DOI:
10.1039/C6QI00137H
(Research Article)
Inorg. Chem. Front., 2016,
3, 1076-1081
Two-step warming solvothermal syntheses, luminescence and slow magnetic relaxation of isostructural dense LnMOFs based on nanoscale 3-connected linkers†
Received
17th May 2016
, Accepted 16th June 2016
First published on 20th June 2016
Abstract
Two isostructural dense lanthanide metal–organic frameworks derived from an extended ligand 1,3,5-tris(4-carboxyphenyl)benzene (H3BTB), [Ln(BTB)H2O]n (Ln = Eu, 1; Ln = Dy, 2), which are constructed via a cross-like folding assembly strategy, were yielded by ‘two-step warming solvothermal synthesis’. Complex 1 shows luminescence while complex 2 exhibits field-induced two-step magnetic relaxation.
Introduction
The design and construction of metal–organic frameworks (MOFs) with extended ligands as linkers open a new avenue to molecular microporous materials different from zeolites. The increasing interest in such molecular materials originates from their structural diversity and their potential applications in many fields such as gas storage, separation and catalysis.1 Although the framework structures with pores can be rationally designed through the judicious choice of metal nodes and extended organic linkers, unexpected densely packed MOFs have been occasionally obtained owing to the mutual interpenetration role.2 Nevertheless, the direct assembly of densely packed MOFs with expanded linkers using the folding principle is very difficult to realize because it needs to overcome the large tension.
Recently, the MOFs have also found many other potential applications not dependent on pores. In particular, lanthanide metal–organic frameworks (LnMOFs) often show intriguing luminescence and/or magnetic properties owing to unique 4f-electrons.3 On the one hand, LnMOFs may display photoluminescence properties because of not only the narrow luminescence emissions of lanthanide ions but also the ‘antenna effect’ of spacer ligands.4 On the other hand, some LnMOFs, particularly DyMOF, may exhibit single-molecule magnet (SMM) behaviours due to large magnetic moments and the remarkable magnetic anisotropy of lanthanide ions.5 However, in comparison with numerous luminescent LnMOFs, LnMOFs displaying SMM behaviours have scarcely been obtained because the coordination geometry and environment of the lanthanide ions are hard to control in the LnMOF system.6 Consequently, only quite limited isostructural LnMOFs, which exhibit either luminescence or slow magnetic relaxation through the change of lanthanide ions, have been well characterized.6p
One striking advantage of the densely packed MOFs is their thermal stability, this is important for many physical properties such as optical and magnetic properties. In this article, we demonstrate that the good control of solvothermal reaction conditions and the deliberate choice of extended ligands may provide a new opportunity to obtain highly-dense LnMOFs without interpenetration. To explore such unique molecular materials, we chose 1,3,5-tris(4-carboxyphenyl)benzene (H3BTB) as the expanded ligand, and two 3D dense LnMOFs, [Ln(BTB)H2O]n (Ln = Eu, 1; Ln = Dy, 2), were prepared via a special solvothermal reaction process, which are isostructural, and exhibit interesting luminescence and magnetic properties, depending on the lanthanide(III) ions used.
Experimental
Instrumentation and methods
The elemental analyses were determined on a Vario ELIII elemental analyser. The infrared spectra were recorded on a Bruker/Tensor-27 spectrophotometer with pressed KBr pellets in the range 4000–400 cm−1. The fluorescence and phosphorescence spectra in the solid state were recorded on a HITACHI F-4500 luminescence spectrophotometer at room temperature. The solid state fluorescence lifetime was measured on an Edinburgh Analytical Instruments F900. The solid state phosphorescence lifetime and the absolute emission quantum yield were determined using an Edinburgh Analytical Instruments F980. The X-ray powder diffraction (XRD) spectra were recorded on a PANalytical/Empyrean-1 (for room temperature) or Empyrean-2 (for high temperature) diffractometer with Cu-Kα (λ = 1.5418 Å) radiation. The magnetic susceptibility measurements were carried out on a Quantum Design MPMS-XL5 SQUID magnetometer. Diamagnetic corrections were estimated from Pascal's constants of all components.
Syntheses of 1 and 2
A mixture of Ln(NO3)3·5H2O (0.2 mmol), H3BTB (0.2 mmol), H2O (2.0 mL) and DMF (3.0 mL) was sealed in a Teflon-lined stainless steel autoclave (25.0 mL) and heated at 130 °C for 2 days, then increased to 170 °C and maintained at this temperature for 3 days. After the autoclave had cooled automatically to room temperature over 10 h, X-ray qualified colourless polyhedron crystals of 1 (Ln = Eu, 72% yield based on Eu), or colourless polyhedron crystals of 2 (Ln = Dy, 68% yield based on Dy) were harvested. These crystals were washed with water and dried in air. Elemental analysis calcd (%) for C27H17EuO7 (1): C, 53.57; H, 2.83; found: C, 53.53; H, 2.85. IR (KBr pellet, cm−1) of 1: 3427 (s, br), 3169 (w), 3067 (w), 2928 (w), 2846 (w), 1658 (w), 1609 (m), 1582 (m), 1531 (s), 1403 (s), 1247 (w), 1188 (w), 1108 (w), 1012 (w), 854 (w), 806 (w), 781 (m), 701 (w), 662 (w), 552 (w), 486 (w). Elemental analysis calcd (%) for C27H17DyO7 (2): C, 52.65; H, 2.78; found: C, 52.60; H, 2.81. IR (KBr pellet, cm−1) of 2: 3432 (s, br), 3167 (w), 3066 (w), 2927 (w), 2845 (w), 1652 (w), 1610 (w), 1582 (m), 1531 (s), 1404 (s), 1247 (w), 1187 (w), 1108 (w), 1012 (w), 853 (w), 807 (w), 780 (m), 701 (w), 662 (w), 553 (w), 486 (w).
Crystallography
A single crystal with dimensions 0.33 × 0.10 × 0.10 mm3 of 1 or 0.12 × 0.10 × 0.09 mm3 of 2 was selected to collect data on a Rigaku MM007HF Saturn724+ diffractometer with Mo-Kα radiation (λ = 0.71073 Å) in the φ-scan mode. Cell parameters were obtained by the global refinement of the positions of all collected reflections. The structure was solved by direct methods and refined with the ShelXL refinement package using least squares minimisation. All non-hydrogen atoms were refined anisotropically, and all hydrogen atoms but those in the solvent hydrate molecule were set in calculated positions and refined as riding atoms. Crystallographic data and structure determination parameters of the two compounds are given in Table 1.
Table 1 Crystal data and structural refinement parameters for complexes 1 and 2
|
1
|
2
|
R
1 = ∑||Fo| − |Fc||/∑|Fo|.
wR2 = ∑{[w(Fo2 − Fc2)2]/∑[wFo2]2}1/2.
|
Chemical formula |
C27H17EuO7 |
C27H17DyO7 |
Formula weight |
605.37 |
615.91 |
Crystal system |
Orthorhombic |
Orthorhombic |
Space group |
F
ddd
|
F
ddd
|
a/Å |
22.503(5) |
22.497(5) |
b/Å |
28.973(6) |
28.847(6) |
c/Å |
29.063(6) |
28.988(6) |
V/Å3 |
18 948(7) |
18 813(7) |
Z
|
32 |
32 |
T/K |
173(2) |
173(2) |
λ(Mo-Kα)/Å |
0.71073 |
0.71073 |
ρ
calc/g cm−3 |
1.698 |
1.740 |
μ(Mo-Kα)/mm−1 |
2.693 |
3.223 |
F(000) |
9536 |
9632 |
θ range |
1.34 °≤ θ ≤ 27.46° |
3.30° ≤ θ ≤ 27.45° |
Limiting indices |
−29 ≤ h ≤ 29, −37 ≤ k ≤ 37, −37 ≤ l ≤ 37 |
−29 ≤ h ≤ 29, −37 ≤ k ≤ 37, −37 ≤ l ≤ 37 |
Reflections collected |
51 201 |
51 832 |
Unique reflections |
5443 |
5386 |
R
1 a [I > 2σ(I)] |
0.0851 |
0.0919 |
wR2 b [I > 2σ(I)] |
0.1812 |
0.2165 |
R
1 a [all data] |
0.0867 |
0.0938 |
wR2 b [all data] |
0.1822 |
0.2168 |
S
|
1.189 |
1.123 |
Results and discussion
Preparation and characterisation
It is noteworthy that the 3-connected linker BTB3− has been used to solvothermally synthesize several LnMOFs recently,7 most of them are microporous molecular materials that can be used for gas storage, separation and luminescence. To avoid yielding similar porous LnMOFs, we developed a two-step solvothermal synthesis method. Both 3D dense LnMOFs were produced in good yields using the hydrothermal reaction of Eu(NO3)3 or Dy(NO3)3, H3BTB (molar ratio 1
:
1) in 5 mL of H2O–DMF (v/v 2
:
3) mixed solvents at 130 °C for 2 days, then 170 °C for 3 days. Surprisingly, no crystalline products could be harvested if either 130 °C or 170 °C was solely utilized as the solvothermal reaction temperature, and the reversal reaction processes at 170 °C for 3 days then 130 °C for 2 days would be fruitless too. Therefore, the ‘first low temperature then high temperature’ two-step solvothermal process is obligatory for these two 3D dense LnMOFs. We call this specific solvothermal method as the ‘two-step warming solvothermal synthesis’, which is different from the occasional solvothermal synthesis of first high temperature then low temperature, in which the low temperature is just used for the better crystallization of products. Interestingly, Hou and Zang et al. also prepared [Eu(BTB)H2O]n and its isostructural compound [Tb(BTB)H2O]n quite recently,8 but they had to use 1-alkyl-3-methylimidazolium bromide ionic liquids to direct the syntheses.
X-ray thermodiffraction analysis of 1 was carried out in air (Fig. S1, ESI†). From 25 to 350 °C, the XRD diffraction peaks match well with the simulated patterns derived from the single-crystal structure, indicating the phase purity and the thermal stability of 1. At about 400 °C, the network of 1 begins to collapse. The thermogravimetric analysis (TGA) also shows that compound 1 starts to decompose at about 400 °C (Fig. S2, ESI†), confirming the thermal stability of 1. The phase purity and the thermal stability of 2 are also examined by its room temperature XRD spectrum (Fig. S3, ESI†) and its TGA spectrum (Fig. S4, ESI†), which exhibits similar results to 1.
Crystal structures
Single crystal X-ray crystallographic study revealed that 1 and 2 are isomorphic, crystallizing in the orthorhombic space group Fddd. So the structure of dysprosium(III) species is described here as representative. As shown in Fig. 1, the asymmetric unit of complex 2 contains a Dy3+ cation, a BTB3− anion and a coordination water molecule. The Dy3+ ion coordinates with seven oxygen atoms from six carboxylate groups of BTB3− ligands and one oxygen atom from the terminal water (Fig. 1a), the formed coordination geometry was analysed by using Shape software9 to be a Johnson elongated triangular bipyramid, with a small deviation of 1.529 from the ideal D3h symmetry (Table S1, ESI†). The Dy–O bond distances (average 2.378 Å) are in the normal range. Each BTB3− ligand bridges three couples of Dy3+ ions through three carboxylate groups (Fig. 1a), which fall into two categories, adopting the syn–syn bridging mode and the η-O,O′–μ-O,O bridging mode,7b respectively.
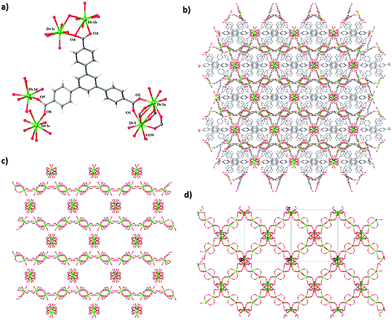 |
| Fig. 1 (a) Coordination environments of the BTB3− ligand and the Dy3+ ion in 2; (b) packing diagram of the 3D framework of 2 viewed down the [110] crystallographic direction; (c) the SBUs viewed down the [110] crystallographic direction; (d) the SBUs viewed down the [011] crystallographic direction. | |
Each Dy3+ ion links its symmetry equivalents through carboxylate bridges, forming a 1D chain as a secondary building unit (SBU) (Fig. 1b). The most interesting feature of 2 is that the SBUs adopt a cross-like arrangement, along the long diagonal line and the short diagonal line of the ac plane, respectively (Fig. 1c and d). This three-dimensional folding array is further enhanced by the bridging role of the BTB3− ligand, each BTB3− ligand links to four Dy3+ ions from two parallel SBUs and two Dy3+ ions from the cross SBU. Consequently, a unique 3D dense framework is generated, of which the potential void volume of open channels is only 7.7%, calculated by using the PLATON program, but no solvent guest molecules could be observed. In comparison, the SBUs in the other electroneutral LnMOFs based on the BTB3− ligand run along only one direction rather than the cross-like mode, forming porous frameworks with a very high potential void volume (>38.5%) (Table S2, ESI†).7a–e
Compound 1 is of the same structure as 2. The average Eu–O bond length (average 2.412 Å) in 1 is slightly larger than the mean Dy–O bond length (average 2.378 Å) in 2, obviously owing to the lanthanide contraction effect.
Luminescence properties of 1
The solid state luminescence of 1 was investigated at room temperature, which includes fluorescence and phosphorescence emissions. For the fluorescence properties, as shown in Fig. 2a, the excitation spectrum (λem = 612 nm) shows absorption bands at 396, 417, 467, 527 and 535 nm, characteristic peaks for the Eu3+ ion. Its emission spectrum (λex = 395 nm) also display characteristic transitions of the Eu3+ ion. The most intense emitting band at 612 nm, which dominates the red light emission, is the 5D0 → 7F2 transition. The other peaks at 591 and 698 nm are assigned to the 5D0 → 7F1 and 5D0 → 7F4 transitions, respectively. The intensity of the 5D0 → 7F2 electric dipole transition is obviously stronger than that of the 5D0 → 7F1 magnetic dipole transition mainly due to the asymmetry of the coordination environment of the Eu3+ ion.6m
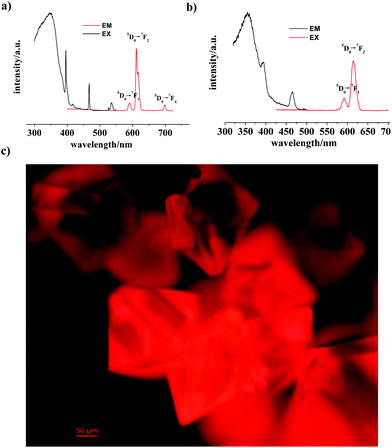 |
| Fig. 2 (a) Fluorescence (λem = 612 nm, λex = 395 nm) and (b) phosphorescence (λem = 615 nm, λex = 395 nm) spectra in the solid state at room temperature for 1; (c) photoluminescence microscopy image of 1 excited with the blue band (450–490 nm) of a mercury lamp. | |
The fluorescence decay curve of 1 was determined by monitoring the maximal emission with excitation at 395 nm at room temperature (Fig. S5, ESI†). This curve could be fitted by a double exponential function, I = A + B1
exp(−t/τ1) + B2
exp(−t/τ2) (τ1 and τ2 are the fast and slow components of the luminescence lifetimes, while A, B1, and B2 are the fitting parameters). The average lifetime (τ) was then calculated based on the formula τ = (B1τ12 + B2τ22)/(B1τ1 + B2τ2),10 giving a value of 0.93 ns. The phosphorescence emissions are obviously weaker than those for the fluorescence (Fig. 2b). Only two peaks at 395 and 466 nm were observed in the excitation spectrum (λem = 615 nm), and only two peaks at 593 and 615 nm, which correspond to the 5D0 → 7F1 and 5D0 → 7F4 transitions too, were observed in the emission spectrum (λex = 395 nm). The phosphorescence lifetime was also fitted by the double exponential function and then calculated to be 430.56 μs (under excitation at 395 nm, Fig. S6, ESI†). The total absolute emission quantum yield, which was measured at room temperature and at 395 nm maximizing the emissions, is 15.28%, being comparable with that of [Eu(BTB)(DMSO)2]·H2O (19.89%).7d Furthermore, under blue light excitation from a mercury lamp, complex 1 exhibits strong red photoluminescence (Fig. 2c).
Magnetic properties of 2
Direct current (dc) magnetic susceptibilities of the microcrystalline powdered sample 2 were determined in the temperature range of 2–300 K in the 1 kOe field. The χT value of 14.14 cm3 kmol−1 at room temperature is in good agreement with the value expected (14.17 cm3 kmol−1) for one spin-only Dy3+ ion (S = 5/2, L = 5, 6H15/2, g = 4/3). The χT product decreases slowly when the temperature drops from 300 K to 100 K, then falls quickly upon further cooling, reaching a value of 8.86 cm3 kmol−1 at 2 K finally (Fig. S7, ESI†). Such χT value diminishment is mainly owing to the ligand field-induced depopulation of the Dy3+ Stark sublevels,5 and the possible weak antiferromagnetic interaction between the Dy3+ ions is another reason.
The field dependence of the magnetization at different temperatures was investigated. The M versus H/T plots are reduced, which are obviously non-superimposed (Fig. S8, ESI†), suggesting the strong magnetic anisotropy. Therefore, the alternating-current (ac) magnetic susceptibilities of 2 were measured to study possible SMM behaviours. However, no peaks appear in the χ′′ versus T plots of 2 at above 2 K (Fig. S9, ESI†). A dc field was then employed to examine the possible quantum-tunnelling effects. As shown in Fig. 3a, under a 1 kOe dc field, the χ′′ peaks could be observed clearly at a high frequency range (the corresponding plots of χ′ vs. T are presented in Fig. S10, ESI†). Such field-improved χ′′ signals indicate the existence of quantum-tunnelling effects, which can be fully or partly suppressed by this dc field through removing the ground-state degeneracy of the Dy3+ ion.11 The Arrhenius law, τ = τ0
exp(Ueff/kT) (here τ = 1/2πf), was adopted to calculate the effective thermal barrier. The ln(τ)–1/T plot based on the peak temperatures corresponding to the ac oscillating frequencies, was fitted to the formula ln
τ = ln
τ0 + Ueff/kT, giving Ueff/k = 17.8 K and τ0 = 1.4 × 10−7 s (Fig. 3b). The τ0 value is in good agreement with those for the SMMs (from 10−6 to 10−10 s); the Ueff/k value of 2 is comparable with those of the reported high-dimensional Dy-MOFs6o but not remarkable, the reason is that the slow magnetic relaxation of 2 is mainly ascribed to the single-ion magnetic behaviour and the symmetry of the ligand field for the Johnson elongated triangular bipyramid Dy(III) ion in 2 (D3h) is not very high.
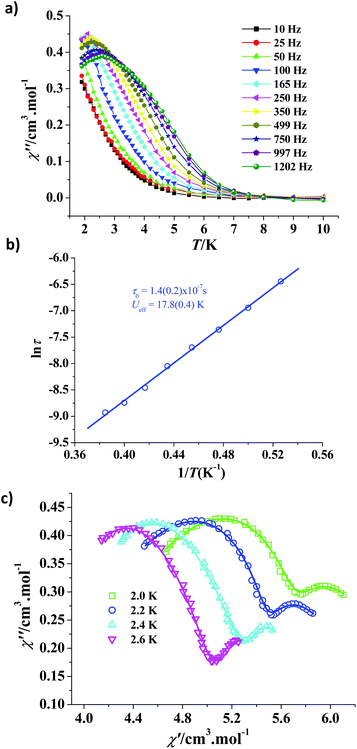 |
| Fig. 3 (a) Plots of χ′′ vs. T measured in a 2.5 Oe oscillating field under a 1 kOe dc field for 2; (b) plot of ln(τ) vs. 1/T for 2, the solid line represents the best fitting with the Arrhenius law; (c) Cole–Cole plots for 2 with fitted lines at fixed temperatures (Hdc = 1 kOe and Hac = 2.5 Oe). | |
In order to estimate the magnetic anisotropy and magnetic axis in 2, the electrostatic model installed in the Magellan program12 was adopted. The calculated results indicate that the magnetic axis directionality of the dysprosium(III) ion is close to the direction defined by the Dy1 atom and the O6#1 atom (symmetry code: #1: 1.25 − x, y, 1.25 − z) with a separation angle of 10.69°, and close to the direction defined by the Dy1 atom and the O1#2 atom (symmetry code: #2: 2 − x, 1 − y, 1 − z) with a separation angle of 12.84° (Fig. S11, ESI†). The shift of χ′′ peak temperatures (Tf) from different frequencies (f) was judged by a parameter, Φ = (ΔTf/Tf)/Δ(log
f), providing the Φ value of 0.47, which is consistent with the normal value for a superparamagnet (Φ > 0.1) but quite larger than that for a spin glass state (Φ ≈ 0.01).13 The result confirms the SMM behaviours of 2.
Interestingly, the frequency-dependent ac susceptibilities determined under the 1 kOe dc field at 2.0–2.6 K revealed that there exist field-induced two-step relaxation processes in 2. As shown in Fig. 3c, the left and the right parts of the Cole–Cole plots correspond to the fast relaxation (FR) phase and the slow relaxation (SR) phase, respectively, which could be fitted by the sum of two modified Debye functions (Fig. S12–15, ESI†).14 The α1 values of 1.86 × 10−7–0.037 are remarkably smaller than the α2 values of 0.39–0.66, suggesting that the fast relaxation phase has a narrow distribution of the relaxation time while the distribution of relaxation time for the slow relaxation phase is not narrow (Table S3, ESI†). Notably, the two-step thermal magnetic relaxation originates from the field-inducing role15 due to only one type of dysprosium(III) ion existing in 2. Such field-induced two-step magnetic relaxation is seldom observed in high-dimensional DyMOFs.6m,o,q In addition, complex 2 does not show any magnetic hysteresis at 1.9 K (Fig. S16, ESI†).
Conclusions
In summary, two 3D dense LnMOFs with expanded ligands have been solvothermally prepared via a folding assembly strategy rather than the mutual interpenetration method. Complex 1 displays the typical luminescence properties of the europium(III) ion, including both fluorescence and phosphorescence emissions; while the dysprosium(III) analogue behaves as a SMM, showing two-step thermal magnetic relaxation under a 1 kOe dc field. Complexes 1 and 2 represent rare examples of isostructural LnMOFs exhibiting either luminescence or single-molecule magnet behaviour through a change of lanthanide ions. This work demonstrates that the two-step warming synthesis provides an effective and facile approach toward novel LnMOFs different from those obtained by normal one-step solvothermal syntheses, and the folding assembly strategy opens a new perspective for the assembly of dense LnMOFs with interesting luminescence and magnetic properties.
Acknowledgements
We gratefully acknowledge financial support from the National Key Basic Research Program of China (2013CB933403), the National Natural Science Foundation of China (21471154 and 91022014), and the Strategic Priority Research Program of the Chinese Academy of Sciences (XDB12010103).
Notes and references
-
(a) M. O'Keeffe and O. M. Yaghi, Chem. Rev., 2012, 112, 675 CrossRef PubMed;
(b) R. B. Getman, Y.-S. Bae, C. E. Wilmer and R. Q. Snurr, Chem. Rev., 2012, 112, 703 CrossRef CAS PubMed;
(c) M. P. Suh, H. J. Park, T. K. Prasad and D.-W. Lim, Chem. Rev., 2012, 112, 782 CrossRef CAS PubMed;
(d) J.-R. Li, J. Sculley and H.-C. Zhou, Chem. Rev., 2012, 112, 869 CrossRef CAS PubMed;
(e) K. Sumida, D. L. Rogow, J. A. Mason, T. M. McDonald, E. D. Bloch, Z. R. Herm, T.-H. Bae and J. R. Long, Chem. Rev., 2012, 112, 724 CrossRef CAS PubMed;
(f) M. Yoon, R. Srirambalaji and K. Kim, Chem. Rev., 2012, 112, 1196 CrossRef CAS PubMed;
(g) S. Ma, X.-S. Wang, D. Yuan and H.-C. Zhou, Angew. Chem., Int. Ed., 2008, 47, 4130 CrossRef CAS PubMed;
(h) A. H. Assen, Y. Belmabkhout, K. Adil, P. M. Bhatt, D.-X. Xue, H. Jiang and M. Eddaoudi, Angew. Chem., Int. Ed., 2015, 54, 14353 CrossRef CAS PubMed;
(i) S. Ou and C.-D. Wu, Inorg. Chem. Front., 2014, 1, 721 RSC;
(j) Y. Zhang, X. Feng, S. Yuan, J. Zhou and B. Wang, Inorg. Chem. Front., 2016 10.1039/C6QI00042H.
- H.-L. Jiang, T. A. Makal and H.-C. Zhou, Coord. Chem. Rev., 2013, 257, 2232 CrossRef CAS.
- J. Rocha, L. D. Carlos, F. A. A. Paza and D. Ananias, Chem. Soc. Rev., 2011, 40, 926 RSC.
-
(a) Y. Cui, B. Chen and G. Qian, Coord. Chem. Rev., 2014, 273, 76 CrossRef;
(b) L. V. Meyer, F. Schoenfeld and K. Mueller-Buschbaum, Chem. Commun., 2014, 50, 8093 RSC.
-
(a) D. Gatteschi and R. Sessoli, Angew. Chem., Int. Ed., 2003, 42, 268 CrossRef CAS PubMed;
(b) D. N. Woodruff, R. E. P. Winpenny and R. A. Layfield, Chem. Rev., 2013, 113, 5110 CrossRef CAS PubMed.
-
(a) X. Zhang, V. Vieru, X. Feng, J.-L. Liu, Z. Zhang, B. Na, W. Shi, B.-W. Wang, A. K. Powell, L. F. Chibotaru, S. Gao, P. Cheng and J. R. Long, Angew. Chem., Int. Ed., 2015, 54, 9861 CrossRef CAS PubMed;
(b) D.-D. Yin, Q. Chen, Y.-S. Meng, H.-L. Sun, Y.-Q. Zhang and S. Gao, Chem. Sci., 2015, 6, 3095 RSC;
(c) B. Na, X.-J. Zhang, W. Shi, Y.-Q. Zhang, B.-W. Wang, C. Gao, S. Gao and P. Cheng, Chem. – Eur. J., 2014, 20, 15975 CrossRef CAS PubMed;
(d) J. J. Baldoví, E. Coronado, A. Gaita-Ariño, C. Gamer, M. Giménez-Marqués and G. M. Espallargas, Chem. – Eur. J., 2014, 20, 10695 CrossRef PubMed;
(e) D. Savard, P. H. Lin, T. J. Burchell, I. Korobkov, W. Wernsdorfer, R. Clérac and M. Murugesu, Inorg. Chem., 2009, 48, 11748 CrossRef CAS PubMed;
(f) F. R. Fortea-Perez, J. Vallejo, M. Julve, F. Lloret, G. De Munno, D. Armentano and E. Pardo, Inorg. Chem., 2013, 52, 4777 CrossRef CAS PubMed;
(g) M. Chen, E. C. Sãnudo, E. Jiménez, S.-M. Fang, C.-S. Liu and M. Du, Inorg. Chem., 2014, 53, 6708 CrossRef CAS PubMed;
(h) X. Yi, G. Calvez, C. Daiguebonne, O. Guillou and K. Bernot, Inorg. Chem., 2015, 54, 5213 CrossRef CAS PubMed;
(i) J. Zhao, G.-H. Zhu, L.-Q. Xie, Y.-S. Wu, H.-L. Wu, A.-J. Zhou, Z.-Y. Wu, J. Wang, Y.-C. Chen and M.-L. Tong, Dalton Trans., 2015, 44, 14424 RSC;
(j) Q.-Y. Liu, Y.-L. Li, Y.-L. Wang, C.-M. Liu, L.-W. Ding and Y. Liu, CrystEngComm, 2014, 16, 486 RSC;
(k) C.-M. Liu, D.-Q. Zhang and D.-B. Zhu, RSC Adv., 2014, 4, 36053 RSC;
(l) C.-M. Liu, D.-Q. Zhang and D.-B. Zhu, RSC Adv., 2015, 5, 63186 RSC;
(m) C.-M. Liu, D.-Q. Zhang, X. Hao and D.-B. Zhu, RSC Adv., 2015, 5, 92980 RSC;
(n) C.-M. Liu, J. Xiong, D.-Q. Zhang, X. Hao, B.-W. Wang and D.-B. Zhu, RSC Adv., 2015, 5, 104854 RSC;
(o) C.-M. Liu, D.-Q. Zhang and D.-B. Zhu, Chem. Commun., 2016, 52, 4804 RSC;
(p) Q. Zhou, F. Yang, B. Xin, G. Zeng, X. Zhou, K. Liu, D. Ma, G. Li, Z. Shi and S. Feng, Chem. Commun., 2013, 49, 8244 RSC;
(q) S. Zhang, H. Ke, X. Liu, Q. Wei, G. Xie and S. Chen, Chem. Commun., 2015, 51, 15188 RSC;
(r) X. Ma, N. Xu, C. Gao, L. Li, B. Wang, W. Shi and P. Cheng, Dalton Trans., 2015, 44, 5276 RSC.
-
(a) T. Devic, C. Serre, N. Audebrand, J. Marrot and G. Férey, J. Am. Chem. Soc., 2005, 127, 12788 CrossRef CAS PubMed;
(b) Z. Lin, R. Zou, J. Liang, W. Xia, D. Xia, Y. Wang, J. Lin, T. Hu, Q. Chen, X. Wang, Y. Zhao and A. K. Burrell, J. Mater. Chem., 2012, 22, 7813 RSC;
(c) B. Mu, F. Li, Y. Huang and K. S. Walton, J. Mater. Chem., 2012, 22, 10172 RSC;
(d) H. Zhang, X. Shan, Z. Ma, L. Zhou, M. Zhang, P. Lin, S. Hu, E. Ma, R. Lia and S. Du, J. Mater. Chem. C, 2014, 2, 1367 RSC;
(e) H. Xu, C.-S. Cao and B. Zhao, Chem. Commun., 2015, 51, 10280 RSC;
(f) V. Guillerm, Ł. J. Weseliński, Y. Belmabkhout, A. J. Cairns, V. D'Elia, Ł. Wojtas, K. Adil and M. Eddaoudi, Nat. Chem., 2014, 6, 673 CrossRef CAS PubMed.
- J.-H. Qin, B. Ma, X.-F. Liu, H.-L. Lu, X.-Y. Dong, S.-Q. Zang and H.-W. Hou, Dalton Trans., 2015, 44, 14594 RSC.
- D. Casanova, M. Llunell, P. Alemany and S. Alvarez, Chem. – Eur. J., 2005, 11, 1479 CrossRef CAS PubMed.
- T. Fujii, K. Kodaira, O. Kawauchi, N. Tanaka, H. Yamashita and M. Anpo, J. Phys. Chem. B, 1997, 101, 10631 CrossRef CAS.
-
(a) A. Mishra, W. Wernsdorfer, K. A. Abboud and G. Christou, J. Am. Chem. Soc., 2004, 126, 15648 CrossRef CAS PubMed;
(b) M. Moragues-Cánovas, E. Riviere, L. Ricard, C. Paulsen, W. Wernsdorfer, G. Rajaraman, E. K. Brechin and T. Mallah, Adv. Mater., 2004, 16, 1101 CrossRef.
- N. F. Chilton, D. Collison, E. J. L. McInnes, R. E. P. Winpenny and A. Soncini, Nat. Commun., 2013, 4, 2551 Search PubMed.
-
J. A. Mydosh, Spin Glasses, An Experimental Introduction, Taylor and Francis, London, 1993 Search PubMed.
-
(a) M. Grahl, J. Kotzler and I. Sessler, J. Magn. Magn. Mater., 1990, 90–91, 187 CrossRef CAS;
(b) Y.-N. Guo, G.-F. Xu, P. Gamez, L. Zhao, S.-Y. Lin, R. Deng, J. Tang and H.-J. Zhang, J. Am. Chem. Soc., 2010, 132, 8538 CrossRef CAS PubMed;
(c) C.-M. Liu, D.-Q. Zhang and D. B. Zhu, Inorg. Chem., 2013, 52, 8933 CrossRef CAS PubMed;
(d) C.-M. Liu, D.-Q. Zhang and D. B. Zhu, Dalton Trans., 2013, 42, 14813 RSC.
-
(a) M. Jeletic, P. H. Lin, J. J. L. Roy, I. Korobkov, S. I. Gorelsky and M. Murugesu, J. Am. Chem. Soc., 2011, 133, 19286 CrossRef CAS PubMed;
(b) F. Habib, J. Long, P.-H. Lin, I. Korobkov, L. Ungur, W. Wernsdorfer, L. F. Chibotaruc and M. Murugesu, Chem. Sci., 2012, 3, 2158 RSC;
(c) J. Ruiz, A. J. Mota, A. Rodríguez-Diéguez, S. Titos, J. M. Herrera, E. Ruiz, E. Cremades, J. P. Costes and E. Colacio, Chem. Commun., 2012, 48, 7916 RSC.
Footnote |
† Electronic supplementary information (ESI) available: Supporting structural and magnetic characterization (Fig. S1–S16 and Tables S1–S3). CCDC 1474035 (1) and 1474036 (2). For ESI and crystallographic data in CIF or other electronic format see DOI: 10.1039/c6qi00137h |
|
This journal is © the Partner Organisations 2016 |
Click here to see how this site uses Cookies. View our privacy policy here.