggest that Zn2+ in the auditory cortex might be involved in the development of salicylate-induced tinnitus.
To further confirm the involvement of auditory cortex Zn2+ in the development of salicylate-induced tinnitus, we injected 1 mg mL−1 of MK-801, a potent and specific NMDA receptor antagonist that blocks both the excitatory and toxic actions of NMDA,15 into the animals (5 mg kg−1, i.p., in 0.1 M phosphate buffer, pH 7.4) after the injection of 100 mg mL−1 of salicylate in phosphate buffer (0.1 M pH 7.4) for 5 min (Sal/MK-801 group) and the concentration of Zn2+ in the microdialysates was detected by the method using the same procedures as those used for the salicylate group. As illustrated in Fig. S4C† and 4A (red curve), the concentration of Zn2+ in the microdialysates of the Sal/MK-801 group first increased from 0 min to 90 min, and then decreased after 90 min. This change was rather different from the results obtained for the salicylate group, suggesting that the salicylate-induced increase in the extracellular Zn2+ level was suppressed after the injection of MK-801.
For better comparison, we summarized the auditory cortex Zn2+ levels of normal, salicylate, and Sal/MK-801 groups recorded with our method. As illustrated in Fig. 4B, the auditory cortex Zn2+ level does not significantly increase both in salicylate (blue bars) and Sal/MK-801 (black bars) groups at the time point of 30 min, as compared with basal levels of the normal group (white bars). However, the auditory cortex Zn2+ levels in both salicylate and Sal/MK-801 groups increase obviously from 60 min to 150 min, as compared with basal levels of the normal group. Two-way ANOVA followed by a post hoc Tukey test indicated significant differences at each time point (from 60 min to 150 min) between the salicylate group and normal group, and between the Sal/MK-801 group and normal group (F = 30.46, P < 0.01; P = 0.01, F(5,18) = 2.77). It should be noted that, at the time point of 90 min, although the auditory cortex Zn2+ level of the Sal/MK-801 group was higher than that of the salicylate group, there is no significant difference between the salicylate group and Sal/MK-801 group. At the time point of 120 min, there were significant differences in the auditory cortex Zn2+ levels among the normal group, salicylate group and Sal/MK-801 group. These results demonstrated that the attenuation of the salicylate-induced increase of the auditory cortex Zn2+ level is mainly caused by the intraperitoneal injection of MK-801. It has been reported that MK-801, as an NMDA receptor antagonist, is competitive to combine with NMDA rather than common neurotransmitters such as glutamate.15 We speculated that the injection of MK-801 may effectively reduce the NMDA receptor excitotoxicity and eventually inhibit the oxidative damage of the auditory cortex and, as a result, attenuate the salicylate-induced increase of the extracellular Zn2+ level in the auditory cortex. These results demonstrate that the method developed here could be used for in vivo sensing of Zn2+ in the cerebral system and will find interesting applications in the investigations of brain functions.
Conclusions
By using NBD-TPEA as the selective fluorescent probe and in vivo microdialysis as the sampling technique, we have successfully developed a fluorescence method for in vivo sensing of the extracellular Zn2+ level in the auditory cortex and its change induced by salicylate. To accomplish in vivo fluorescence sensing with simple signal readout recording, minimal fluorescence bleaching, less background contamination and acceptable time resolution, the microdialysates continuously sampled from the auditory cortex were pre-mixed with the NBD-TPEA probe, and the mixtures were perfused into a fluorescent cuvette for continuous-flow fluorescence detection to form a new method. The method is highly selective and sensitive for sensing of the dynamic change in the Zn2+ level in the brain microdialysates and will find interesting applications in the investigations of brain functions.
Acknowledgements
We acknowledge the financial support from the National Natural Science Foundation of China (21321003, 21210007, 21127901, 91213305, and 91232000 for L.M., and 21175141 for L.Y.), the National Basic Research Program of China (the 973 programs 2010CB33502 and 2013CB933704), and the Chinese Academy of Sciences.
References
-
(a) W. Sun, J. Lu, D. Stolzberg, L. Gray, A. Deng, E. Lobarinas and R. Salvi, J. Neurosci., 2009, 159, 325–334 CrossRef CAS PubMed
;
(b) N. Gong, M. Zhang, X. Zhang, L. Chen, G. Sun and T. Xu, Neuropharmacology, 2008, 54, 454–463 CrossRef CAS PubMed
.
-
(a) J. Ruel, C. Chabbert, R. Nouvian, R. Bendris, M. Eybalin, C. L. Leger, J. Bourien, M. Mersel and J. L. Puel, J. Neurosci., 2008, 29, 7313–7323 CrossRef PubMed
;
(b) M. J. Guitton, J. Caston, J. Ruel, R. M. Johnson, R. Pujol and J. L. Puel, J. Neurosci., 2003, 9, 3944–3952 Search PubMed
.
-
(a) G. Yang, E. Lobarinas, L. Zhang, J. Turner, D. Stolzberg, R. Salvi and W. Sun, Hear. Res., 2007, 226, 244–253 CrossRef CAS PubMed
;
(b) M. Zhi, J. T. Ratnanather, E. Ceyhan, A. S. Popel and W. E. Brownell, Hear. Res., 2007, 228, 95–104 CrossRef CAS PubMed
.
-
(a)
M. Knipper, M. Müller and U. Zimmermann, Springer Handbook of Auditory Research, Springer, New York, 2012, ch. 3, p. 47 Search PubMed
;
(b) J. J. Eggermont and M. Kenmochi, Hear. Res., 1998, 117, 149–160 CrossRef CAS
;
(c) D. Oliver, D. Z. He, N. Klocker, J. Ludwig, U. Schulte, S. Waldegger, J. P. Ruppersberg, P. Dallos and B. Fakler, Science, 2001, 292, 2340–2343 CrossRef CAS PubMed
.
-
(a) J. Ruel, J. Wang, G. Rebillard, M. Eybalin, R. Lloyd, R. Pujol and J. L. Puel, Hear. Res., 2007, 227, 19–27 CrossRef CAS PubMed
;
(b) K. Tabuchi, B. Nishimura, S. Tanaka, K. Hayashi, Y. Hirose and A. Hara, Curr. Neuropharmacol., 2010, 8, 128–134 CrossRef CAS PubMed
.
- J. Liu, P. Yu, Y. Lin, N. Zhou, T. Li, F. Ma and L. Mao, Anal. Chem., 2012, 84, 5433–5438 CrossRef CAS PubMed
.
-
(a) R. J. Radford and S. J. Lippard, Curr. Opin. Chem. Biol., 2013, 17, 129–136 CrossRef CAS PubMed
;
(b) S. L. Sensi, P. Paoletti, A. I. Bush and I. Sekler, Nat. Rev. Neurosci., 2009, 10, 780–789 CrossRef CAS PubMed
;
(c) C. J. Frederickson, J. Y. Koh and A. I. Bush, Nat. Rev. Neurosci., 2005, 6, 449–462 CrossRef CAS PubMed
;
(d) L. C. Costello and R. B. Franklin, J. Biol. Inorg. Chem., 2011, 16, 3–8 CrossRef CAS PubMed
;
(e) S. C. Burdette and S. J. Lippard, Proc. Natl. Acad. Sci. U. S. A., 2003, 100, 3605–3610 CrossRef CAS PubMed
;
(f) C. B. Coelho, R. Tyler and M. Hansen, Prog. Brain Res., 2007, 166, 279–285 CAS
.
-
(a) A. Takeda, S. Takefuta, S. Okada and N. Oku, Brain Res., 2000, 859, 352–357 CrossRef CAS
;
(b) S. W. Suh, S. J. Won, A. M. Hamby, B. H. Yoo, Y. Fan, C. T. Sheline, H. Tamano, A. Takeda and J. Liu, J. Cereb. Blood Flow Metab., 2009, 29, 1579–1588 CrossRef CAS PubMed
;
(c) K. M. Dean, Y. Qin and A. E. Palmer, Biochim. Biophys. Acta, 2012, 1823, 1406–1415 CrossRef CAS PubMed
;
(d) P. Paoletti, A. M. Vergnano, B. Barbour and M. Casado, Neuroscience, 2009, 158, 126–136 CrossRef CAS PubMed
;
(e) J. Qian and J. L. Noebels, J. Physiol., 2005, 566, 747–758 CrossRef CAS PubMed
;
(f) J. Qian and J. L. Noebels, J. Neurosci., 2006, 26, 6089–6095 CrossRef CAS PubMed
.
-
(a) G. Wei, C. J. Hough, Y. Li and J. M. Sarvey, Neuroscience, 2004, 125, 867–877 CrossRef CAS PubMed
;
(b) R. B. Thompson, W. O. Whetsell Jr, B. P. Maliwal, C. A. Fierke and C. J. Frederickson, J. Neurosci. Methods, 2000, 96, 35–45 CrossRef CAS
;
(c) S. Ueno, M. Tsukamoto, T. Hirano, K. Kikuchi, M. K. Yamada, N. Nishiyama, T. Nagano, N. Matsuki and Y. Ikegaya, J. Cell Biol., 2002, 158, 215–220 CrossRef CAS PubMed
.
-
(a) F. J. Jimenez-Jimenez, J. A. Molina, M. V. Aguilar, I. Meseguer, C. J. Mateos-Vega, M. J. Gonzalez-Munoz, F. Bustos, A. Martinez-Salio, M. Orti-Pareja, M. Zurdo and M. C. Martinez-Para, J. Neural Transm., 1998, 105, 497–505 CrossRef CAS
;
(b) A. P. Jayasooriya, M. L. Ackland, M. L. Mathai, A. J. Sinclair, H. S. Weisinger, R. S. Weisinger, J. E. Halver, K. Kitajka and L. G. Puskas, Proc. Natl. Acad. Sci. U. S. A., 2005, 102, 7133–7138 CrossRef CAS PubMed
;
(c) T. Hirano, K. Kikuchi, Y. Urano and T. Nagano, J. Am. Chem. Soc., 2002, 124, 6555–6562 CrossRef CAS PubMed
;
(d) K. R. Gee, Z. L. Zhou, W. J. Qian and R. Kennedy, J. Am. Chem. Soc., 2002, 124, 776–778 CrossRef CAS PubMed
;
(e) P. K. Lee, W. H.-T. Law, H. W. Liu and K. K.-W. Lo, Inorg. Chem., 2011, 50, 8570–8579 CrossRef CAS PubMed
;
(f) R. H. Yang, K. A. Li, K. M. Wang, F. L. Zhao and F. Liu, Anal. Chem., 2003, 75, 612–621 CrossRef CAS
.
- F. Qian, C. Zhang, Y. Zhang, W. He, X. Gao, P. Hu and Z. Guo, J. Am. Chem. Soc., 2009, 131, 1460–1468 CrossRef CAS PubMed
.
-
(a) K. Liu, Y. Lin, L. Xiang, P. Yu, L. Su and L. Mao, Neurochem. Int., 2008, 52, 1247–1255 CrossRef CAS PubMed
;
(b) K. Liu, Y. Lin, P. Yu and L. Mao, Brain Res., 2009, 1253, 161–168 CrossRef CAS PubMed
;
(c) K. Liu, P. Yu, Y. Lin, Y. Wang, T. Ohsaka and L. Mao, Anal. Chem., 2013, 85, 9947–9954 CrossRef CAS PubMed
;
(d) M. Zhang, P. Yu and L. Mao, Acc. Chem. Res., 2012, 45, 533–543 CrossRef CAS PubMed
.
-
G. Paxinos and C. Waston, The Rat Brain in Stereotoxic Coordinates, Academic Press, New York, 3rd edn, 1997 Search PubMed
.
-
(a) C. J. Frederickson, L. J. Giblin, A. Krezel, D. J. McAdoo, R. N. Muelle, Y. Zeng, R. V. Balaji, R. Masalha, R. B. Thompson, C. A. Fierke, J. M. Sarvey, M. Valdenebro, D. S. Prough and M. H. Zornow, Exp. Neurol., 2006, 198, 285–293 CrossRef CAS PubMed
;
(b) D. Yang, J. Lee, M. Lin, Y. Huang, H. Liu, Y. Liang and F. Cheng, J. Am. Coll. Nutr., 2004, 23, 552S–555S CrossRef CAS
;
(c) Y. Kitamura, Y. Iida, J. Abe, M. Mifune, F. Kasuya, M. Ohta, K. Igarashi, Y. Saito and H. Saji, Brain Res. Bull., 2006, 69, 622–625 CrossRef CAS PubMed
;
(d) Y. Kitamura, Y. Iida, J. Abe, M. Mifune, F. Kasuya, M. Ohta, K. Igarashi, Y. Saito and H. Saji, Biol. Pharm. Bull., 2006, 29, 821–823 CrossRef CAS
;
(e) C. J. Frederickson, Int. Rev. Neurobiol., 1989, 31, 145–238 CrossRef CAS
.
- D. W. Choi and S. M. Rothman, Annu. Rev. Neurosci., 1990, 13, 171–182 CrossRef CAS PubMed
.
Footnote |
† Electronic supplementary information (ESI) available. See DOI: 10.1039/c4an01443j |
|
This journal is © The Royal Society of Chemistry 2015 |
Click here to see how this site uses Cookies. View our privacy policy here.