DOI:
10.1039/C5SC00877H
(Edge Article)
Chem. Sci., 2015,
6, 4255-4260
Consecutive C–F bond activation and C–F bond formation of heteroaromatics at rhodium: the peculiar role of FSi(OEt)3†
Received
10th March 2015
, Accepted 9th May 2015
First published on 26th May 2015
Abstract
C–F activation of 2,3,5,6-tetrafluoropyridine at [Rh{Si(OEt)3}(PEt3)3] (1) yields [Rh{2-(3,5,6-C5F3HN)}(PEt3)3] (2) and FSi(OEt)3, but in an unprecedented consecutive reaction FSi(OEt)3 acts as a fluoride source to give [Rh(4-C5F4N)(PEt3)3] (4) by regeneration of the C–F bond and C–H activation. Analogous refluorination steps were observed for other 2-pyridyl rhodium complexes. NMR spectroscopic studies revealed a delicate balance between the feasibility for C–F bond formation accompanied by a C–H activation and the occurrence of competing reactions such as hydrodefluorinations induced by the intermediary presence of H2.
Introduction
Fluorinated organic compounds are of considerable interest because of their wide range of applications in materials science and medicine.1 Therefore, there is a strong demand to access new fluorinated building blocks, but there is also enormous interest regarding their reactivity. Transition-metal-mediated routes to fluoroorganics often involve fluorination of non-fluorinated precursors2 or selective C–F bond cleavage reactions at highly fluorinated molecules.3 The latter approach frequently comprises the formation of metalated fluorinated entities, which can then be functionalized in the coordination sphere of the transition metal via very sophisticated reaction pathways.3 C–F bond activation steps are often challenged by the particularly high strength of the C–F bond.4 One requirement to achieve the C–F bond cleavage is, therefore, the formation of very stable element–fluorine bonds, such as H–F, B–F, Al–F or Si–F bonds.3m–r Thus, suitable metal complexes for an initial activation step might possess hydrido, boryl or silyl ligands which react with highly fluorinated organics to yield HF, fluoroboranes or fluorosilanes and the metal complex bearing the fluorinated organyl ligand.3l
Another important issue in C–F bond activations can be their regio- and chemoselectivity. Often there is a preference of C–H activation over C–F activation, but there are also a considerable number of examples in which a C–F bond is cleaved in the presence of a C–H bond.3,5 Previously, we reported that the rhodium(I) complexes [RhH(PEt3)3] (6), [Rh(Bpin)(PEt3)3] (HBpin = 4,4,5,5-tetramethyl-1,3,2-dioxaborolane, pinacolborane) and [Rh{Si(OR)3}(PEt3)3] (R = Me, Et) are useful starting compounds for various C–F bond activation reactions.6 For instance, [RhH(PEt3)3] (6) reacted with pentafluoropyridine to give [Rh(4-C5NF4)(PEt3)3] (4) by C–F activation at the 4-position,6e whereas conversions with [Rh(Bpin)(PEt3)3] and [Rh{Si(OR)3}(PEt3)3] (R = Me, 1: R = Et) resulted in an activation at the 2-position to yield [Rh(2-C5NF4)(PEt3)3].6f,g DFT calculations on the activation in the model compounds [Rh(Bpin)(PMe3)3] and [Rh{Si(OMe)3}(PMe3)3] suggested a ligand-assisted reaction pathway, which involves a direct transfer of fluorine to the boron or silicon centers via four-membered transition states. For [Rh{Si(OEt)3}(PEt3)3] (1) a phosphine dissociation seemed to occur prior to the C–F activation step. However, treatment of [RhH(PEt3)3] (6) or [Rh(Bpin)(PEt3)3] with 2,3,5,6-tetrafluoropyridine selectively led to [Rh(4-C5NF4)(PEt3)3] (4) by C–H activation. In contrast, [Rh{Si(OR)3}(PEt3)3] (R = Me, 1: R = Et) reacted with 2,3,5,6-tetrafluoropyridine exclusively by a C–F activation at the 2-position to afford [Rh{2-(3,5,6-C5F3HN)}(PEt3)3] (2) and fluorosilane.6e–g Earlier, Marder and Perutz et al. observed, in a reaction of 2,3,5,6-tetrafluoropyridine with [Rh(SiPh3)(PMe3)3], C–F activation at the ortho-position as well as C–H activation accompanied with the formation of FSiPh3 and HSiPh3 in a nearly 1
:
1 ratio. The resulting products were stable towards FSiPh3.5f
Herein we report on the very peculiar role of FSi(OEt)3 in C–F bond activation and C–F bond formation reactions of fluorinated pyridines at Rh. Although the promotion of C–F activation steps by the generation of fluorosilanes is well known, a consecutive refluorination with the fluorosilane as a fluoride source is unprecedented. Formally, this Si–F activation sets back the C–F activation process with consequences for the overall chemoselectivity of bond activation reactions at fluorinated heteroaromatics. Such a reversion of a C–F activation step at a highly fluorinated aromatic ligand is unique and has never been considered before.3,7
Results and discussion
Treatment of [Rh{Si(OEt)3}(PEt3)3] (1) with stoichiometric amounts of 2,3,5,6-tetrafluoropyridine at room temperature yielded the C–F activation product [Rh{2-(3,5,6-C5F3HN)}(PEt3)3] (2) as well as approximately 10% of the non-classical silane complex [Rh(4-C5F4N){η2-HSi(OEt)3}(PEt3)2] (3) by C–H activation (Scheme 1). Traces of [Rh(4-C5F4N)(PEt3)3] (4) were also present; this compound was previously synthesized and also characterized by X-ray crystallography.6e The activation of 2,3,5,6-tetrafluoropyridine at the ortho position might occur via a similar silyl ligand-assisted pathway as stated above. However, a slight excess of 2,3,5,6-tetrafluoropyridine led to the generation of 2 only.6g An independent reaction revealed that complex 4 reacts with HSi(OEt3)3 to give [Rh(4-C5F4N){η2-HSi(OEt)3}(PEt3)2] (3) and free phosphine. Addition of an excess of PEt3 to the reaction solution of 3 led to the regeneration of 4 (Scheme 1).
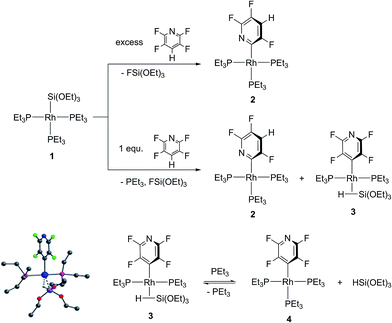 |
| Scheme 1 Reactivity of 1 towards 2,3,5,6-tetrafluoropyridine to yield 2 and 3. Computed structure of 3; hydrogen atoms of the Et groups are omitted for clarity. | |
Complex 3 could not be isolated in a pure form, because of the presence of 4, and was characterized via NMR spectroscopy only. For the hydrogen atom of the metal-bound silane HSi(OEt)3 a multiplet at δ = −12.9 ppm was observed in the 1H NMR spectrum. 19F decoupling experiments revealed a doublet of triplets with a coupling constant of 20 Hz to the rhodium atom and a coupling constant of 14 Hz to the phosphorus atoms. The 29Si,1H HMBC NMR spectrum exhibited a resonance at δ = −39 ppm in the 29Si domain, which correlates to the signals of the Si–H hydrogen and the CH2 groups of the ethoxy moieties. The spectrum revealed a hydrogen–silicon coupling constant of 65 Hz which is typical for non-classical silane complexes.8 DFT calculations were performed to model the structure of 3 which is depicted in Scheme 1. The calculations confirmed the assignment as a η2-silane complex and revealed a Si–H distance of 1.824 Å.
Remarkably, the NMR spectra of the mixture of the complexes [Rh{2-(3,5,6-C5F3HN)}(PEt3)3] (2), phosphine, triethoxyfluorosilane and [Rh(4-C5F4N){η2-HSi(OEt)3(PEt3)2] (3), which is in equilibrium with complex [Rh(4-C5F4N)(PEt3)3] (4), after 18 h showed only distinct signals for the complex 4. No resonances for the fluorosilane were detected. Monitoring this conversion by 31P and 19F NMR spectroscopy confirmed the assumption that 4 can be generated from 2 and fluorosilane. Consequently, [Rh{Si(OEt)3}(PEt3)3] (1) was treated with 2,3,5,6,-tetrafluoropyridine and the volatiles (solvent and fluorosilane) were subsequently removed from the reaction mixture and a solution of phosphine in [D8]-toluene was added. This led to a mixture of [Rh{2-(3,5,6-C5F3HN)}(PEt3)3] (2), [Rh(4-C5F4N){η2-HSi(OEt)3(PEt3)2] (3) and [Rh(4-C5F4N)(PEt3)3] (4) (80
:
8
:
12). Without the presence of fluorosilane, no conversion of 2 into 4 was observed within 3 h. However, addition of FSi(OEt)3 triggered the generation of 4 from 2, which again confirmed the involvement of fluorosilane in the fluorination reaction. Using FSi(OMe)3 for the fluorination reaction also led to a conversion of 2 into 4, whereas using FSiEt3 did not. Furthermore, small amounts of hydrogen were detected by 1H NMR spectroscopy. However, the fate of the silicon containing units was ambiguous. 29Si,1H HMBC NMR spectra after a reaction of 2 with FSi(OEt)3 confirmed the formation of Si(OEt)4, (EtO)3SiOSi(OEt)3 and other siloxanes, which could not be further characterized. We ascribe this to a fluorination pathway proceeding via intermediate fluorosilicate anions, which might be formed in the presence of the glass surface of the reaction vessel. Silicate anions are known to undergo spontaneous and manifold condensation reactions.9 Note that a conversion of 2 into 4 was considerably slower and competing reaction pathways became dominant (see below) when the reaction was performed in a PFA tube instead of a glass NMR tube.
In order to test this hypothesis and the involvement of fluorosilicate anions in the conversion of 2 into 4, the reaction was performed in the presence of NaOEt to induce the formation of silicate anions from fluorosilane. This led to a faster conversion of 2 into 4. After 9 h, 2 was fully consumed (Fig. 1). Additionally, the generation of Si(OEt)4 was observed by NMR spectroscopy. A reaction in [D8]-THF instead of [D8]-toluene led to a slight increase of the reaction rate. Addition of EtOH instead of ethanolate also led to an increase of the reaction rate, but the concomitant evolution of H2 resulted in the generation of additional hydrodefluorination products (29%, see also below).10 The use of ethanol-D6 led to the formation of Si(OEt)3(OCD2CD3), as was evident from a 2H NMR spectrum.
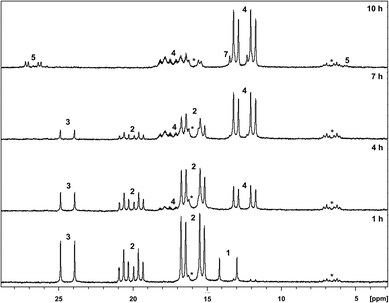 |
| Fig. 1 Parts of the 31P{1H} NMR spectra (121.5 MHz in [D8]toluene) which reveal the fluorination of 2 in the presence of FSi(OEt)3 and NaOEt to yield 4. In side reactions 5, 7 and cis,fac-[Rh(H)2{Si(OEt)3}(PEt3)3] (*) were formed. | |
The formation of hydrogen was only observed at the beginning of the fluorination reaction, because it undergoes a number of subsequent reactions (Scheme 2). (i) The complex [Rh(4-C5F4N)(PEt3)3] (4) and H2 are in equilibrium with the oxidative addition product [Rh(H)2(4-C5F4N)(PEt3)3] (5), which was identified in the reaction solution.6d (ii) Complex 5 can release 2,3,5,6-tetrafluoropyridine and form [RhH(PEt3)3] (6), which explains the detection of small amounts of 2,3,5,6-tetrafluoropyridine. (iii) In a reaction competing with the generation of 4 from [Rh{2-(3,5,6-C5F3HN)}(PEt3)3] (2), the formation of [Rh{4-(2,3,5-C5F3HN)}(PEt3)3] (7) was observed, which can be explained by a reaction 2 with H2 to give 2,3,5-trifluoropyridine and [RhH(PEt3)3] (6), and a subsequent C–H activation at the 4-position of the 2,3,5-trifluoropyridine at 6 (Scheme 2). The reaction steps that led to the generation of 7 or 2,3,5-trifluoropyridine were confirmed by independent experiments. Note that hydrogenolysis was reported before for pentafluoropyridine to yield 2,3,5,6-tetrafluoropyridine in the presence of H2.6d (iv) Finally, complex 6 might react further with HSi(OEt)3, which stems from [Rh(4-C5F4N){η2-HSi(OEt)3(PEt3)2] (3) to give cis,fac-[Rh(H)2{Si(OEt)3}(PEt3)3].6g Note that the ratio of products formed in the competing reactions strongly depended on the reaction conditions. In the presence of ethanol more hydrodefluorination products were detected, whereas ethanolate favored the fluorination reaction (for product ratios and reaction conditions see ESI†).
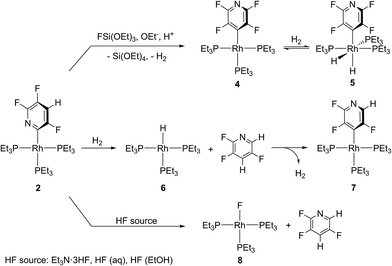 |
| Scheme 2 Reactivity of 2 towards FSi(OEt)3, H2 and HF. | |
A proposed mechanism for the fluorination of 2 is shown in Scheme 3. Although we assume a rather complicated reaction pattern, we exemplify the fluorination process by the intermediary formation of pentacoordinated fluorosilicate anions such as [FSi(OEt)4]−. The latter might be formed by the reaction of FSi(OEt)3 and OEt− or ethanol.11 The use of ethanol requires an additional base like PEt3.9c,12 For such a model a transfer of F− to the ortho-carbon atom of the pyridyl ligand of [Rh{2-(3,5,6-C5F3HN)}(PEt3)3] (2) would yield a Meisenheimer-type intermediate A (Scheme 3).13 Alternatively, the fluoride anion can be bound to the rhodium atom and then migrate to the carbon atom to give the same intermediate species A. In A the rhodium atom can migrate from the 2-position of the anionic tetrafluoropyridyl ligand to the 4-position to give B. Similar rearrangements, though proceeding via an intermediary η2-coordination of the aromatic compounds, were observed for reversible C–H activation reactions at the nickel fluorophenyl complex [NiH(2,3,5,6-C6F4H)(PEt3)2].14 During the formation of 4 from 2 no such intermediates were observed and we suggest a sigmatropic 1,3-rearrangement as is known for allyl complexes.15 Dihydrogen elimination by protonation with adventitious water, which might for instance be generated in condensation reactions of silicate anions,9 gives [Rh(4-C5F4N)(PEt3)3] (4). Alternatively the hydride can migrate to the metal center and protonation yields [Rh(H)2(4-C5F4N)(PEt3)3] (5).
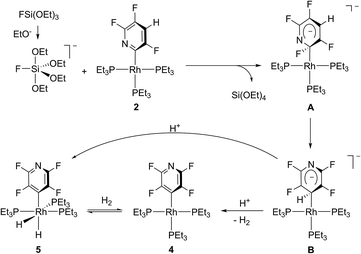 |
| Scheme 3 Simplified mechanistic proposal for the fluorination of 2 to yield 4. | |
To support a possible involvement of fluorosilicate anions in the conversion of [Rh{2-(3,5,6-C5F3HN)}(PEt3)3] (2) into [Rh(4-C5F4N)(PEt3)3] (4), complex 2 was treated with tris(dimethylamino)sulfonium difluorotrimethylsilicate (TASF), which is a source of F−.16 However, the formation of compound 4 was observed only in very small amounts. In addition, 10% of [RhF(PEt3)3] (8) and considerable amounts (42%) of rhodium complexes were generated, which could not be identified further.6b Note also, that the addition of CsF and LiF suppressed the conversion of [Rh{2-(3,5,6-C5F3HN)}(PEt3)3] (2) and FSi(OEt)3 into [Rh(4-C5F4N)(PEt3)3] (4).
Furthermore, the possible involvement of HF in the fluorination reaction of 2 to yield 4 was elucidated. Notably, 2 did not convert to 4, either with NEt3·3HF or with HF in aqueous or ethanolic solution as fluorinating agents. Instead, the generation of [RhF(PEt3)3] (8) and 2,3,5-trifluoropyridine was observed (Scheme 2). In contrast, [Rh(4-C5F4N)(PEt3)3] (4) or [Rh{4-(2,3,5-C5F3HN)}(PEt3)3] (7) showed no reactivity towards HF sources at room temperature.17
In order to investigate the scope of this unique fluorination reaction at [Rh{2-(3,5,6-C5F3HN)}(PEt3)3] (2) further, we synthesized several fluoroaryl complexes by C–H activation at [Rh(CH3)(PEt3)3] (9).18 On treatment with 1,2,3,5-tetrafluorobenzene, 1,2,4,5-tetrafluorobenzene, 2,3,5-trifluoropyridine or 2,3,6-trifluoropyridine the complexes [Rh(2,3,4,6-C6F4H)(PEt3)3] (10), [Rh(2,3,5,6-C6F4H)(PEt3)3] (11), [Rh{4-(2,3,5-C5F3HN)}(PEt3)3] (7) and [Rh{4-(2,3,6-C5F3HN)}(PEt3)3] (12) were accessible. These were then treated with FSi(OEt)3 or FSiEt3 in the presence of OEt−, but in neither case was a fluorination of the aromatic ligand observed. Apparently, a pyridyl ligand is more susceptible to fluorination than a phenyl ligand. In addition, complexes with the rhodium atom at the 2-position of the pyridyl ligand were less stable than the isomer with the aromatic ligand bound at the 4-position. Note that previous DFT calculations indicated that [Rh(4-C5F4N)(PMe3)3] was 13 kcal mol−1 more stable than [Rh(2-C5F4N)(PMe3)3].6g
Consequently, we synthesized pyridyl complexes that bear the metal at the 2-position of the heterocycle and are less fluorinated than [Rh{2-(3,5,6-C5F3HN)}(PEt3)3] (2). This can be achieved by C–F bond activation of 2,3,6- or 2,3,5-trifluoropyridine at [Rh{Si(OEt)3}(PEt3)3] (1) (Scheme 4). Remarkably, the reactions led solely to the cleavage of the C–F bond at the ortho position to the nitrogen atom in both cases, even when C–F activation was competing with C–H activation at the 2-position.3,5,6h,19
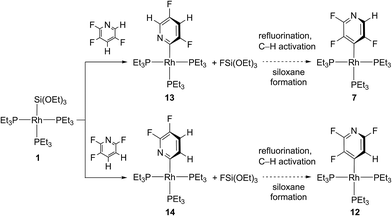 |
| Scheme 4 C–F activation of trifluoropyridines at 1 and consecutive fluorination reactions. | |
[Rh{2-(3,5-C5F2H2N)}(PEt3)3] (13) and [Rh{2-(5,6-C5F2H2N)}-(PEt3)3] (14) were only characterized by NMR spectroscopy, because small amounts of 7 or 12 were always present. Note that the signal for the fluorine atom at the ortho position to the nitrogen atom in 13 characteristically appears at low field in the 19F NMR spectrum.5a,h,6,20 However, conversions of 13 and 14 in the presence of FSi(OEt)3 led to the formation of the products of fluorination [Rh{4-(2,3,5-C5F3HN)}(PEt3)3] (7) and [Rh{4-(2,3,6-C5F3HN)}(PEt3)3] (12) (Scheme 4). These conversions took place at a slower rate when compared to the reaction of 2 with FSi(OEt)3. This is consistent with the observation that competing hydrogenolysis reactions yielded the hydrodefluorination products [RhH(PEt3)3] (6) and 3,5-difluoropyridine or 2,3-difluoropyridine before 13 and 14 were fully consumed to give 7 and 12. However, after 21 h the generation proceeded, with up to 58% for 7 and after 15 h 68% for 12 at room temperature. Conversions of 13 or 14 in a PFA tube, or in the presence of EtOH or OEt− proceeded with very low selectivity to give a range of products (in part unidentified), but very small amounts of 7 or 12.
Conclusion
In conclusion, we have reported the unique regeneration of C–F bonds at rhodium bound pyridyl ligands as a subsequent step after a C–F bond activation reaction. The crucial role of the fluorosilane FSi(OEt)3 is peculiar and it can stimulate both C–F bond cleavage and C–F bond generation. Such involvement of fluorosilanes in consecutive C–F bond activation and C–F bond formation reactions to regenerate the same C–F bond is unprecedented and has never been considered before. The observations are of fundamental importance in understanding the selectivity of C–F bond cleavage steps at transition metal centers. Selective C–H activation preceded by reversible C–F activation might also have consequences for the overall C–F versus C–H activation selectivity. For comparison, however, [RhH(PEt3)3] (6) or [Rh(Bpin)(PEt3)3] react selectively with 2,3,5,6-tetrafluoropyridine to yield [Rh(4-C5NF4)(PEt3)3] (4) and H2 or HBpin by C–H activation and there are no indications for an intermediate C–F activation step.6d–f
Acknowledgements
Support from the research training group “Fluorine as a Key Element” funded by the Deutsche Forschungsgemeinschaft is gratefully acknowledged. We would also like to thank Dr Mike Ahrens for the DFT calculations and Dr Sabrina Kalläne for an additional NMR experiment.
Notes and references
-
(a) K. P. Shine and W. T. Sturges, Science, 2007, 315, 1804 CrossRef CAS PubMed;
(b) K. Müller, C. Faeh and F. Diederich, Science, 2007, 317, 1881 CrossRef PubMed;
(c)
P. Kirsch, Modern Fluoroorganic Chemistry. Synthesis, Reactivity, Applications, Wiley-VCH, Weinheim, 2004 Search PubMed;
(d)
T. Hiyama, Organofluorine Compounds. Chemistry and Applications, 2000 Search PubMed;
(e)
J. T. Welch and S. Eswarakrishnan, Fluorine in Bioorganic Chemistry, John Wiley & Sons, 1991 Search PubMed;
(f)
A. Tressaud and G. Haufe, Fluorine and Health: Molecular Imaging, Biomedical Materials and Pharmaceuticals, Elsevier Science, 2008 Search PubMed.
- See for example:
(a) T. W. Lyons and M. S. Sanford, Chem. Rev., 2010, 110, 1147 CrossRef CAS PubMed;
(b) V. V. Grushin, Acc. Chem. Res., 2009, 43, 160 CrossRef PubMed;
(c) V. Gouverneur, Science, 2009, 325, 1630 CrossRef CAS PubMed;
(d) T. Liang, C. N. Neumann and T. Ritter, Angew. Chem., 2013, 125, 8372 (
Angew. Chem., Int. Ed.
, 2013
, 52
, 8214
) CrossRef PubMed;
(e) T. Furuya, A. S. Kamlet and T. Ritter, Nature, 2011, 473, 470 CrossRef CAS PubMed;
(f) V. Rauniyar, A. D. Lackner, G. L. Hamilton and F. D. Toste, Science, 2011, 334, 1681 CrossRef CAS PubMed;
(g) D. A. Watson, M. J. Su, G. Teverovskiy, Y. Zhang, J. García-Fortanet, T. Kinzel and S. L. Buchwald, Science, 2009, 325, 1661 CrossRef CAS PubMed;
(h) C. N. Neumann and T. Ritter, Angew. Chem., 2015, 127, 3261 (
Angew. Chem., Int. Ed.
, 2013
, 54
, 3216
) CrossRef PubMed.
-
(a) H. Amii and K. Uneyama, Chem. Rev., 2009, 109, 2119 CrossRef CAS PubMed;
(b) R. P. Hughes, Eur. J. Inorg. Chem., 2009, 31, 4591 CrossRef PubMed;
(c) J. L. Kiplinger, T. G. Richmond and C. E. Osterberg, Chem. Rev., 1994, 94, 373 CrossRef CAS;
(d) H. Torrens, Coord. Chem. Rev., 2005, 249, 1957 CrossRef CAS PubMed;
(e) J. Burdeniuc, B. Jedlicka and R. H. Crabtree, Chem. Ber., 1997, 130, 145 CrossRef CAS PubMed;
(f) W. D. Jones, Dalton Trans., 2003, 3991 RSC;
(g) M. Klahn and M. Rosenthal, Organometallics, 2012, 31, 1235 CrossRef CAS;
(h) A. Nova, R. Mas-Ballesté and A. Lledós, Organometallics, 2012, 31, 1245 CrossRef CAS;
(i) M. Crespo, Organometallics, 2012, 31, 1216 CrossRef CAS;
(j) S. A. Macgregor, Chem. Soc. Rev., 2007, 36, 67 RSC;
(k) S. A. Begum, J. Terao and N. Kambe, Chem. Lett., 2007, 36, 196 CrossRef CAS;
(l) T. Braun and F. Wehmeier, Eur. J. Inorg. Chem., 2011, 613 CrossRef CAS PubMed;
(m) D. Lentz, T. Braun and M. Kuehnel, Angew. Chem., 2013, 125, 3412 (
Angew. Chem., Int. Ed.
, 2013
, 52
, 3328
) CrossRef PubMed;
(n) E. Clot, O. Eisenstein, N. Jasim, S. A. Macgregor, J. E. McGrady and R. N. Perutz, Acc. Chem. Res., 2011, 44, 333 CrossRef CAS PubMed;
(o) G. Meier and T. Braun, Angew. Chem., 2009, 121, 1575 (
Angew. Chem., Int. Ed.
, 2009
, 48
, 1546
) CrossRef PubMed;
(p) M. Okada, Y. Nakamura, A. Saito, A. Sato, H. Horikawa and T. Taguchi, Tetrahedron Lett., 2002, 43, 5845 CrossRef CAS;
(q) J. Terao, S. A. Begum, Y. Shinohara, M. Tomita, Y. Naitoh and N. Kambe, Chem. Commun., 2007, 855 RSC;
(r) H. F. T. Klare and M. Oestreich, Dalton Trans., 2010, 9176 RSC;
(s) J. Weaver and S. Senaweera, Tetrahedron, 2014, 70, 7413 CrossRef CAS PubMed;
(t) A. D. Sun and J. A. Love, Dalton Trans., 2010, 10362 RSC;
(u)
L. Keyes and J. A. Love, C–H and C–X Bond Functionalization: Transition Metal Mediation, ed. X. Ribas, The Royal Society of Chemistry, 2013 Search PubMed;
(v) T. Ahrens, J. Kohlmann, M. Ahrens and T. Braun, Chem. Rev., 2015, 115, 931 CrossRef CAS PubMed.
-
Y. R. Luo, Comprehensive Handbook of Chemical Bond Energies, 2007 Search PubMed.
-
(a) S. J. Archibald, T. Braun, J. A. Gaunt, J. E. Hobson and R. N. Perutz, Dalton Trans., 2000, 2013 RSC;
(b) J. A. Hatnean and S. A. Johnson, Organometallics, 2012, 31, 1361 CrossRef CAS;
(c) J. A. Panetier, S. A. Macgregor and M. K. Whittlesey, Angew. Chem., 2011, 123, 2835 (
Angew. Chem., Int. Ed.
, 2011
, 50
, 2783
) CrossRef PubMed;
(d) M. Aizenberg and D. Milstein, Science, 1994, 265, 359 CAS;
(e) M. Aizenberg and D. Milstein, J. Am. Chem. Soc., 1995, 117, 8674 CrossRef CAS;
(f) R. J. Lindup, T. B. Marder, R. N. Perutz and A. C. Whitwood, Chem. Commun., 2007, 3664 RSC;
(g) M. K. Whittlesey, R. N. Perutz and M. H. Moore, Chem. Commun., 1996, 787 RSC;
(h) T. Braun, S. P. Foxon, R. N. Perutz and P. H. Walton, Angew. Chem., 1999, 111, 3543 (
Angew. Chem., Int. Ed.
, 1999
, 38
, 3326
) CrossRef;
(i) P. Fischer, K. Goetz, A. Eichhorn and U. Radius, Organometallics, 2012, 31, 1374 CrossRef CAS;
(j) B. L. Edelbach and W. D. Jones, J. Am. Chem. Soc., 1997, 119, 7734 CrossRef CAS;
(k) B. Procacci, Y. Jiao, M. E. Evans, W. D. Jones, R. N. Perutz and A. C. Whitwood, J. Am. Chem. Soc., 2015, 137, 1258 CrossRef CAS PubMed.
-
(a) D. Noveski, T. Braun, M. Schulte, B. Neumann and H.-G. Stammler, Dalton Trans., 2003, 4075 RSC;
(b) T. Braun, D. Noveski, B. Neumann and H. G. Stammler, Angew. Chem., 2002, 114, 2870 (
Angew. Chem., Int. Ed
, 2002
, 41
, 2745
) CrossRef;
(c) T. Braun, F. Wehmeier and K. Altenhöner, Angew. Chem., 2007, 119, 5415 (
Angew. Chem., Int. Ed.
, 2007
, 46
, 5321
) CrossRef PubMed;
(d) T. Braun, D. Noveski, M. Ahijado and F. Wehmeier, Dalton Trans., 2007, 3820 RSC;
(e) D. Noveski, T. Braun, B. Neumann, A. Stammler and H. G. Stammler, Dalton Trans., 2004, 4106 RSC;
(f) M. Teltewskoi, J. A. Panetier, S. A. Macgregor and T. Braun, Angew. Chem., 2010, 122, 4039 (
Angew. Chem., Int. Ed.
, 2010
, 49
, 3947
) CrossRef PubMed;
(g) A. L. Raza, J. A. Panetier, M. Teltewskoi, S. A. Macgregor and T. Braun, Organometallics, 2013, 32, 3795 CrossRef CAS;
(h) S. I. Kalläne, M. Teltewskoi, T. Braun and B. Braun, Organometallics, 2015, 34, 1156 CrossRef.
- For examples of reversible fluorinations at transition metal centers see:
(a) D. Huang, P. R. Koren, K. Folting, E. R. Davidson and K. G. Caulton, J. Am. Chem. Soc., 2000, 122, 8916 CrossRef CAS;
(b) J. A. Akana, K. X. Bhattacharyya, P. Müller and J. P. Sadighi, J. Am. Chem. Soc., 2007, 129, 7736 CrossRef CAS PubMed;
(c) C. Hollingworth, A. Hazari, M. N. Hopkinson, M. Tredwell, E. Benedetto, M. Huiban, A. D. Gee, J. M. Brown and V. Gouverneur, Angew. Chem., 2011, 123, 2661 (
Angew. Chem., Int. Ed.
, 2011
, 50
, 2613
) CrossRef PubMed;
(d) P. Kläring and T. Braun, Angew. Chem., 2013, 125, 11302 (
Angew. Chem., Int. Ed.
, 2013
, 52
, 11069
) CrossRef PubMed;
(e) J. Goodman, V. V. Grushin, R. B. Larichev, S. A. Macgregor, W. J. Marshall and D. C. Roe, J. Am. Chem. Soc., 2009, 131, 4236 CrossRef CAS PubMed.
-
(a) G. Alcaraz and S. Sabo-Etienne, Coord. Chem. Rev., 2008, 252, 2395 CrossRef CAS PubMed;
(b) L. Zámostná, M. Ahrens and T. Braun, J. Fluorine Chem., 2013, 155, 132 CrossRef PubMed.
- For silicate anions in condensation reactions see:
(a) C. G. Swain, R. M. Esteve and R. H. Jones, J. Am. Chem. Soc., 1949, 71, 965 CrossRef CAS;
(b) R. R. Holmes, Chem. Rev., 1990, 90, 17 CrossRef CAS , for other examples of silicate anions see;
(c) C. Chuit, R. J. P. Corriu, C. Reye and J. C. Young, Chem. Rev., 1993, 93, 1371 CrossRef CAS;
(d) S. Steinhauer, H.-G. Stammler, B. Neumann, N. Ignat'ev and B. Hoge, Angew. Chem., 2014, 126, 573 (
Angew. Chem., Int. Ed.
, 2014
, 53
, 562
) CrossRef PubMed;
(e) F. D. Osterholtz and E. R. Pohl, J. Adhes. Sci. Technol., 1992, 6, 127 CrossRef CAS PubMed;
(f) B. Arkles, J. R. Steinmetz, J. Zazyczny and P. Mehta, J. Adhes. Sci. Technol., 1992, 6, 193 CrossRef CAS PubMed.
- Note that silanes can be converted into Si(OEt)4 and H2 in the presence of EtOH:
(a) T. Mitsudome, Y. Yamamoto, A. Noujima, T. Mizugaki, K. Jitsukawa and K. Kaneda, Chem.–Eur. J., 2013, 19, 14398 CrossRef CAS PubMed;
(b) Z. Huang, Y. Li, Q. Tian, Z. Zhang, X. Li, X. Liang and L. Du, J. Fluorine Chem., 2014, 158, 6 CrossRef CAS PubMed.
- Many examples of pentacoordinated silicate anions are described in the literature, but no fluorosilicate anions with four ethoxy or methoxy substituents are known. Treatment of Si(OEt)4 with KF/18-crown-6 or treatment of FSi(OEt)3 with NaOEt did not yield [FSi(OEt)4]−.
-
(a) R. R. Holmes, R. O. Day and J. S. Payne, Phosphorus, Sulfur Silicon Relat. Elem., 1989, 42, 1 CrossRef CAS PubMed;
(b) C. L. Frye, J. Am. Chem. Soc., 1970, 92, 1205 CrossRef CAS.
-
(a) I. P. Beletskaya, G. A. Artamkina, A. Y. Mil'chenko, P. K. Sazonov and M. M. Shtern, J. Phys. Org. Chem., 1996, 9, 319 CrossRef CAS;
(b) A. Steffen, M. I. Sladek, T. Braun, B. Neumann and H. G. Stammler, Organometallics, 2005, 24, 4057 CrossRef CAS.
-
(a) S. A. Johnson, C. W. Huff, F. Mustafa and M. Saliba, J. Am. Chem. Soc., 2008, 130, 17278 CrossRef CAS PubMed;
(b) A competition experiment was done, in which pentafluoropyridine was added. If a reversible C–F activation mechanism is operative, the silyl complex 1 would be generated as an intermediate which would then react with pentafluoropyridine to yield [Rh(2-C5F4N)(PEt3)3]. However, no formation of any product arising from the intermediate formation of 1 was observed.
- J. García-Álvarez, S. E. García-Garrido, P. Crochet and V. Cadierno, Curr. Top. Catal., 2012, 10, 35 Search PubMed.
-
(a) J. E. Veltheer, P. Burger and R. G. Bergman, J. Am. Chem. Soc., 1995, 117, 12478 CrossRef CAS;
(b) R. R. Burch, R. L. Harlow and S. D. Ittel, Organometallics, 1987, 6, 982 CrossRef CAS.
- Note that [Rh{2-(3,5,6-C5F3HN)}(PEt3)3] (2) gives, in the presence of stoichiometric amounts of water and FSi(OEt)3, the fluoro complex [RhF(PEt3)3] (8) and 2,3,5-trifluoropyridine, which could result from a metal-mediated hydrolysis of the fluorosilane to yield HF.
- P. Zhao and J. F. Hartwig, Organometallics, 2008, 27, 4749 CrossRef CAS.
- NMR experiments showed that for 6 a C–H activation of 2,3,5-trifluoropyridin occurred at the 4-position (see Scheme 2) whereas [Rh(Bpin)(PEt3)3] reacted by C-F activation at the 2-position to give 13 and FBpin, as well as by C–H activation at the 4-position to give 7 and HBpin. Complex [Rh{4-(3,5-C5F2H2N)}(PEt3)3]6h and 3,5-difluoropyridine were also generated, because 13 reacted further with HBpin. 3,5-Difluoropyridine subsequently gave with [Rh(Bpin)(PEt3)3] the complex [Rh{4-(3,5-C5F2H2N)}(PEt3)3] by C–H activation at the 4-position6h.
-
(a) M. Ahijado, T. Braun, D. Noveski, N. Kocher, B. Neumann, D. Stalke and H.-G. Stammler, Angew. Chem., Int. Ed., 2005, 44, 6947 (
Angew. Chem.
, 2005
, 117
, 7107
) CrossRef CAS PubMed;
(b) G. Meier and T. Braun, Angew. Chem., Int. Ed., 2011, 50, 3280 (
Angew. Chem.
, 2011
, 123
, 3338
) CrossRef CAS PubMed;
(c) G. Meier and T. Braun, Angew. Chem., Int. Ed., 2012, 51, 12564 (
Angew. Chem.
, 2012
, 124
, 12732
) CrossRef CAS PubMed;
(d) M. Ahijado and T. Braun, Angew. Chem., Int. Ed., 2008, 47, 2954 (
Angew. Chem.
, 2008
, 120
, 2996
) CrossRef CAS PubMed;
(e) M. Ahijado Salomon, T. Braun and A. Penner, Angew. Chem., Int. Ed., 2008, 47, 8867 (
Angew. Chem.
, 2008
, 120
, 8999
) CrossRef PubMed.
Footnote |
† Electronic supplementary information (ESI) available: Experimental procedures, additional spectra and computational details. See DOI: 10.1039/c5sc00877h |
|
This journal is © The Royal Society of Chemistry 2015 |
Click here to see how this site uses Cookies. View our privacy policy here.