Cyanobacterial management in full-scale water treatment and recycling processes: reactive dosing following intensive monitoring
Received
23rd November 2015
, Accepted 15th January 2016
First published on 19th January 2016
Abstract
The presence of cyanobacteria within full-scale treatment plants including spent filter backwash water and sludge treatment facilities is an increasing problem faced by water utilities. The fate of cyanobacterial associated taste and odour (T&O) compounds during these processes is unknown. This study aimed to better understand the occurrence of cyanobacteria and T&O compounds, 2-methylisoborneol (MIB) and geosmin, within a full-scale water treatment additional plant with recycling processes and investigate effectiveness of supplementary treatment processes applied within the plant following results of intensive and real time sampling. Samples were obtained from the source water and at key points within the treatment train, including the sludge thickener where MIB was noted to reach approximately 1500 ng L−1. Potassium permanganate and powdered activated carbon were dosed into the sludge thickener and the concentration of MIB producing cyanobacterial cells and MIB in the sludge thickener was reduced by greater than 99%. However, MIB concentrations were still measured at approximately 20 ng L−1 in the finished water, attributed to the addition of water recycled from the sludge thickener. A correlation between total MIB and the sample fluorescence was established and used to guide chemical dosing to maintain low levels. These results demonstrate that in situ fluorescence probes could be used to optimise the application of potassium permanganate and activated carbon when applied to reduce reducing cyanobacteria and MIB concentration during water treatment however further investigation would be required.
Water impact
Presence of cyanobacteria and their associated taste-and-odour (T&O) compounds in water sources, within full-scale treatment plants including spent filter backwash water and sludge, and reuse processes are increasing problems faced by water utilities. Geosmin and 2-methylisoborneol are the main cyanobacterial T&O compounds and can cause complaints from consumers at levels as low as 10 ng L−1. Literature is scarce on the fate of cyanobacteria and T&O compounds in the full-scale processes. This paper provides a significant record of cyanobacterial species and T&O compounds and their removal within a full-scale sludge treatment plant. This paper also identifies the possibility of applying in situ fluorescence measurements to guide chemical dosing to minimise the risk of accumulation of cells/T&O under operational conditions in sludge handling/reuse facilities.
|
1. Introduction
The presence of cyanobacteria in water sources and within treatment plants is an increasing problem faced by water supply managers.1–5 Climate change effects and the associated global warming provide more favourable conditions for cyanobacterial growth in warm and temperate climates leading to more frequent bloom events.6–8 Several cyanobacterial species are potent producers of taste and odour (T&O) compounds and/or toxins.9–11 In drinking water, 2-methylisoborneol (MIB – C11H20O) and geosmin (C12H22O) are two commonly detected cyanobacterial T&O compounds.1,11–14 Extracellular MIB and geosmin are detected by humans at a concentration as low as 10 ng L−1;11,15 and impart an earthy musty taste or odour to water leading to customer complaints.11,13,16,17 Known cyanobacterial genera that produce MIB and/or geosmin include Pseudanabaena, Anabaena, Phormidium and Geitlerinema. While MIB and geosmin do not represent a known risk to public health,11 unpleasant T&O in distributed water invokes the perception that its consumption may be unsafe and results in a loss of customer confidence in the utilities' ability to provide safe drinking water.13 Furthermore, production of anatoxins and microcystins (neurotoxin and hepatotoxin, respectively) by T&O producing species have been documented.14 Hence, identification of the source of T&O producing cyanobacteria in a water supply and the implementation of appropriate management strategies are essential in supplying water that is at risk of cyanobacterial impact.9,18,19
The World Health Organisation (WHO) and other national and local regulatory agencies have alert levels designed to reduce the likelihood of harmful cyanobacterial metabolites entering treated water supply systems.4,9,18–21 However, less is known about the potential risk of cyanobacteria becoming established within the boundary of the water treatment plants (WTPs). While the risk within a WTP might be similar to source water risk,21–23 accumulation of cyanobacterial cells can occur within the WTP resulting in eventual breakthrough.1,4,21 Notably, one study reported cyanobacterial cell counts as high as 2 × 104 cells per mL within the WTP despite a low risk source water with cell counts less than 400 cells per mL.24 The fate of cyanobacterial cells and their metabolites, the potential for cell lysis and cell division and the mechanisms by which accumulation of cells occurs in a WTP is not well understood; especially for WTPs with low risk source water.
The sludge from clarification process, the surface of clarification basins and gravity filters are all potential sites for cell accumulation.1,2,19,23,25–27 The recycling of process water recovered from filter backwashing or sludge dewatering is common in many plants to maximize production; hence, if cyanobacteria are present, storage of the sludge and spent backwash water during the recycling process increases the risk of cyanobacteria accumulation and necessitates appropriate treatment.26,27 While recent studies in limited WTPs have focused on conventional and advanced water treatment processes;1,4,15,19,23 the fate of cyanobacterial T&O compounds within full-scale sludge treatment facilities, the impact on water recovery process, and the appropriate level of sludge and/or spent water treatment require further investigation.15 Literature is scarce on the fate of cyanobacteria and their associated T&O compounds in full-scale sludge handling and water reuse processes.
Potassium permanganate (KMnO4) and powdered activated carbon (PAC) have been successfully applied for the removal of cyanobacteria and their metabolites from water.13,25,28–34 Selection of PAC dose and type of activated carbon depend on the target compounds and on water quality.30,35,36 KMnO4 has been used as an algaecide, with the lethal dose varying from 1 to 10 mg L−1 depending on the species and water quality conditions,37–39 and also as a conditioner to improve coagulation and clarification processes.37 While the application of KMnO4 and PAC has been examined extensively to remove cells and metabolites from raw water;4 investigation on (1) their use in the treatment of a plant's spent backwash water and sludge, and (2) the timing, concentration and optimal location of chemical dosing within the existing WTPs to remove T&O compounds has received far less attention.
The timing of the response to cyanobacteria is a critical factor in the successful management of cyanobacteria and their by-products in WTPs.11,40 Currently, the water industry relies on microscopic analysis with turnaround times up to 5 days.18,41 The unpredictability of cyanobacterial breakthrough events makes real-time detection methods desirable to help to respond to the presence of elevated levels of cyanobacteria using an appropriate chemical and dose.2,40In situ fluorescence measurement tools have been developed for the rapid detection of cyanobacterial specific pigment, i.e. phycocyanin.41–43 These are valuable tools for screening of phytoplankton assemblages and detecting phycocyanin containing cyanobacteria.3,42,44–46In situ fluorescence probes enable real time cost-effective cyanobacterial detection and management including treatment intervention.47–49
The overall objective of this study was to study the fate of cyanobacteria and their T&O compounds within a full-scale water treatment plant focussing in particular on the treatment and recycling of supernatant recovered from spent filter backwash water and sludge dewatering. The specific objectives of this study were to: 1) monitor the breakthrough and accumulation of cyanobacterial cells and total and extracellular MIB and geosmin in a full-scale WTP, its sludge handling/treatment facility and water reuse system, 2) verify the efficacy of coagulation and reactive dosing, that are PAC and KMnO4, for removing cyanobacterial cells, controlling growth of the cells and production/release of their T&O compounds, and 3) assess applicability of an in situ fluorescence probe for real time treatment adjustment. This paper provides a significant record of cyanobacterial species and their removal within a full-scale sludge treatment plant. This paper also identifies the possibility of applying in situ fluorescence measurement to guide operational chemical dosing to minimise the risk of breakthrough and accumulation of cyanobacterial cells under real operational conditions in sludge handling facilities.
2. Material and methods
2.1. Water source and site description
The studied WTP is situated 70 km south east of Melbourne (Victoria, Australia). The study period was from September 2014 to February 2015. Fig. 1 shows a schematic of the treatment train and water recycling procedures at this WTP. The treatment process includes dissolved air flotation filtration (DAFF) for cyanobacterial removal (Table 1). Alum and polydiallyldimethyl ammonium chloride (polyDADMAC) are the primary coagulant and aid respectively, with fixed doses over the study period (Table 1). The filtered water was treated with UV, pH corrected and disinfected with chlorine. During the study period, the treatment plant operated at a constant flow rate of 20 MLD. The plant performance was determined from filtered and floated water turbidity and constantly met water quality targets. Filters were backwashed at 24 hour intervals. Additional filter backwash events were not necessary during the study period.
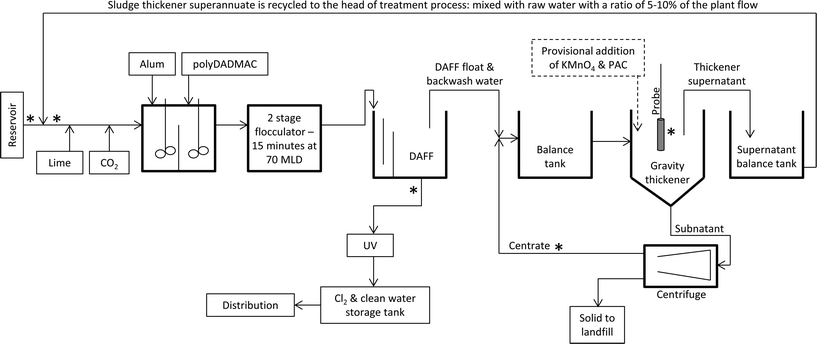 |
| Fig. 1 Schematic of the treatment train, water sampling (*) and probe installation sites. | |
Table 1 Water quality parameters and characteristics of treatment processes used in the studied WTP (average/range from operational records)
Water treatment process |
Water quality & chemical |
Descriptions |
Raw water |
pH |
7.4 |
Temperature (°C) |
20.7 |
DOC (mg L−1) |
6.4 |
Turbidity (NTU) |
37 |
Aluminium sulfate (alum) addition |
Concentration (mg L−1) |
43 (as Al2(SO4)·14H2O) |
polyDADMAC addition |
Concentration (mg L−1) |
0.4 |
DAFF |
Hydraulic retention time (minutes) |
50 |
Solid retention time (hours) |
4 |
Balance tank |
DOC (mg L−1) |
12.3–13.5 |
UV (UV254) |
0.271–0.276 |
Color (HU) |
22–29 |
Turbidity (NTU) |
61–134 |
Sludge handling basin |
Hydraulic retention time (minutes) |
90–160 |
Solid retention time (hours) |
24–48 |
The studied WTP is an example of several plants which use clarification to recover water from spent filter backwash water and sludge. Plant treated water is used for backwash with a chlorine residual less than 0.3 mg L−1. All the water from spent backwash water and DAFF float is treated and recycled to the head of the plant (Fig. 1). The spent filter backwash water and DAFF float are combined in a single balance tank and fed to a gravity thickener which is dosed with polymer to facilitate settling. Sludge thickener supernatant is pumped to the head of the treatment plant prior to any chemical dosing at a ratio of 5–10% of the plant total flowrate (Fig. 1). The underflow from the sludge thickener is dewatered by decanter type centrifuges. The centrifuge centrate is returned to the balance tank and mixed with the DAFF float and spent backwash water (Fig. 1).
2.2. Procedure for water and sludge supernatant sampling in full-scale WTPs
Initially, weekly sampling of the sludge thickener, reservoir and product water was performed from early September to mid November 2014. Sampling records are documented in the plant hazard analysis and critical control points (HACCP) plan. The frequency of sampling was increased to biweekly from mid November 2014 due to increase in concentration of detected T&O compounds. Targeted sampling was also conducted at various locations within the treatment plant to establish plant performance and the distribution of algal/cyanobacterial species throughout the plant as follows: (1) raw water at the plant intake, (2) after addition of sludge thickener supernatant to the raw water prior to any treatment, (3) post DAFF, (4) sludge thickener supernatant, and (5) centrifuge centrate (Fig. 1). The samples were taken at specific times relative to the estimated hydraulic detention times in each treatment process in order to sample, as closely as possible, the same “parcel” of water. All sampling taps were fully flushed prior to sampling. Duplicate samples were obtained to measure total metabolites using 350 mL polyethylene terephthalate bottles with no head space. Samples were immediately placed on ice. Samples were subjected to three sonication cycles prior to analysis for total geosmin and total MIB. Pretests were conducted to confirm the efficacy of these cycles (data not shown). Raw water samples were preserved in Lugol's iodine for species identification and enumeration. This study also included periods of provisional intervention and temporary treatment adjustment as described in section 2.5. The targeted sampling was not conducted during these periods to maintain the consistency of the results.
2.3. Treatment of water within the sludge handling facility: KMnO4 and PAC dosing
2.3.1. Laboratory based jar tests.
Jar tests were performed using influent of sludge thickener for removal of Pseudanabaena cells and extracellular MIB. These tests were used to identify an optimal strategy that was implemented in the full-scale plant. Alum and various PAC dosages (Acticarb PS1300) were used to achieve MIB removal (separate tests for alum and PAC). For alum jar tests 8000 cells per mL, 50
000 cells per mL and 100
000 cells per mL of laboratory cultures Pseudanabaena (isolated from samples from the studied WTP) were spiked into the thickener water samples. In the case of PAC tests 100 ± 10% ng L−1 of MIB was spiked in to the water samples. Two mixing times of 30 minutes and six hours were tested for the jar tests to mimic the estimated minimum and maximum range of contact times available at full scale when dosing PAC into the sludge thickener surface zone.
Additional jar tests to assess treatment intervention for intact cell removal and/or cell lysis in the sludge thickener were conducted. For these tests thickener water sample that contained naturally occurring numbers of cells were used. Water samples were taken before and after the tests to assess cell removal. The mixing time was two hours followed by 22 hours of settling corresponding to the maximum treatment time available for settling with the treatment plant in operation. These tests included four scenarios to enhance coagulation and settling of cells by adding coagulant aid and pre-oxidation, and in one scenario to lyse the cells via pre-oxidation. The details of these jar tests are as follows: (a) sludge thickener water sample containing naturally grown/accumulated cells as control; (b) 150 mg L−1 of bentonite was added to a subsample of the same water as the control; (c) 150 mg L−1 of polyDADMAC was added to a subsample of the same water as the control; (d) 150 mg L−1 bentonite and 50 mg L−1 alum were added to a subsample of the same water as the control; (e) 3.0 ± 0.5 mg L−1 KMnO4, 150 mg L−1 bentonite and 50 mg L−1 alum (as Al2(SO4)·14H2O) were added to a subsample of the same water as the control (samples were taken at predetermined contact times to measure KMnO4 decay); (f) 3.0 ± 0.5 mg L−1 KMnO4 was spiked into a subsample of the same water as the control (KMnO4 decay was measured as well).
2.3.2. KMnO4 and PAC dosing in the full-scale sludge thickener.
Reactive dosing was conducted in two stages. Stage 1 included potassium permanganate (10 ± 0.5 mg L−1) dosing into the sludge thickener on several occasions over the period of 19th December 2014 to 31st December 2014. The focus of this period was to understand the efficacy of KMnO4 on cell removal, lysis and release of MIB. PAC was not added since the plant finished water was not distributed to customers. In order to assess the performance of KMnO4 on cell lysis and because of the time lag between sampling and microscopic cell count results, an in situ probe was installed to continuously monitor the level of cyanobacterial cells in the thickener supernatant during the spiking period. The frequency of dosing was adjusted according to the raw fluorescence probe reading. The probe measurement in the thickener supernatant with very low cyanobacterial cell counts was set as the baseline. When the probe in situ measurement reached 10% of the baseline threshold, KMnO4 was dosed. The required frequency ranged from every 24 hours to fortnightly. Stage 2 included simultaneous dosing of KMnO4 (10 ± 0.5 mg L−1) and PAC (20 ± 0.5 mg L−1) from 14th January 2015 to 17th January 2015. Probe was not used for in situ fluorescence measurements during stage 2. The dosing locations are shown on Fig. 1. Potassium permanganate was added in two stages. The permanganate solution was dosed into the central feed well while the thickener feed pump was operating to treat water in the middle to lower depth of the thickener. An hour after the initial dosing, the thickener feed pumps were manually stopped and the surface of the sludge thickener was dosed. Compressed air was used to disperse the KMnO4 and applied for approximately 60 minutes. Good mixing was confirmed by visual observation of a change in colour. The thickener was kept offline for approximately 5 hours to allow sedimentation of the MnO2 and cyanobacterial floc. This was the maximum time before the process was required to restart to treat the next filter backwash. Potassium permanganate solutions were prepared by adding 3.5 kg of potassium permanganate (97.5% w/w, Orica Australia) into 200 L of filtered water. The dosing of the tank took ∼10 minutes. PAC was dosed in a similar fashion to KMnO4. Slurry of 1.75% w/w was prepared by adding 3.5 kg of powdered activated carbon (Acticarb PS1300) to 200 L of filtered water. The slurry was mixed into the thickener and kept in suspension for 60 minutes followed by a 4 hour period of quiescence to encourage settling. KMnO4 decay (initial dose of 10 mg L−1) was also assessed in the sludge thickener. Samples were taken at the dosing location prior and post dosing (at the predetermined time intervals) for analysis.
2.4.
In situ fluorescence monitoring
An online YSI EXO2 water-quality multi-probe (YSI, Yellow Springs, Ohio, USA) fitted with in situ phycocyanin fluorescence (Blue Green Algae probe) was used in this study.50 The probe was installed in the sludge thicker within the top 30 cm of surface water (Fig. 1) at the same location from which the supernatant sample was collected. In situ probe measurements were recorded every 15 min. The phycocyanin probe excites the cyanobacterial phycocyanin at 590 nm (with a pass-band of 565–605 nm) and measures the emission of the pigment at 660 nm. The probe detects light emitted in the range of 640–680 nm. The raw readings of the phycocyanin probe are presented in relative fluorescent units (RFUs). The probe resolution, range of reading, detection limit, and limit of quantification are 0.1 RFU, 0–100 RFU, 0.2 RFU and 0.7 RFU, respectively. The highest detection limit of the EXO2 phycocyanin probe is 250
000 cells per mL. Thus, cell counts exceeding 200
000 cells per mL cannot be considered reliable. The online probe monitoring results were reviewed and compared against cell identification/quantification to establish the relationship between cell counts and in situ fluorescence measurement results.
2.5. Analysis of geosmin and MIB and other water quality parameters
All analyses were conducted in triplicate for each sample. Water samples for geosmin and MIB analyses were concentrated using a solid phase micro extraction (SPME) syringe fibre (Gerstel) and analysed on a 7890A Gas Chromatograph System with 5975C VL Series Mass Selective Detector (Agilent Technologies) against quantified labelled internal standards (Ultrafine Chemicals).51,52 The injection port was operated in split less mode with temperature controlled at 250 °C. The oven temperature was held at 60 °C for 0.5 min, increased to 100 °C at 30 °C min−1, followed by an increase at a rate of 20 °C min−1 to 185 °C. Then, the oven temperature was further increased to 250 °C at a rate of 40 °C min−1, and held for 2.30 min.52 The reporting limits for MIB and geosmin were 4 and 2 ng L−1, respectively. Geosmin and MIB were purchased from a commercial supplier (Ultrafine Chemicals) and were used for the analytical method.
Algal/cyanobacterial species identification and enumeration on samples preserved in Lugol's iodine were conducted using a compound microscope in a Sedgewick-Rafter counting chamber.53 TOC and dissolved organic carbon (DOC) measurements were conducted on an 820 total organic carbon analyser (Sievers Instruments Inc.). Measurements of UV absorbance at 254 nm (UV254) were carried out on a UV-1201 UV/vis Spectrophotometer (Shimadzu Corp.). Prior to DOC and UV analyses, samples were filtered through 0.45 μm membrane filters.
3. Results and discussion
Results of this research project are presented as follows:
1. Discussing the outcome of the sampling campaign within the WTP to map the scale of the problem (corresponding section: 3.1).
2. Investigating the results of the targeted sampling to identify the suitable additional treatment barriers, the timing and location of their application (corresponding section: 3.2).
3. Laboratory scale investigation of treatment option to select the most suitable treatment option (corresponding section: 3.3.1).
4. Presenting the outcome of implementation of the selected treatment barriers (corresponding section: 3.3.2).
3.1. Discovering cyanobacterial challenges from source to recycle stream: accumulation and T&O issues
Cyanobacterial genera found in water samples from the source supplying the studied WTP included Aphanocapsa, Aphanothece, Cyanodictyon, Microcystis, Oscillatorian, Planktolyngbya, Pseudanabaenaceae, Pseudanabaena and Radiocystis. The maximum recorded total cyanobacterial cell count in all river samples was less than 104 cells per mL (Fig. 2). Furthermore, the average and median total cyanobacterial cell number in the river was below 2000 cells per mL (Fig. 2), with all MIB-producing Pseudanabaena less than 103 cells per mL. This is lower than the alert level proposed by the WHO (2000 cells per mL), indicating that the source is not deemed to be at high risk of cyanobacterial proliferation.9 The source water is directed into a reservoir prior to treatment. The list of cyanobacterial genera recorded in samples taken from the reservoir includes: Aphanocapsa, Aphanothece, Cyanodictyon, Microcystis, Phormidium, Planktolyngbya, Oscillatorian, Pseudanabaena and Synechocystis. The average total cyanobacterial cell number in the reservoir was 2700 cells per mL with all MIB-producing Pseudanabaena cell count results always below 103 cells per mL (Fig. 2). MIB or geosmin has not been detected in routine source water sampling. The dynamics of the cyanobacterial community and their metabolites, for example T&O compounds, in the source water and reservoir has been consistent between sampling years and seasons. The consistency in species present over long periods of time, over 20 years, has been observed in Australian water bodies.11,41
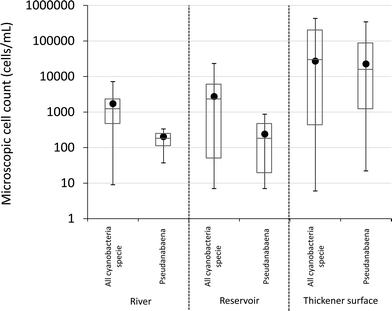 |
| Fig. 2 All available data regarding total cyanobacteria and MIB-geosmin producing cyanobacteria species in the river (source), reservoir and sludge thickener of the studied plant (the box plots display the distribution of data based on the minimum, first quartile, median, third quartile, and maximum values of the data; the black circle is the average values of the data). | |
Following established cyanobacterial management procedures, weekly cyanobacterial sampling was conducted at the water intake and surface of gravity thickener once the 2000 cells per mL was attained. In September 2014, total cyanobacteria and Pseudanabaena cell numbers started to increase in samples taken from the surface of the sludge thickener (data not shown). Similar to the source water data, all available data regarding total cyanobacteria and T&O producing Pseudanabaena in the sludge thickener were compiled (Fig. 2). Average and median cell numbers were consistently greater than 104 cells per mL with 4.3 × 105 and 3.4 × 105 cells per mL as maximum total cyanobacteria and Pseudanabaena cell numbers (Fig. 2). These total cyanobacteria and Pseudanabaena cell numbers surpassed the highest WHO source alert level which is 105 cells per mL.9 Notably, the concentration of Pseudanabaena cells in the settled supernatant was on average 100 times greater than the raw water (Fig. 2). The discrepancy between the number of cyanobacterial cells and in particular the MIB-producing Pseudanabaena between the source water and sludge thickener (Fig. 2) demonstrates accumulation of cells within the recycle process. Similar situations have been reported in two south eastern Canadian WTPs where cyanobacterial numbers in the source were low (cells numbers always below 400 cells per mL) and yet Microcystis cells accumulated from 2600 to 3.2 × 104 cells per mL in the sludge bed and clarified water.24
A small but steady increase was observed in concentration of MIB and geosmin in thickener supernatant from early October 2014 (Fig. 3a by early November 2014, while geosmin concentration decreased, MIB measurements showed a major increase reaching a peak of 1500 ng L−1 by mid-December 2014 (Fig. 3a). In early December 2014, the MIB concentration in the supernatant was 200 ng L−1 and MIB was first detected in treated water (Fig. 3a). The MIB concentration in the treated water and supernatant follow a similar pattern due to water recovery practice implemented in this plant (Fig. 1). Although the supernatant recycle to the raw water is less than 10% of the plant flowrate and is diluted, the MIB concentration in the supernatant was high enough to surpass the plant's capacity to remove MIB. Hence, the MIB concentration peak in finished water corresponds to the MIB peak in thickener supernatant (Fig. 3a).
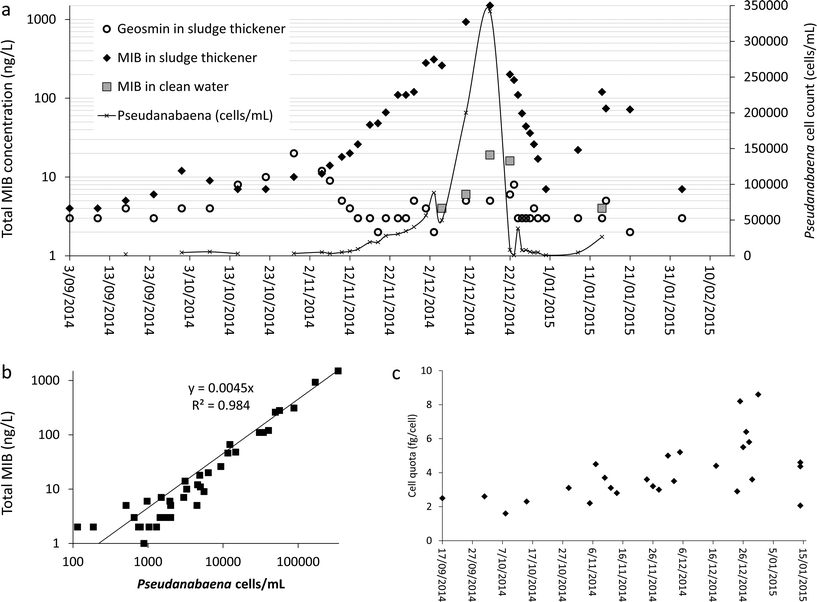 |
| Fig. 3 (a) Total MIB and total geosmin concentration (in sludge thickener and clean water storage water samples), Pseudanabaena cell numbers and in situ probe readings (in sludge thickener water samples) in the studied period, (b) correlation between total MIB measurements and Pseudanabaena cell counts in sludge thickener samples, and (c) total MIB cell quota calculation in samples from the sludge thickener. | |
The significant increase in MIB concentration in the sludge thickener (Fig. 3a) corresponds to the increase in Pseudanabaena cell numbers with a strong linear correlation between the two parameters (Fig. 3b). While the MIB concentration in the sludge thickener reached its maximum measured value, the total cyanobacteria cell count reached >2 × 105 cells per mL with Pseudanabaena dominance. Hence, the MIB production per Pseudanabaena cell also known as cell quota is estimated at 4.4 ± 2.1 fg per cell (Fig. 3c). The cell quota deviation value (47%) is within the published deviation range (16% to 290%) for cell quota estimates of cyanobacterial cellular metabolites and pigments.54 However, the deviation in MIB cell quota (MIB concentration per cell) estimate demonstrates the need for further systematic studies to improve the accuracy and precision of the estimation process and the cell quota value.
3.2. Implications of the targeted sampling campaign: understating fate of cells within the plant
On average, 89% of total cyanobacterial phyla were removed by the DAFF system (Table 2). The speciation analyses demonstrated that Pseudanabaena was removed to below detection limit and Planktolyngbya was the only cyanobacterial genera that broke through the DAFF process (Fig. 4). Yap et al.55 demonstrated that 55 mg L−1 of alum (as Al2(SO4)·14H2O) was required to achieve 99% removal of cyanobacterial species Microcystis aeruginosa using conventional DAF process. Species present, water pH, coagulant/flocculent type and dose, conditions of rapid mix, flocculation and flotation including the bubble concentration have been identified as the influencing factors during algal cell removal using DAF systems.56,57 Similar removal efficiencies were observed for the removal of green algae and diatoms (Table 2) compared to the total cyanobacterial phyla. Notably, in a North American full-scale plant Aphanizomenon was the only genera that broke through the clarification and filtration process, with a minimum 85% removal, while Pseudanabaena, Anabaena and Microcystis were removed to below detection limit.19 Furthermore, this study helped to quantify the scale of cell accumulation within sludge thickener and centrifuge during water recovery (Table 2). Cyanobacterial species demonstrated the highest cell accumulation within the thickener and centrifuge (Table 2). Further studies are warranted to shed light on whether the low accumulation rates observed for green algae and diatoms are due to higher sensitivity to hydraulic pressure, loss of cell integrity, slower growth rates under prevailing conditions in the plant or a higher cell removal on account of more rapid sedimentation.
Table 2 Percentage of cell removal(r) or accumulation(a) (compared to cell numbers at the head of the plant post addition of recycled portion) during the studied water treatment processes (the targeted sampling results)
Process |
Pseudanabaena
|
Cyanophyceae (total cyanobacteria) |
Chlorophyceae (green algae) |
Bacillariophyceae (diatoms) |
DAFF |
100%(r) |
89%(r) |
74%(r) |
100%(r) |
Thickener |
−1400%(a) |
−406%(a) |
−195%(a) |
−25%(a) |
Centrifuge |
NA |
−2599%(a) |
−29%(a) |
−3%(a) |
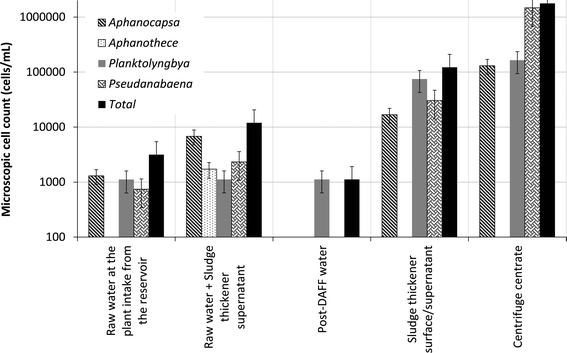 |
| Fig. 4 Cyanobacterial speciation and average cell count within the studied treatment plant (targeted sampling campaign for the period of study). | |
The results of the targeted sampling for the period of this study provides additional evidence that the recycle of sludge thickener supernatant contributes significantly to the increase in cell numbers prior to DAFF treatment process (Fig. 4). Particularly, the accumulation of Pseudanabaena and cyanobacterial cells in the centrifuge centrate, recycle of the centrate to the head of thickener and return of the thickener supernatant to the head of the plant contribute to (a) increase of cell numbers (Fig. 4) and (b) higher risk of MIB release prior to DAFF. These results demonstrate that the current operation of the gravity sludge thickener (Fig. 1) is not adequate for complete removal of all cyanobacterial cells particularly MIB producing Pseudanabaena cells. In summary, this plant recycles a high percentage of cyanobacterial cells to the head of the treatment process. The overall impact on treated water quality is potentially compensated by the ability of DAFF to treat water containing high cell counts. The filtered water UV transmittance was maintained above 90% for the duration of the study period.
These results demonstrate the importance of understanding the fate of cyanobacterial species that could result in potential break through and/or accumulation of cells or metabolites. While recycling the recovered water from the sludge provides the opportunity to improve the efficiency of water production, the handling and treatment of sludge has proved to be an operational challenge.26,27 Exposed sludge handling basins or lagoons are another site for cell accumulation. The residence times of sludge and decanted water in these lagoon systems are long providing opportunity for growth and lysis.1,15,26,27 Furthermore, an increase in cell biomass can cause other operational challenges, such as higher consumption of chemical coagulants, increased sludge production, inefficient flocculation and filter clogging, and increased chlorine demand.1,19 These issues were not apparent in this study however. Variations in the characteristics of algogenic organic matter between cyanobacterial species, green algae and diatoms can also influence the performance of coagulation and DAF processes for removal of these phytoplankton;56–59 hence, the importance of identification of all present species and their fate during the treatment.
Based on the increasing concentration of MIB in the sludge thickener supernatant in early December, the water utility made the decision to disconnect the plant from supply but continued to operate the plant. This eliminated the risk of supplying water to customers with unacceptable taste and odour while providing the opportunity to trial different treatment intervention/adjustment techniques under normal operating conditions. Ideally, any intervention developed to manage cyanobacterial levels should allow the plant to continue supplying water.
3.3. Treatment of water within the sludge handling facility
3.3.1. Laboratory based investigations.
Pseudanabaena cell number values of over 50
000 cells per mL with optimal coagulation conditions required an alum dose of 60 mg L−1 (as Al2(SO4)·14H2O) to achieve >95% cell removal (Fig. 5a). Similarly, jar test results (6 hours long experiments) published by Drikas et al.60 identified 65–70 mg L−1 as the optimal alum dose for removal of over 70% of cyanobacteria cells (106 cells per mL of cultured Microcystis aeruginosa). These results demonstrate that alum addition to the influent of the gravity thickener could enhance the settling and removal of cells. However, alum binding process might vary based on species present, between natural and cultured cells, and in real-world environmental conditions affecting its performance for cell removal during plant operation.60
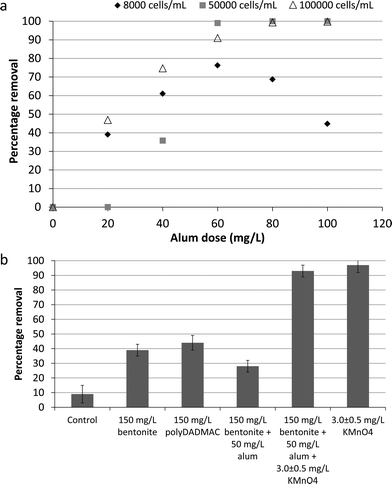 |
| Fig. 5
Pseudanabaena cell removal (a) as a function of alum dose using laboratory cultured cells spiked in the sludge thickener water sample, and (b) after 24 hours of exposure to different treatment scenarios using the thickener water sample that contained naturally grown/accumulated cells. | |
Spiking the thickener influent with bentonite and polyDADMAC in addition to alum in order to enhance the settling process in the thickener water with naturally grown/present cyanobacterial cells was also studied (Fig. 5b). However, less than 50% removal was observed using these treatment adjustment options (Fig. 5b). Therefore, KMnO4 was trialled using a dose of 3.0 ± 0.5 mg L−1 to enhance the coagulation (Fig. 5b). In this case, similar cell removal results (95 ± 2%) were obtained with KMnO4 (initial dose of 3.0 ± 0.5 mg L−1 and 1.3 ± 0.5 mg L−1 residual after 2 hours) in the presence and absence of coagulant and aid-coagulant agents (Fig. 5b). Pre-oxidation by ozone and permanganate potassium to breakdown cyanobacterial/algal cell-bound organic matter have been shown to enhance the coagulation and clarification of cells.56,61 However, the results of this study indicates similar and even better removal in absence of coagulant compare to its presence, due to cell lysis caused by the oxidative effect of KMnO4 and/or addition of MnO2.25,38,39,62 These results showed that the required mass of bentonite to achieve over 90% cell removal would be large increasing the solid disposal cost. Furthermore, alum or cationic polymer alone suffered from inadequate mixing intensity and time hence resulted lower than 45% cell removal. Conversely, the addition of a higher KMnO4 dose is more easily integrated into the existing treatment process and offers the potential of very high cell removal.
Table 3 (non-italic columns) demonstrates that 10 mg L−1 of virgin PAC and 30 minutes of contact reduces the MIB concentration by 42% (initial concentration = 100 ± 10% ng L−1) in laboratory jar tests, while 73% was removed with a PAC dose of 20 mg L−1 and 30 minutes contact. A minimum dose of 50 mg L−1 of PAC and 6 hours of contact time was required to remove 100 ng L−1 of MIB to below detection limits (Table 3: non-italic columns). Several studies have demonstrated the effectiveness of PAC for removal of cyanobacterial/algal released T&O compounds.29,31,34 While Jung et al.31 published similar range of removal percentages for treatment of MIB using virgin PAC, data from Table 3 differ to results presented by Kim et al.34 These authors34 found that virgin coarse PAC dose of 15 mg L−1 was sufficient to reduce the concentration of MIB (initial concentration: 50 ng L−1) below odor threshold concentration. The type of PAC, and the concentration and characteristics of the natural organic matter and their competitive effect, strongly affect the efficiency of PAC for removal of MIB.29,36,63,64 Application of KMnO4 and the consequent cell membrane damage due to oxidant impact and release of cell-bound compounds is a major concern with direct oxidation of cells.62,65 For this reason, the simultaneous addition of PAC was trialled in this study.
Table 3 Removal of total MIB by PAC in jar tests (non-italic columns) and at full scale (italic columns)
PAC dose (mg L−1) |
% removal of MIB after 30 minutes |
% removal of MIB after 6 hours |
% removal in sludge thickener 14th January 2015 (60 minutes)
|
% removal in sludge thickener 15th January 2015 (60 minutes)
|
% removal in sludge thickener 16th January 2015 (60 minutes)
|
10 |
41.6 |
74.0 |
—
|
—
|
—
|
20 |
72.9 |
91.2 |
21.8
|
20.0
|
21.0
|
30 |
79.5 |
93.3 |
—
|
—
|
—
|
50 |
94.9 |
100 |
—
|
—
|
—
|
70 |
96.3 |
99.2 |
—
|
—
|
—
|
100 |
100.0 |
100 |
—
|
—
|
—
|
3.3.2. Reactive dosing in the full-scale plant and implication of in situ monitoring.
Stage 1 (only KMnO4 dosing).
Enhancing cell removal within the sludge gravity thickener was identified as essential to control (a) the likelihood of MIB breakthrough (Fig. 3a) and (b) recycle of MIB producing Pseudanabaena cells within the plant (Fig. 4). To achieve this goal and following the results of the laboratory based studies, KMnO4 dosing was implemented into the sludge thickener (data presented for the period of 19th December 2014 to 27th December 2015 – Fig. 6). Fig. 6 demonstrates that for a 10 mg L−1 KMnO4 dose, the oxidant residual in the water would persist for around 15 hours providing a reasonable contact time to achieve cell lysis. The microscopic analysis of water samples demonstrates 13–98% reduction in total cyanobacterial and Pseudanabaena cell numbers due cell lysis caused by KMnO4 spiking during this period (Fig. 6 and Table 4: non-italic rows). However, chlorophyceae and other micro algae phyla that were present in the water demonstrate a stronger resistance to oxidation by KMnO4 (Table 4: non-italic rows). Thus, an increase in number of Chlorophyceae was observed post-KMnO4; however these observations highlight the need for further investigation to understand the cell damage and lysis process, release of intracellular organic matter, and the ratio of low and high molecular weight organic matter during oxidation with KMnO4. The cell lysis due to KMnO4 oxidation and settlement reduced the number of cells and cell-bound MIB whilst the measured extracellular MIB concentration was similar to total MIB prior to the dosing as KMnO4 does not oxidize MIB. Fan et al.,62 documented loss in membrane integrity of the entire 7 × 105 cells per mL of Microcystis aeruginosa using 10 mg L−1 of KMnO4 with 6 hours of contact with a slow (lower than 20 M−1 s−1) rate of intracellular compounds release. However, these authors conducted their experiment using laboratory cultured cells (unicellular or double cells) spiked in ultrapure water; in operational conditions the presence of other compounds in water and cellular agglomerates could influence the efficiency of the oxidant agent.39,56,57 Also pre-oxidation by permanganate could enhance the sedimentation of the cell within the gravity thickener which would increase the fraction of cells removed from the process via solid waste.61
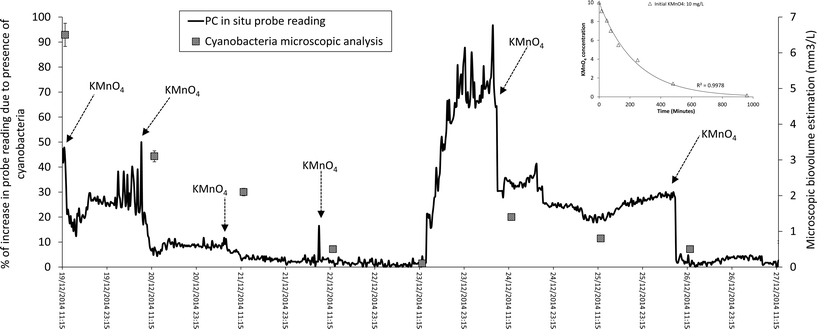 |
| Fig. 6 KMnO4 spiking to control T&O producing cells in gravity sludge thickener, application of in situ fluorescence monitoring technique to adjust the spiking timing and intensive sampling to verify the efficiency of the applied treatment (right hand side graph: KMnO4 decay curve). | |
Table 4 Reduction in Pseudanabaena, total cyanobacteria and green algae cell number by permanganate following dosing of KMnO4 into the sludge thickener
Date |
Removal of Pseudanabaena |
Removal of total cyanobacteria |
Removal of chlorophyceae |
Removal of other micro algae phyla |
19/Dec/14 |
79% |
92% |
89% |
91% |
20/Dec/14 |
39% |
44% |
−1829% |
−415% |
21/Dec/14 |
90% |
59% |
63% |
61% |
22/Dec/14 |
90% |
98% |
90% |
96% |
24/Dec/14 |
80% |
45% |
−2127% |
24% |
26/Dec/14 |
30% |
21% |
−220% |
−35% |
14/Jan/15
|
61%
|
50%
|
93%
|
56%
|
15/Jan/15
|
13%
|
94%
|
−147%
|
88%
|
16/Jan/15
|
45%
|
13%
|
−452%
|
−103%
|
Recently in situ fluorescence monitoring probes have been used for monitoring cyanobacterial presence in clarified, filtered and chlorinated water.24,40 However, to the authors' knowledge this is the first documented application of these probes to monitor sludge handling and water reuse facilities within WTPs. The adjustment of KMnO4 dosing using probe measured fluorescence (Fig. 6) is a novel operational approach. Despite the success of using the probe to monitor the cyanobacterial numbers in this instance, several important questions are raised with respect to the impact of (a) KMnO4 residual, (b) water quality at the thickener, and (c) cell numbers and probe's highest limit of detection, on probe accuracy and performance. Several sources of interference including presence of chlorophyll-a from other phytoplankton, water turbidity and light scattering particles, varying cyanobacterial cell sizes and cellular agglomerations, and optic probe function/calibration, have been associated with in situ fluorescence measurements.41,45,66 Thus, the interpretation of data recorded by in situ fluorescence probes under varying water quality conditions and their application to trigger action, particularly in conditions that are appreciably different to natural environments (for example inside the sludge thickener), is a real challenge and requires further investigation.2,40,45 Therefore, further systematic studies would be necessary to answer these questions and to provide the knowledge support to implement this practice at other plants. Setting an in situ fluorescence limit for process monitoring and treatment adjustment using the correlation between in situ phycocyanin monitoring and cyanobacterial cell count/biovolume estimation and T&O compounds cell quota (similar to Fig. 3c) is the topic of an on-going investigation.
In order to assess the in situ probe performance the probe measurements in RFU were plotted against the biovolume of cyanobacteria, which was dominant by Pseudanabaena, estimated using the reference microscopic method (Fig. 7). A linear correlation with an R2 of 0.7 was observed between the probe measurement sand the reference method values. Similar correlation and R2 was observed for probe readings within a full scale WTP in Canada.40 Notably, these R2 values are higher than observed correlation factors from data collected in freshwater sources;45,46,48 which could be due to the fact that the water within a plant follows a conditioned environment with more controlled parameters compared to natural environment.40
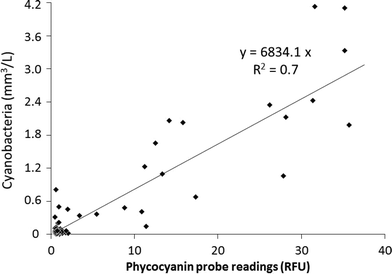 |
| Fig. 7 Phycocyanin (PC) containing cyanobacteria in situ monitoring using the fluorescence probe versus cyanobacterial taxonomy results (Pseudanabaena was identified as the dominant species). | |
Stage 2 (simultaneous KMnO4 and PAC dosing).
In early January 2015 an increase in MIB concentrations within the thickener (Fig. 3a) was observed while geosmin concentrations stayed below 5 ng L−1. From 31st December 2014 (MIB: 7 ng L−1) to 8th January 2015 (MIB: 22 ng L−1) the measured MIB values tripled; and on the morning of 14th January 2015 reached a maximum of 120 ng L−1 with total cyanobacterial cell number measured at 370
000 cells per mL. Thus, the treatment adjustment program was re-launched with KMnO4 spiking for a period of 72 hours. Additionally in order to prevent the breakthrough of extracellular MIB to treated water PAC was also spiked to the water. In situ probe measurements were not used during this period as addition of PAC would interfere with the probe readings. Total cyanobacterial cell numbers after the 72 hours of intervention dropped to below 10
000 cells per mL. In average 40% and 52% of Pseudanabaena and total cyanobacterial cells were lysed per day during the three day intervention, respectively (Table 4: italic rows). PAC dosing also helped to remove in average 21% of the extracellular MIB (Table 3: italic columns). 20 mg L−1 of PAC with double contact time (60 minutes) was successful in removing about 75% less MIB in the full-scale thickener (Table 3: italic columns) compared to the laboratory assays (Table 3: non-italic columns). During the laboratory assays (Table 3: non-italic columns) MIB was spiked into the water in 100% extracellular form, while within the full-scale thickener (Table 3: italic columns) MIB is in both intracellular and extracellular form. The ratio of intra/extracellular compounds has a significant impact of efficiency of PAC for removal of cyanobacterial/algal T&O compounds.11,67–69 High concentrations (over 60 ng L−1) of cyanobacterial T&O compounds were detected in water post-addition of PAC in South Australian plants due to the variations in this ratio.15 The ratio of intra/extracellular T&O compounds could be influenced by (a) conditions of the water body, (b) species present, (c) age of producing cells, (d) environmental conditions within the sludge handling basins, and (e) hydraulic stress during sludge pumping.11,15,67,69 While this study only focused on total MIB, the ratio of intra/extracellular MIB and the fate of the cells and their associated compounds within the sludge handling facility require further investigation.
4. Conclusions
• The potential for accumulation and growth of T&O producing cyanobacteria in a treatment plant does not necessarily correspond with the measured source (river and reservoir) risk. This is an issue related to design and operation of the water treatment plant and the fate of cells within the recovery/recycle/sludge management processes. The hypothetic growth of cells within sludge requires a detailed systematic study.
• A relationship between recycling of sludge thickener supernatant and finished water quality was observed. For this plant, a MIB concentration in the sludge supernatant greater than 200 ng L−1 resulted in MIB being detected in treated water. Due to very high concentrations in the recycled supernatant the plant was unable to reduce MIB concentration to below 10 ng L−1 odour threshold.
• Accumulation of cells and their associated T&O compounds were observed in sludge thickener and sludge centrifuge sampling. Even though MIB producing Pseudanabaena cells were removed by DAFF, the release of cell-bound MIB, breakthrough of extracellular MIB into filtered water and inefficiency of oxidation with UV and/or chlorination for MIB removal led to the detection of T&O compounds in the treated water. Notably, Planktolyngbya was the only cyanobacterial genera that broke through the DAFF process.
• Data from monitoring in the studied operational WTP demonstrate that (1) the sludge supernatant recovery ratio (the ratio at which the supernatant is added to the raw water) during recycle process and (2) the ratio of extra/intracellular compounds in sludge supernatant can play key roles in determining the efficiency of removal of T&O compounds by the treatment plant, including activated carbon where it is dosed. Furthermore, understating characteristics of reactions which cause cell damage and release of intracellular metabolites during oxidation requires further investigation.
• These results provide treatment plant operators a guide to optimise the application of potassium permanganate at reducing the number of MIB producing cyanobacteria during water recovery. Applicability of in situ fluorescence measurements to implement a reliable monitoring and treatment strategy require further investigation.
Acknowledgements
Sampling and data collection was supported by Melbourne Water. Data analysis was supported by Australian Research Council Linkage Project (LP1301000330 which included support from SA Water, Seqwater, Melbourne Water, WaterNSW, NSW Office of Water, Water Corporation and WaterRA) and the research team at UNSW and SA Water – AWQC. The authors wish to express their gratitude to the operational staff at studied WTP including Aaron Ward, Josh Rogers, Gary Anderson, Neveille Whitehead and Mick Ohalloran for sampling support and Dr David Hill from University of Melbourne for isolation, identification and maintenance of cell cultures.
References
-
S. Kommineni, K. Amante, B. Karnik, M. Sommerfeld and T. Dempster, Strategies for Controlling and Mitigating Algal Growth within Water Treatment Plants, Water Research Foundation, Denver, Colorado, USA, 2009 Search PubMed.
- W. Schmidt, H. Petzoldt, K. Bornmann, L. Imhof and C. Moldaenke, Use of cyanopigment determination as an indicator of cyanotoxins in drinking water, Water Sci. Technol., 2009, 59(8), 1531–1540 CrossRef CAS PubMed.
-
American Water Works Association (AWWA), Algae source to treatment, AWWA M57, American Water Works Association, Denver, Colorado, USA, 2010 Search PubMed.
-
A. Zamyadi, Emerging toxic cyanobacterial issues in freshwater sources: Influence of climate change, In: Seafood and Freshwater Toxins: Pharmacology, Physiology, and Detection, ed. L. M. Botana, Taylor & Francis, USA, 3rd edn, 2014, ch. 5 Search PubMed.
- L. C. Bowling, S. Blais and M. Sinotte, Heterogeneous spatial and temporal cyanobacterial distributions in Missisquoi Bay, Lake Champlain: An analysis of a 9 year data set, J. Great Lakes Res., 2015, 41, 164–179 CrossRef.
- C. Wiedner, J. Rucker, R. Bruggemann and B. Nixdorf, Climate change affects timing and size of populations of an invasive cyanobacterium in temperate regions, Oecologia, 2007, 152(3), 473–484 CrossRef PubMed.
- J. A. Elliott, Is the future blue-green? A review of the current model predictions of how climate change could affect pelagic freshwater cyanobacteria, Water Res., 2012, 46(5), 1364–1371 CrossRef CAS PubMed.
- H. W. Paerl and V. J. Paul, Climate change: links to global expansion of harmful cyanobacteria, Water Res., 2012, 46(5), 1349–1363 CrossRef CAS PubMed.
-
I. Chorus and J. Bartram, Toxic Cyanobacteria in Water: a Guide to Their Public Health Consequences, Monitoring and Management, World Health Organization (WHO), London, United Kingdom, 1999 Search PubMed.
- W. W. Carmichael, S. M. F. O. Azevedo, J. S. An, R. J. R. Molica, E. M. Jochimsen, S. Lau, K. L. Rinehart, G. R. Shaw and G. K. Eaglesham, Human fatalities from cyanobacteria: chemical and biological evidence for cyanotoxins, Environ. Health Perspect., 2001, 109, 663–668 CrossRef CAS PubMed.
-
P. Hobson, C. Fazekas, J. House, R. Daly, T. Kildea, S. Giglio, M. Burch, T.-F. Lin and Y.-M. Chen, Tastes and odours in reservoirs – Research report 73, Water Quality Research Australia, Adelaide, Australia, 2010, p. 96 Search PubMed.
- I. H. Suffet, D. Khiari and A. Bruchet, The drinking water taste and odor wheel for the millennium: beyond geosmin and 2-methylisoborneol, Water Sci. Technol., 1999, 40(6), 1–13 CrossRef CAS.
-
K. M. Smith, Characterization of activated carbon for taste and odour control, M.A. Sc. thesis, University of Toronto, Canada, 2011, p. 136 Search PubMed.
- S. Suurnakki, G. V. Gomez-Saez, A. Rantala-Ylinen, J. Jokela, D. P. Fewer and K. Sivonen, Identification of geosmin and 2-methylisoborneol in cyanobacteria and molecular detection methods for the producers of these compounds, Water Res., 2015, 68, 56–66 CrossRef CAS PubMed.
- A. Zamyadi, R. Henderson, R. Stuetz, R. Hofmann, L. Ho and G. Newcombe, Fate of geosmin and 2-methylisoborneol in full-scale water treatment plants, Water Res., 2015, 83, 171–183 CrossRef CAS PubMed.
- J. Ridal, B. Brownlee, G. McKenna and N. Levac, Removal of taste and odour compounds by conventional granular activated carbon filtration, Water Qual. Res. J. Can., 2001, 36(1), 43–54 CAS.
- Y. R. Rao, M. G. Skafel, T. Howell and R. C. Murthy, Physical processes controlling taste and odour episodes in Lake Ontario drinking water, J. Great Lakes Res., 2003, 29(1), 70–78 CrossRef.
-
G. Newcombe, International Guidance Manual for the Management of Toxic Cyanobacteria, Global Water Research Coalition and Water Quality Research Australia, London, 2009 Search PubMed.
- A. Zamyadi, S. Dorner, S. Sauvé, D. Ellis, A. Bolduc, C. Bastien and M. Prévost, Species-dependence of cyanobacteria removal efficiency by different drinking water treatment processes, Water Res., 2013, 47, 2689–2700 CrossRef CAS PubMed.
- S. Merel, D. Walker, R. Chicana, S. Snyder, E. Baurès and O. Thomas, State of knowledge and concerns on cyanobacterial blooms and cyanotoxins, Environ. Int., 2013, 59, 303–327 CrossRef CAS PubMed.
- C. Quiblier, S. Wood, I. Echenique-Subiabre, M. Heath, A. Villeneuve and J.-F. Humbert, A review of current knowledge on toxic benthic freshwater cyanobacteria: Ecology, toxin production and risk management, Water Res., 2013, 47, 5464–5479 CrossRef PubMed.
- S. Merel, M. C. Villarín, K. Chung and S. Snyder, Spatial and thematic distribution of research on cyanotoxins, Toxicon, 2013, 76, 118–131 CrossRef CAS PubMed.
- A. Zamyadi, S. L. MacLeod, Y. Fan, N. McQuaid, S. Dorner, S. Sauvé and M. Prévost, Toxic cyanobacterial breakthrough and accumulation in a drinking water plant: a monitoring and treatment challenge, Water Res., 2012, 46, 1511–1523 CrossRef CAS PubMed.
- A. Zamyadi, S. Dorner, M. Ndong, D. Ellis, A. Bolduc, C. Bastien and M. Prévost, Low-risk cyanobacterial bloom sources: Cell accumulation within full-scale treatment plants, J. - Am. Water Works Assoc., 2013, 105(11), E651–E663 CrossRef.
- W. Schmidt, H. Willmitzer, K. Bornmann and J. Pietsch, Production of drinking water from raw water containing cyanobacteria: Pilot plant studies for assessing the risk of microcystin breakthrough, Environ. Toxicol., 2002, 17(4), 375–385 CrossRef CAS PubMed.
- L. Ho, J. Dreyfus, J. Boyer, T. Lowe, H. Bustamante, P. Duker, T. Meli and G. Newcombe, Fate of cyanobacteria and their metabolites during water treatment sludge management processes, Sci. Total Environ., 2012, 424, 232–238 CrossRef CAS PubMed.
- L. Ho, A. Barbero, J. Dreyfus, D. R. Dixon, F. Qian, P. J. Scales and G. Newcombe, Behaviour of cyanobacterial bloom material following coagulation and/or sedimentation, J. Water Supply: Res. Technol.--AQUA, 2013, 62(6), 350–358 CrossRef CAS.
- S. Lalezary, M. Pirbazari, M. Dale, T. Tanaka and M. McGuire, Optimising the removal of geosmin and 2-methylisoborneol by powdered activated carbon, J. - Am. Water Works Assoc., 1988, 80(3), 73–80 Search PubMed.
- D. Cook, G. Newcombe and P. Sztajnbok, The application of powdered activated carbon for MIB and geosmin removal: predicting PAC doses in four raw waters, Water Res., 2001, 35(5), 1325–1333 CrossRef CAS PubMed.
- G. Newcombe and D. Cook, Influences on the removal of tastes and odours by PAC, J. Water Supply: Res. Technol.--AQUA, 2002, 51, 463–474 CAS.
- S.-W. Jung, K.-H. Baek and M.-J. Yu, Treatment of taste and odor material by oxidation and adsorption, Water Sci. Technol., 2004, 49(9), 289–295 CAS.
-
D. R. U. Knappe, R. C. Belk, D. S. Briley, S. R. Gandy, N. Rastogi, A. H. Rike, H. Glasgow, E. Hannon, W. D. Frazier, P. Kohl and S. Pugsley, Algae detection and removal strategies for drinking water treatment plants, American Water Works Association Research Foundation, Denver, CO., 2004 Search PubMed.
- E. Rodriguez, M. E. Majado, J. Meriluoto and J. L. Acero, Oxidation of microcystins by permanganate: reaction kinetics and implications for water treatment, Water Res., 2007, 41, 102–110 CrossRef CAS PubMed.
- C. Kim, S. I. Lee, S. Hwang, M. Cho, H.-S. Kim and S. H. Noh, Removal of geosmin and 2-methylisoboneol by membrane system
combined with powdered activated carbon (PAC) for drinking water treatment, J. Water Process Eng., 2014, 4, 91–98 CrossRef.
- G. Newcombe, M. Drikas and R. Hayes, Influence of characterised natural organic material on activated carbon adsorption: II. Effect on pore volume distribution and adsorption of 2-methylisoborneol, Water Res., 1997, 31(5), 1065–1073 CrossRef CAS.
- G. Newcombe, J. Morrison, C. Hepplewhite and D. Knappe, Simultaneous adsorption of MIB and NOM onto activated carbon: II. Competitive effects, Carbon, 2002, 40(12), 2147–2156 CrossRef CAS.
- J. Ma, G. B. Li, Z. L. Chen, G. R. Xu and G. Q. Cal, Enhanced coagulation of surface waters with high organic content by permanganate peroxidation, Water Sci. Technol.: Water Supply, 2001, 1(1), 51–61 CAS.
- J.-J. Chen and H.-H. Yeh, The mechanisms of potassium permanganate on algae removal, Water Res., 2005, 39, 4420–4428 CrossRef CAS PubMed.
- J. J. Fan, R. Daly, P. Hobson, L. Ho and J. Brookes, Impact of potassium permanganate on cyanobacterial cell integrity and toxin release and degradation, Chemosphere, 2013, 92, 529–534 CrossRef CAS PubMed.
- A. Zamyadi, S. Dorner, M. Ndong, D. Ellis, A. Bolduc, C. Bastien and M. Prévost, Application of in situ measurements for the management of cyanobacteria breakthrough into drinking water treatment plants, Environ. Sci.: Processes Impacts, 2014, 16, 313–323 CAS.
- L. Bowling, D. Ryan, J. Holliday and G. Honeyman, Evaluation of in situ fluorometry to determine cyanobacterial abundance in the Murray and lower Darling rivers, Australia, River Res. & App., 2013, 29(8), 1059–1071 Search PubMed.
- M. Beutler, K. H. Wiltshire, B. Meyer, C. Moldaenke, C. Lüring, M. Meyerhöfer, U.-P. Hansen and H. Dau, A fluorometric method for the differentiation of algal populations in situ and in situ, Photosynth. Res., 2002, 72, 39–53 CrossRef CAS PubMed.
-
M. Babin, Phytoplankton Fluorescence: Theory, Current Literature and in situ Measurement. In Real-Time Coastal Observing System for Marine Ecosystem Dynamics and Harmful Algal Blooms, ed. B. Marcel, S. R. Collin and J. C. John, United Nations Educational, Scientific and Cultural Organization: Paris, France, 2008, pp. 237–280 Search PubMed.
- J. Gregor, B. Maršálek and H. Šípková, Detection and estimation of potentially toxic cyanobacteria in raw water at the drinking water treatment plant by in situ fluorescence method, Water Res., 2007, 41(1), 228–234 CrossRef CAS PubMed.
- A. Zamyadi, N. McQuaid, S. Dorner, F. D. Bird, M. Burch, P. Baker, P. Hobson and M. Prévost, Cyanobacterial detection using in situ fluorescence probes: Managing interferences for improved decision-making, J. - Am. Water Works Assoc., 2012, 104, E466–E479 CrossRef.
- A. Zamyadi, N. McQuaid, M. Prévost and S. Dorner, Monitoring of potentially toxic cyanobacteria using an online multi-probe in drinking water sources, J. Environ. Monit., 2012, 14, 579–588 RSC.
- K. Izydorczyk, C. Carpentier, J. Mrowczynski, A. Wagenvoort, T. Jurczak and M. Tarczynska, Establishment of an alert level framework for cyanobacteria in drinking water resources by using the algae online analyser for monitoring cyanobacterial chlorophyll a, Water Res., 2009, 43, 989–996 CrossRef CAS PubMed.
- N. McQuaid, A. Zamyadi, M. Prévost, D. F. Bird and S. Dorner, Use of in situ phycocyanin fluorescence to monitor potential microcystin-producing cyanobacterial biovolume in a drinking water source, J. Environ. Monit., 2011, 13(2), 455–463 RSC.
- R. K. Henderson, Y. Shutova, A. Baker, A. Zamyadi, P. Le-Clech, A. Branch, G. Newcombe, S. Khan and R. Stuetz, Fluorescence: State-of-the-art monitoring for water treatment systems, Water: J. of the Australian Water Association, 2015, 42(2), 108–113 CAS.
-
YSI Inc., EXO: Advanced water quality monitoring platform (User manual), Yellow Springs Instruments (YSI) Inc., Ohio, USA, 2013 Search PubMed.
-
D. Graham and K. P. Hayes, Application of solid phase micro extraction for the analysis of off-flavours in water, In Proceedings of the WaterTECH Conference, Brisbane, Australia, 1998 Search PubMed.
- S. W. Lloyd, J. M. Lea, P. V. Zimba and C. C. Grimm, Rapid analysis of geosmin and 2methylisoborneol in water using solid phase micro extraction procedures, Water Res., 1998, 32(7), 2140–2146 CrossRef CAS.
-
Cooperative Research Centre for Freshwater Ecology (CRCFE), A Guide to the Identification of Common Blue-Green Algae (Cyanoprokaryotes) in Australian Freshwaters, CRCFE, Australia, 1999 Search PubMed.
-
C. S. Reynolds, The Ecology of Freshwater Phytoplankton, Cambridge University Press, New York, USA, 1984 Search PubMed.
- R. K. L. Yap, M. Whittaker, M. Diao, R. M. Stuetz, B. Jefferson, V. Bulmus, W. L. Peirson, A. V. Nguyen and R. K. Henderson, Hydrophobically-associating cationic polymers as micro-bubble surface modifiers in dissolved air flotation for cyanobacteria cell separation, Water Res., 2014, 61, 253–262 CrossRef CAS PubMed.
- R. Henderson, S. A. Parsons and B. Jefferson, The impact of algal properties and pre-oxidation on solid–liquid separation of algae, Water Res., 2008, 42, 1827–1845 CrossRef CAS PubMed.
- R. K. Henderson, S. A. Parsons and B. Jefferson, The impact of differing cell and algogenic organic matter (AOM) characteristics on the coagulation and flotation of algae, Water Res., 2010, 44(12), 3617–3624 CrossRef CAS PubMed.
- R. K. Henderson, A. Baker, S. A. Parsons and B. Jefferson, Characterisation of algogenic organic matter extracted from cyanobacteria, green algae and diatoms, Water Res., 2008, 42, 3435–3445 CrossRef CAS PubMed.
- J. K. Edzwald, Dissolved air flotation and me, Water Res., 2010, 44(7), 2077–2106 CrossRef CAS PubMed.
- M. Drikas, C. W. K. Chow, J. House and M. D. Burch, Using coagulation, flocculation, and settling to remove toxic cyanobacteria, J. - Am. Water Works Assoc., 2001, 93(2), 100–111 Search PubMed.
- J.-J. Chen, H.-H. Yeh and I.-C. Tseng, Effect of ozone and permanganate on algae coagulation removal – Pilot and bench scale tests, Chemosphere, 2009, 74, 840–846 CrossRef CAS PubMed.
- J. Fan, P. Hobson, L. Ho, R. Daly and J. Brookes, The effects of various control and water treatment processes on the membrane integrity and toxin fate of cyanobacteria, J. Hazard. Mater., 2014, 264, 313–322 CrossRef CAS PubMed.
- M. R. Graham, R. S. Summers, M. R. Simpson and B. W. Macleod, Modeling equilibrium adsorption of 2-methylisoborneol and geosmin in natural waters, Water Res., 2000, 34(8), 2291–2300 CrossRef CAS.
- J. Yu, M. Yang, T.-F. Lin, Z. Guo, Y. Zhang, J. Gu and S. Zhang, Effects of surface characteristics of activated carbon on the adsorption of 2-methylisobornel (MIB) and geosmin from natural water, Sep. Purif. Technol., 2007, 56, 363–370 CrossRef CAS.
- H. G. Peterson, S. E. Hrudey, I. A. Cantin, T. R. Perley and S. L. Kenefick, Physiological toxicity, cell membrane damage and the release of dissolved organic carbon and geosmin by Aphanizomenon flos-aquae after exposure to water treatment chemicals, Water Res., 1995, 29, 1515–1523 CrossRef CAS.
- C. Bastien, R. Cardin, E. Veilleux, C. Deblois, A. Warren and I. Laurion, Performance evaluation of phycocyanin probes for the monitoring of cyanobacteria, J. Environ. Monit., 2011, 13, 110–118 RSC.
- J.-T. Wu and F. Jüttner, Differential partitioning of geosmin and 2-methylisoborneol between cellular constituents in Oscillatoria tenuis, Arch. Microbiol., 1988, 150, 580–583 CrossRef CAS.
- R. Srinivasan and G. A. Sorial, Treatment of taste and odor causing compounds 2-methylisoborneol and geosmin in drinking water: A critical review, J. Environ. Sci., 2011, 23(1), 1–13 CrossRef CAS.
- M. Su, J. Yu, J. Zhang, H. Chen, W. An, R. D. Vogt, T. Anderson, D. Jia, J. Wang and M. Yang, MIB-producing cyanobacteria (Planktothrix sp.) in a drinking water reservoir: Distribution and odor producing potential, Water Res., 2015, 68, 444–453 CrossRef CAS PubMed.
|
This journal is © The Royal Society of Chemistry 2016 |
Click here to see how this site uses Cookies. View our privacy policy here.