Optimization of porous structure of superparamagnetic nanoparticle adsorbents for higher and faster removal of emerging organic contaminants and PAHs†
Received
8th March 2016
, Accepted 31st March 2016
First published on 1st April 2016
Abstract
Superparamagnetic permanently confined micelle array (Mag-PCMAs) nanoparticle adsorbents have been successfully synthesized with a core/shell structure of a silica/surfactant mesostructured hybrid layer on negatively charged maghemite nanoparticles. A micelle swelling agent, 1,3,5-trimethyl benzene, was introduced during the synthesis and removed afterward to optimize the porous structure of Mag-PCMAs to achieve larger surface area and higher pore volume. The isotherms and kinetics of three representative EOCs (methyl orange, sulfamethoxazole and gemfibrozil) and two PAHs (acenaphthene and phenanthrene) onto Mag-PCMAs were determined, and the regeneration and reusability of Mag-PCMAs for methyl orange removal was also investigated. With the optimization of porous structure, the sorption kinetics and capacities of EOCs and PAHs were significantly improved. All of the results showed that Mag-PCMAs can provide fast, effective and sustainable approach for EOCs and PAHs remediation.
Water impact
The porous structure of tailored magnetic nanomaterials was optimized via a micelle swelling agent to achieve larger surface area and higher pore volume. Sorption studies demonstrated significant higher and faster removal of emerging organic compounds and PAHs as well as excellent reusability. These hybrid magnetic core nanoparticle adsorbents can be used successfully for water treatment.
|
1. Introduction
Emerging organic contaminants (EOCs) are a relatively new group of as yet unregulated contaminants due to the limited ecotoxicological information available. EOCs include a wide range of compounds across various chemical classes, such as pharmaceuticals and personal care products (PPCPs), pesticides, surfactants, various industrial additives, and endocrine disruptors (EDCs).1,2 The presence of these EOCs in fresh water or wastewater poses possible toxicological effects to the environment and living organisms.3,4 Current treatment options for the removal of EOCs from aquatic systems typically include advanced oxidation processes5 and membrane technologies,6 since conventional water treatment processes do not provide a high removal efficiency of many EOCs.7–9 However, the major drawback of utilizing these advanced treatment processes is the high level of energy consumption and cost.10
Magnetic core hybrid nanoparticle adsorbents have shown promise for application in water decontamination owing to their large surface area, and high ratio of surface-to-volume.1,8,11–13 With the superparamagnetic feature, these nanoparticle sorbents could be extracted and recycled from wastewater system by applying an external magnetic field.13,14 In previous studies, we synthesized magnetic permanently confined micelle arrays (Mag-PCMAs) with a maghemite core and a silica porous layer that permanently confines cationic surfactant micelles within the mesopores, and had been successfully applied to remove hydrophobic compounds,14 pesticides,15 natural organic matter,16 oxyanions17 and emerging organic contaminants.1 However, since the cationic surfactant is permanently anchored on the silica framework through –Si–O–Si– covalent bonding,14 the surfactant micelles occupy most of the pore space, reducing the adsorption capacity and hindering the kinetics.
Recently, a micelle swelling agent was used to expand the micelles of similar composite materials, to increase the removal of HOCs.18 Thus, one potential strategy to optimize the porous structure of Mag-PCMAs would be to add a micelle swelling agent during the synthesis and later remove it to increase pore volume and surface area. In this study, we introduced 1,3,5-trimethyl benzene (TMB) as a micelle swelling agent to the synthesis of Mag-PCMAs and investigated the effect of different amounts of TMB on the porous structure of Mag-PCMAs, as well as the effect on removal efficiency and sorption kinetics of three EOCs and two PAHs. Relevant physicochemical properties for the contaminants are presented in Table S1.† In addition, since the optimized Mag-PCMAs can be regenerated and reused, they provide a more sustainable approach for decontamination of EOCs and PAHs.
2. Experimental
2.1. Chemicals
Maghemite (iron(III) oxide) nanoparticles (30 nm in diameter) were purchased from Alfa Aesar (USA). Tetramethyl ammonium hydroxide (TMAOH) (25 wt% in water), 3- (trimethoxysily)propyl-octadecyldimethyl-ammonium chloride (TPODAC) (72 wt% in methanol), ammonia (28%), methanol, tetraethyl orthosilicate (TEOS), gemfibrozil, and sulfamethoxazole were purchased from Sigma-Aldrich (USA). Methyl orange, acenaphthene (99% pure), phenanthrene (98% pure), and 1,3,5-trimethyl benzene (TMB) were purchased from Acros Organics (Geel, Belgium). All chemicals were used as received, without further purification. All solutions were prepared with deionized water (18 MΩ cm) from a Barnstead NANOpure Diamond Water Purification System (USA).
2.2. Synthesis of Mag-PCMAs
0.1 g maghemite nanoparticles were dispersed in 40 mL of TMAOH solution (25% by weight) under constant mixing overnight as pre-treatment to activate the surface. Then 2.5 mL of TPODAC and 0.8 mL of TMB were added to maghemite redispersed in water and ethanol in a volumetric ratio of 1
:
6 under constant stirring. 5 mL of 28% ammonium hydroxide were added for base-catalyzed sol–gel hydrolysis along with 1 mL of TEOS to bind the surfactant onto the magnetic iron core. The mixture was stirred overnight at room temperature (22–25 °C), then transferred into a hydrothermal bomb, and treated at 110 °C for 24 h. After being rinsed with ethanol, the particles were dried under vacuum at 60 °C overnight to remove TMB. This method was adapted and modified from the synthesis of Mag-PCMAs reported in a previous study.1 The new material was denominated Mag-PMCA-30, as the weight ratio of TMB to TPODAC is 30%. To investigate the effect of the amount of micelle swelling agent on the sorption capacity and kinetics for EOCs and PAHs adsorption onto Mag-PCMAs, Mag-PCMA-0 and Mag-PMCA-60 were synthesized following the same procedure as Mag-PMCA-30 except that the amount of TMB was changed to 0 mL and 1.6 mL, respectively.
2.3. Characterization of Mag-PCMAs
Transmission electron microscopy (TEM) images were obtained using a JEOL 1230 transmission electron microscope operated at 80 kV. Electron microscopy images were acquired using a Philips Electron Optics environmental scanning electron microscope (ESEM) using an accelerating voltage of 3.00 kV. The surface area and pore volume of Mag-PCMAs were determined using a computer-controlled nitrogen gas adsorption analyzer (TriStar 3000). Before measurements, the samples were degassed at 90 °C in a nitrogen flow for 12 h.20 Thermogravity measurements were used to investigate the amount of surfactant confined on magnetic particle sorbents; thermogravimetric analyses (TGA) were carried out on a TA Instruments Discovery under an air flow of 25 mL min−1 with a heating rate of 10 °C min−1. Magnetization measurements were performed on a Quantum Design MPMS 5XL superconducting quantum interference device (SQUID) Magnetometer.
2.4. Batch sorption of EOCs and PAHs
For most experiments 20 mg of the Mag-PCMAs (Mag-PCMA-0, Mag-PCMA-30, and Mag-PCMA-60) particles were mixed in a 20 ml glass vial with 20.0 ml of a solution with an EOC or PAH with no headspace. Adsorption kinetics studies were carried out at the same conditions as previously stated but for a set amount of time, varying from 5 min to 24 h. The sorption isotherms of EOCs and PAHs onto Mag-PCMAs were determined by the same procedure as the sorption kinetics determination and the equilibration time was 24 h uniformly, to ensure sorption equilibrium is reached. The concentration of adsorbent was varied from 0.05 to 1 g L−1 to study the adsorption isotherms of EOCs and PAHs onto Mag-PMCAs. Additionally, solutions with varying initial concentrations of EOCs and PAHs, which ranged from 0.5 mg L−1 to 100 mg L−1 were treated with 20 mg of Mag-PCMA particles. All vials were placed in an end-over-end shaker on a Dayton-6Z412A Parallel Shaft (USA) roller mixer with a speed of 70 rpm. After this mixing, the Mag-PCMA particles were separated from the mixture with an Eclipse magnet N821 permanent hand-held magnet (50 mm × 50 mm × 12.5 mm; 243.8 g; pull force: 40.1 N). All experiments were conducted at ambient temperature (22–25 °C).
2.5. Regeneration and reuse of Mag-PCMA
To investigate the regeneration and reuse of the three different Mag-PCMAs, 10 mg L−1 methyl orange were used with the same adsorption process, followed by separation of the Mag-PCMAs from solution with the handheld magnet. After the Mag-PCMAs were collected the methyl orange was extracted with methanol. The regenerated Mag-PCMA particles were then reused for subsequent methyl orange sorption experiments. The sorption, extraction, and reuse processes were repeated for five times. Changes in sorption capacity was determined at every cycle.
2.6. Analysis
A Shimadzu high performance liquid chromatograph (HPLC) system (SPD-M10AVP, Shimadzu, MD) equipped with an Ascentis C-18 column (250 × 4.6 mm, 10 μm) and a UV-Vis spectrometer (BioSpec 1601, Shimadzu, MD) were used for EOCs and PAHs analysis.
The equilibrium adsorption of EOCs or PAHs was evaluated according to Langmuir and Freundlich isotherms by eqn (1) and (2), respectively:19
| 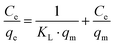 | (1) |
| 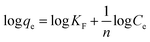 | (2) |
where
Ce is solute concentration (mg L
−1) at equilibrium and
qe is amount adsorbed (mg g
−1),
qm is the maximum sorption capacity (mg g
−1).
KL and
KF are the Langmuir and Freundlich sorption equilibrium constants (L mg
−1), respectively.
Kinetics were analyzed using the pseudo-second-order model in eqn (3),21 since it has been shown to be appropriate for many sorption processes:22–24
| 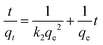 | (3) |
where
k2 (g mg
−1 h
−1) is the rate constant. A first-order kinetics model was also considered, but the fit was inferior.
3. Results and discussion
3.1. Mag-PCMAs synthesis and characterization
The synthesis of Mag-PCMAs is schematically presented in Fig. 1, and the core/shell structure of Mag-PCMAs is demonstrated by the TEM micrograph in Fig. 2. SEM images (Fig. 2A, C and E) show that the roughness of the surface of the Mag-PCMAs changed by adjusting the ratio of micelle swelling agent to surfactant. It indicates that adding TMB during synthesis increases the surface area and pore volume, which is in agreement with the specific BET surface area and the total pore volume calculated based on the nitrogen sorption data (Table 1). In particular, comparing the SEM observation of Mag-PCMA-0 (Fig. 2B) and Mag-PCMA-60 (Fig. 2E), there are large cavities in the silica framework of Mag-PCMA-60, while the surface of Mag-PCMA-0 was smoother with no obvious cells. This can also be seen in the TEM micrographs (Fig. 2B, D and F), where Mag-PCMA-30 (Fig. 2D) and Mag-PCMA-60 (Fig. 2F) exhibit more mesoporous structure and channels inside their particles, which is induced by the micelle swelling agent.
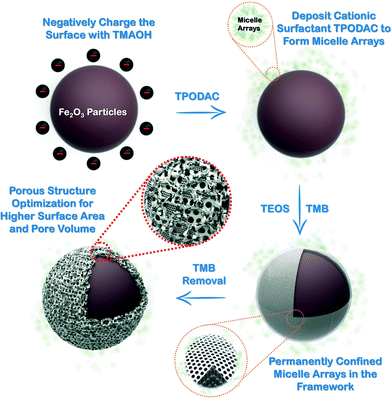 |
| Fig. 1 Schematic representation of Mag-PCMAs synthesis with TMB additive (note: the core and shell are not drawn to scale). | |
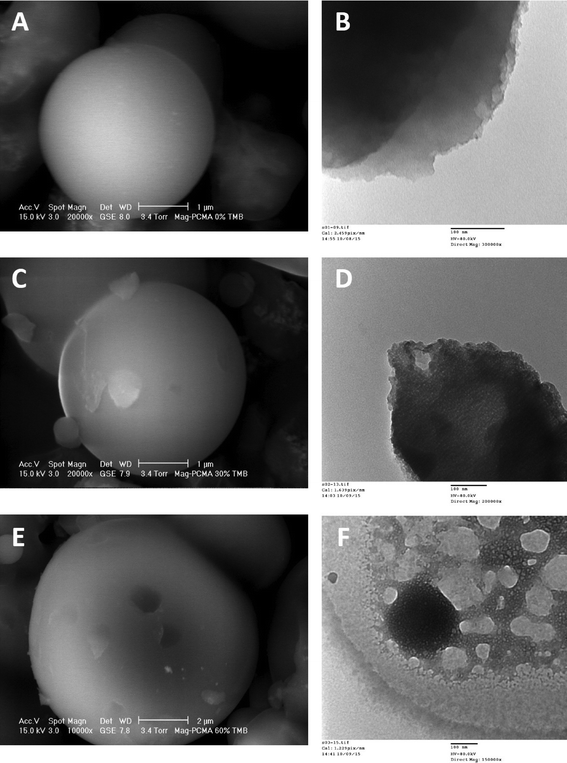 |
| Fig. 2 (A) ESEM micrographs of Mag-PCMA-0 at 20 000×, scale bar = 1 μm; (B) TEM micrographs of Mag-PCMA-0 at 300 000×, scale bar = 100 nm; (C) ESEM micrographs of Mag-PCMA-30 at 20 000×, scale bar = 1 μm; (D) TEM micrographs of Mag-PCMA-30 at 200 000×, scale bar = 100 nm; (E) ESEM micrographs of Mag-PCMA-60 at 10 000×, scale bar = 2 μm; (F) TEM micrographs of Mag-PCMA-60 at 150 000×, scale bar = 100 nm. | |
Table 1 Surface area and pore volume of Mag-PCMA-0, Mag-PCMA-30, and Mag-PCMA-60
|
Surface area (m2 g−1) |
Pore volume (cm3 g−1) |
Mag-PCMA-0 |
1.35 |
0.03 |
Mag-PCMA-30 |
1.61 |
0.04 |
Mag-PCMA-60 |
1.63 |
0.05 |
The TGA curves of the different Mag-PCMAs (Fig. S1†) did not show any significant difference, suggesting that incorporating TMB in the synthesis and post-synthesis removal did not affect the mass percentage of the surfactant confined within the ordered silica framework. Surfactant mass percentage can be determined by the difference of initial and final mass of the sample in TGA curve, and was around 45.5%, 48.6% and 44.6% of the total mass of Mag-PCMA-0, Mag-PCMA-30 and Mag-PCMA-60, respectively. The derivative curve indicates three weight loss steps at about 220, 310, and 600 °C, which can be referred to the decomposition of quaternary ammonium group, the decomposition and carbonization of alkyl chain, and the burn off of carbon, respectively.14
Magnetic characterization with a SQUID magnetometer at 300 K indicated that Mag-PCMA-0, Mag-PCMA-30 and Mag-PCMA-60 have magnetization saturation values of 5.48, 5.24 and 7.09 emu g−1, respectively (Fig. S2†), indicating a relatively high magnetization. Additionally, no remanence was detected in any of the Mag-PCMAs particles, indicating a super-paramagnetism feature due to the nanosized maghemite. Due to the strong magnetization, Mag-PCMAs suspended in water can be quickly separated from the dispersion with a magnet (1000 Oe), indicating that the Mag-PCMAs possesses excellent magnetic responsivity.
3.2. Sorption kinetics of EOCs and PAHs
Time dependent removal of methyl orange (initial concentration = 100 mg L−1) by the three different types of Mag-PCMAs (1 g L−1) showed rapid adsorption of EOCs in the first 30 minutes with above 98% removal efficiency, and thereafter, the rate decreased gradually and reached equilibrium (Fig. 3A). However the removal rate increased significantly with the amount of TMB used during synthesis (Fig. 3A and B). Removal is demonstrated visually in Fig. 3B, where the color of methyl orange contaminated solution faded to clear after 60, 30 and 15 min treatment with Mag-PCMA-0, Mag-PCMA-30, and Mag-PCMA-60, respectively. As all of 3 different Mag-PCMAs showed fast sorption rates of EOCs, the sorption kinetics were conducted with mixing times of 1, 5, 10, 15, 30 and 360 minutes for 3 EOCs and 2 PAHs. The kinetics model was then used to investigate the adsorption rate (Fig. 3C). The results show that k2 (Table 2) decreased with increasing molecular mass (Table S1†) of adsorbant since the process is mostly controlled by diffusion of the organic molecules through the porous structure of the Mag-PCMAs, and diffusivity is a strong inverse function of molecular mass.25 The negative relationship between k2 and molecular mass indicates that the sorption of EOCs and PAHs onto Mag-PCMAs are dominated by the diffusion rate.26 For the sorption of the same EOCs or PAHs onto different Mag-PCMAs, k2 increased as a function of micelle swelling agent used in the synthesis. With larger pore sizes and more porous channels present (Fig. 2), there is a significant increase in diffusion rates and thus sorption kinetics.
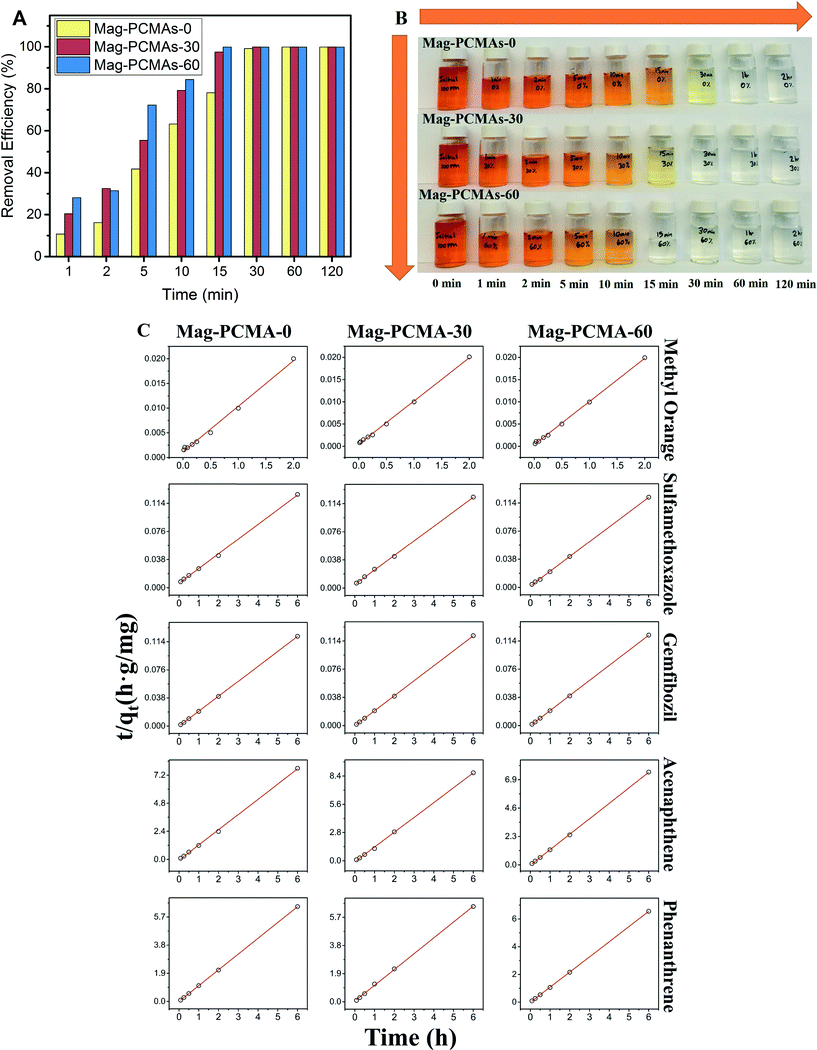 |
| Fig. 3 Methyl orange sorption onto Mag-PCMAs (A) removal efficiency versus time; and (B) visualization of color changes across time sequence; (C) EOCs and PAHs (methyl orange, sulfamethoxazole, gemfibrozil, acenaphthene and phenanthrene) sorption kinetics fitted by pseudo-second order model onto Mag-PCMAs in solution, symbols represent experimental data, and red line represents model prediction. | |
Table 2 Kinetic parameters for EOCs and PAHs sorption by Mag-PCMAs
EOCs/PAHs |
Mag-PCMAs |
q
e (mg g−1) |
k
2 (g h−1 mg) |
R
2
|
Methyl orange |
Mag-PCMA-0 |
109 |
0.077 |
0.995 |
Mag-PCMA-30 |
103 |
0.20 |
0.998 |
Mag-PCMA-60 |
104 |
0.27 |
0.999 |
Sulfamethoxazole |
Mag-PCMA-0 |
50.3 |
0.066 |
0.999 |
Mag-PCMA-30 |
51.0 |
0.082 |
0.999 |
Mag-PCMA-60 |
50.0 |
0.24 |
0.999 |
Gemfibrozil |
Mag-PCMA-0 |
50.0 |
13.3 |
0.999 |
Mag-PCMA-30 |
50.8 |
19.4 |
0.999 |
Mag-PCMA-60 |
50.0 |
16.0 |
0.999 |
Acenaphthene |
Mag-PCMA-0 |
0.84 |
889 |
0.992 |
Mag-PCMA-30 |
0.83 |
1042 |
0.999 |
Mag-PCMA-60 |
0.83 |
1130 |
0.999 |
Phenanthrene |
Mag-PCMA-0 |
0.95 |
362 |
0.999 |
Mag-PCMA-30 |
0.94 |
489 |
0.996 |
Mag-PCMA-60 |
0.95 |
700 |
0.999 |
3.3. Sorption isotherms of EOCs and PAHs
Fig. 4 presents the experimental results of the sorption of EOCs and PAHs along with the fit of the Freundlich isotherm model (fitted parameter values are summarized in Table 3). The Langmuir isotherm model (Fig. S3†) was also applied to analyze the sorption behavior. Based on the correlation coefficients (R2) in Table 3 and Table S2,† the Freundlich model was a better fit, suggesting it's likely to be a multilayer sorption process.27 For the compounds with relatively high log Kow (>3.5), the sorption of different EOCs or PAHs onto specific Mag-PCMA, KF increased with increasing log Kow, suggesting the sorption mechanism was dominated by hydrophobic interactions between surfactant micelles and organic contaminants,15,28 while for compounds with relatively low log Kow (<1), the relationship between KF and log Kow was less obvious, and another potential mechanism could be electrostatic interactions as the confined surfactant micelles are cationic.1,17 For the sorption of the same EOCs or PAHs onto different Mag-PCMAs, higher sorption capacities were achieved with increasing percentage of TMB used in the synthesis (Table 3). It suggests that with larger pore size and higher pore volume (Table 1) sorption capacity increases. The large amount of surfactant micelles confined in the silica framework (Fig. S1†) also contributed.
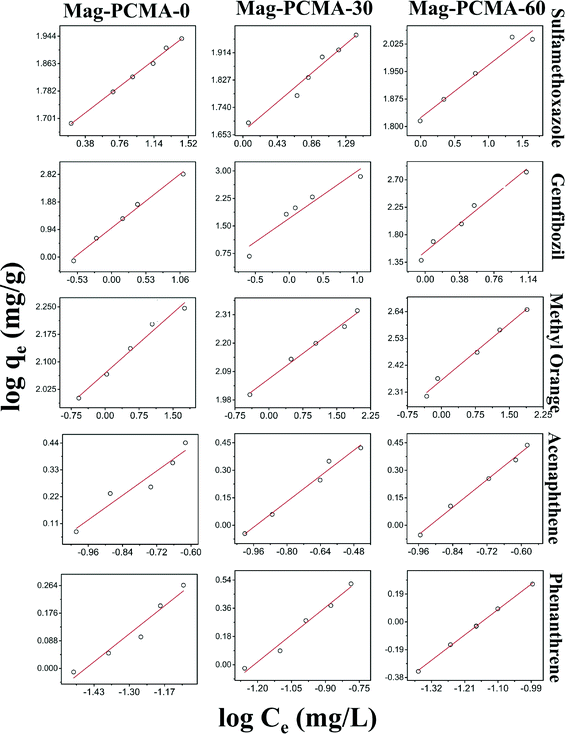 |
| Fig. 4 Adsorption of EOCs and PAHs (sulfamethoxazole, gemfibrozil, methyl orange, acenaphthene and phenanthrene) onto Mag-PCMA in solution with Freundlich adsorption isotherms fit, symbols represent experimental data, and red line represents model prediction. | |
Table 3 Freundlich isotherm parameters for EOCs and PAHs sorption on Mag-PCMAs
EOCs and PAHs |
Mag-PCMAs |
Freundlich |
K
F ((mg g−1)/(L mg−1)−n) |
1/n |
R
2
|
Sulfamethoxazole |
Mag-PCMA-0 |
43.6 |
0.21 |
0.999 |
Mag-PCMA-30 |
46.1 |
0.22 |
0.959 |
Mag-PCMA-60 |
66.6 |
0.14 |
0.981 |
Gemfibrozil |
Mag-PCMA-0 |
9.88 |
1.7 |
0.993 |
Mag-PCMA-30 |
50.9 |
1.3 |
0.960 |
Mag-PCMA-60 |
33.2 |
1.2 |
0.990 |
Methyl orange |
Mag-PCMA-0 |
117 |
0.11 |
0.982 |
Mag-PCMA-30 |
115 |
0.13 |
0.992 |
Mag-PCMA-60 |
229 |
0.16 |
0.991 |
Acenaphthene |
Mag-PCMA-0 |
8.47 |
0.84 |
0.926 |
Mag-PCMA-30 |
9.94 |
1.2 |
0.982 |
Mag-PCMA-60 |
14.1 |
1.3 |
0.995 |
Phenanthrene |
Mag-PCMA-0 |
10.1 |
0.69 |
0.952 |
Mag-PCMA-30 |
25.6 |
1.2 |
0.981 |
Mag-PCMA-60 |
65.3 |
1.6 |
0.998 |
3.4. Regeneration and reuse of Mag-PCMAs
To demonstrate the regenerability and reusability of the modified Mag-PCMAs, the recovery of methyl orange sorbed onto the Mag-PCMA was investigated using methanol extraction. The removal of methyl orange from three different Mag-PCMAs during five continuous cycles of regeneration and reuse are shown in Fig. 5. No significant losses of sorption capacity of methyl orange was observed for the regenerated Mag-PCMA after 5 cycles, indicating good reusability of Mag-PCMAs.
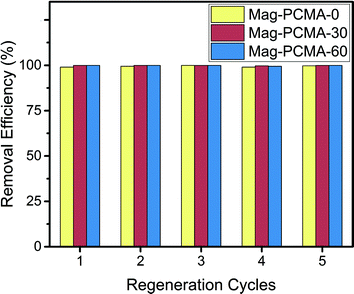 |
| Fig. 5 Sorption of acenaphthene onto Mag-PCMA during five regeneration cycles. | |
4. Conclusions
The porous structure of the synthesized magnetic nanoparticle sorbents were optimized by introducing a micelle swelling agent and removing it after synthesis. The resulting sorbents have a core/shell structure with a maghemite core and a silica layer with high porosity that permanently confines the surfactant micelles within the framework. The surfactant, TPODAC, has a reactive –Si(OCH3)3 group on its hydrophilic end, which allows the surfactant micelles to permanently anchor on the silica framework through covalent bonding. This strong binding of the surfactant enables the Mag-PCMAs to be regenerable at a lower operating cost. The optimized Mag-PCMAs showed significantly higher sorption kinetic rate as well as slightly higher sorption capacity to EOCs and PAHs. The Mag-PCMAs are promising sorbents for fast, effective and sustainable remediation of EOCs and PAHs. Based on the fast sorption kinetics and high sorption capacity for EOCs and PAHs, with high regeneration performance of Mag-PCMAs, larger scale continuous batch reactors can be designed for water treatment.
Acknowledgements
This work made use of MRL Central Facilities supported by the MRSEC Program of the National Science Foundation under awards NO. DMR 1121053; a member of the NSF-funded Materials Research Facilities Network (http://www.mrfn.org). We thank the MRL Central Facilities for the use of their TGA, SEM, BET Porosimeter, and SQUID instruments as well as Amanda Strom and Paige Roberts at the UCSB MRL for their help with these instruments.
References
- Y. Huang and A. A. Keller, ACS Sustainable Chem. Eng., 2013, 1, 731–736 CrossRef CAS.
- K. E. Murray, S. M. Thomas and A. A. Bodour, Environ. Pollut., 2010, 158, 3462–3471 CrossRef CAS PubMed.
- V. S. Thomaidi, A. S. Stasinakis, V. L. Borova and N. S. Thomaidis, J. Hazard. Mater., 2015, 283, 740–747 CrossRef CAS PubMed.
- Z. H. Yan, X. F. Yang, G. H. Lu, J. C. Liu, Z. X. Xie and D. H. Wu, Sci. Total Environ., 2014, 470, 171–179 CrossRef PubMed.
- M. Ibanez, E. Gracia-Lor, L. Bijlsma, E. Morales, L. Pastor and F. Hernandez, J. Hazard. Mater., 2013, 260, 389–398 CrossRef CAS PubMed.
- L. N. Nguyen, F. I. Hai, J. G. Kang, W. E. Price and L. D. Nghiem, Int. Biodeterior. Biodegrad., 2013, 85, 474–482 CrossRef CAS.
- P. E. Stackelberg, E. T. Furlong, M. T. Meyer, S. D. Zaugg, A. K. Henderson and D. B. Reissman, Sci. Total Environ., 2004, 329, 99–113 CrossRef CAS PubMed.
- A. S. Adeleye, J. R. Conway, K. Garner, Y. Huang, Y. Su and A. A. Keller, Chem. Eng. J., 2016, 286, 640–662 CrossRef CAS.
- V. Matamoros, R. Gutierrez, I. Ferrer, J. Garcia and J. M. Bayona, J. Hazard. Mater., 2015, 288, 34–42 CrossRef CAS PubMed.
- A. Garcia-Rodriguez, V. Matamoros, C. Fontas and V. Salvado, Environ. Sci. Pollut. Res., 2014, 21, 11708–11728 CrossRef PubMed.
- Y. Su, A. S. Adeleye, A. A. Keller, Y. Huang, C. Dai, X. Zhou and Y. Zhang, Water Res., 2015, 74, 47–57 CrossRef CAS PubMed.
- Y. Su, A. S. Adeleye, Y. Huang, X. Sun, C. Dai, X. Zhou, Y. Zhang and A. A. Keller, Water Res., 2014, 63, 102–111 CrossRef CAS PubMed.
- Y. Huang and A. A. Keller, Water Res., 2015, 80, 159–168 CrossRef CAS PubMed.
- P. Wang, Q. H. Shi, Y. F. Shi, K. K. Clark, G. D. Stucky and A. A. Keller, J. Am. Chem. Soc., 2009, 131, 182–188 CrossRef CAS PubMed.
- K. K. Clark and A. A. Keller, Water, Air, Soil Pollut., 2012, 223, 3647–3655 CrossRef CAS.
- H. Wang, A. A. Keller and K. K. Clark, J. Hazard. Mater., 2011, 194, 156–161 CrossRef CAS PubMed.
- K. K. Clark and A. A. Keller, Water Res., 2012, 46, 635–644 CrossRef CAS PubMed.
- Y. F. Shi, B. Li, P. Wang, R. Dua and D. Y. Zhao, Microporous Mesoporous Mater., 2012, 155, 252–257 CrossRef CAS.
-
F. M. M. Morel and J. G. Hering, Principles and Applications of Aquatic Chemistry, Wiley, 1993 Search PubMed.
- Y. Huang, J.-K. Yang and A. A. Keller, ACS Sustainable Chem. Eng., 2014, 2, 1128–1138 CrossRef CAS.
- N. T. Coleman, A. C. Mcclung and D. P. Moore, Science, 1956, 123, 330–331 CAS.
- Y.-S. Ho and G. McKay, Process Biochem., 1999, 34, 451–465 CrossRef CAS.
- Y.-S. Ho, J. Hazard. Mater., 2006, 136, 681–689 CrossRef CAS PubMed.
- F.-C. Wu, R.-L. Tseng, S.-C. Huang and R.-S. Juang, Chem. Eng. J., 2009, 151, 1–9 CrossRef CAS.
- W. J. Weber and J. C. Morris, J. Sanit. Eng. Div., Am. Soc. Civ. Eng., 1963, 89, 31–60 Search PubMed.
- I. T. Yeom, M. M. Ghosh and C. D. Cox, Environ. Sci. Technol., 1996, 30, 1589–1595 CrossRef CAS.
- W. J. Weber, P. M. Mcginley and L. E. Katz, Water Res., 1991, 25, 499–528 CrossRef CAS.
-
R. P. Schwarzenbach, P. M. Gschwend and D. M. Imboden, Environmental organic chemistry, John Wiley & Sons, 2005 Search PubMed.
Footnote |
† Electronic supplementary information (ESI) available. See DOI: 10.1039/c6ew00066e |
|
This journal is © The Royal Society of Chemistry 2016 |
Click here to see how this site uses Cookies. View our privacy policy here.