DOI:
10.1039/C6NR08675F
(Communication)
Nanoscale, 2017,
9, 91-98
Sub-6 nm monodisperse hexagonal core/shell NaGdF4 nanocrystals with enhanced upconversion photoluminescence†
Received
6th November 2016
, Accepted 23rd November 2016
First published on 24th November 2016
Abstract
The ability to fabricate lanthanide-doped upconversion nanocrystals (UCNCs) with tailored size and emission profile has fuelled their uses in a broad spectrum of biological applications. Yet, limited success has been met in the preparation of sub-6 nm UCNCs with efficient upconversion photoluminescence (UCPL), which enable high contrast optical bioimaging with minimized adverse biological effects entailed by size-induced rapid clearance from the body. Here, we present a simple and reproducible approach to synthesize a set of monodispersed hexagonal-phase core NaGdF4:Yb/Ln (Ln = Er, Ho, Tm) of ∼3–4 nm and core/shell NaGdF4:Yb/Ln@NaGdF4 (Ln = Er, Ho, Tm) UCNCs of ∼5–6 nm. We show that the core/shell UCNCs can be up to ∼1000 times more efficient than the corresponding core UCNCs due to the effective suppression of surface-related quenching effects for the core. The observation of prolonged PL lifetime for the core/shell than that for the core UCNCs demonstrates the role of the inert shell layer for the protection of the core. The achievement of sub-6 nm NaGdF4 UCNCs with significantly improved luminescence efficiency constitutes a solid step towards high contrast UCPL optical imaging with secured biological safety.
1. Introduction
Lanthanide-doped upconversion nanocrystals (UCNCs) are able to convert long wavelength near-infrared (NIR) excitation into short wavelength luminescence (e.g., shorter NIR, visible or ultraviolet) through the use of real ladder-like energy levels of trivalent lanthanide ions incorporated in an appropriate inorganic host lattice.1,2 Typically, they utilize the sensitizer Yb3+ ions to absorb the NIR light, and then transfer the energy to the activator Ln3+ (Ln = Er, Tm, and Ho, etc.) ions to produce upconversion photoluminescence (UCPL). Owing to their unique optical properties, UCNCs are promising for 3-D displays,3,4 photovoltaics,5 drug delivery,6 biosensing,7 photodynamic therapy,8 and especially for bioimaging. Among the reported UCNCs, paramagnetic Gd3+-containing fluoride nanoparticles are of particular interest, as they not only can be used for optical imaging, but can also be used as contrast agents for magnetic resonance imaging (MRI).9,10 Like the hexagonal NaYF4 host lattice, the hexagonal NaGdF4 has been known to be one of the most efficient upconversion matrices due to its low phonon cutoff energy that can effectively reduce nonradiative losses, and in turn result in high UCPL.11,12 Lanthanide-doped NaGdF4 UCNCs with size ranging from 10 to 60 nm have been prepared by using several solution-based chemical approaches, such as, hydrothermal, thermolysis, co-precipitation, and sol–gel methods.13,14 They have also been successfully demonstrated for uses in single molecule imaging, bimodal (UCPL and MRI) imaging, multimodal (UCPL, MRI, and PET) imaging, and imaging-guided therapeutics.15–18 Despite these successes, the size of the reported UCNCs is not optimal for their use as bioimaging probes, as it prevents them from body clearance. Moreover, these inorganic nanocrystals are not biodegradable; the accumulation of these contrast agents in organs or blood vessels for a long time will possibly elicit serious adverse inflammation effects or even lead to deadly toxic effects. It has been shown that inorganic nanoparticles with size ≤6 nm can be rapidly cleared through a renal route from the body after completing their intended roles.19–21 However, the preparation of sub-6 nm monodisperse hexagonal NaGdF4 nanomaterials with efficient UCPL remains elusive until this point. This demands not only a synthetic approach to confine the resulting nanocrystal size to be <6 nm, but also the ability to address the concomitant evanescing UCPL caused by the exceptionally high surface-related quenching effect.
The surface-related UCPL quenching is a consequence of the reduction of the particle size to the nanoscale regime. This is because UCNCs have a large “surface-to-volume” ratio that can expose numerous sensitizer (Yb3+) and activator (Ln3+) ions directly to the surface quenching effects (surface defects, surface strains, ligand and solvent molecules with groups possessing high vibration energy).22,23 Moreover, the energy contained by Yb3+ ions far from the nanocrystal surface can migrate through the Yb sub-lattice (or the Yb–Yb network) to the surface quenching centers, thus quenching UCPL through an indirect way. The extent of surface-related UCPL quenching is in a reverse proportion to the size of UCNCs that determines its “surface-to-volume” ratio. For UCNCs with size less than 6 nm that enables efficient renal clearance, nearly all the sensitizer and activators incorporated in the lattice experience strong surface-related deactivations, which results in a near-complete quenching of UCPL, making their applications in optical bioimaging impossible. The core/inert shell structure has been shown to be an effective strategy to address surface-related quenching due to the passivation of lattice defects on the surface, and the shell-enabled spatial isolation of the core nanocrystal from the environment.24–30 However, application of the core/shell strategy to ultrasmall UCNCs, while retaining the size of the entire core/shell nanostructure to be less than 6 nm, remains a great challenge, as this posts a demanding requirement of a precise control over the growth process of the core and the shell layer.
Herein, we report on the controlled synthesis of 3–4 nm sized monodispersed hexagonal NaGdF4:Yb/Ln (Ln = Er, Ho, Tm) UCNCs through co-precipitaion of metallic and fluoride precursors in a high-boiling mixture of oleic acid (OA) and octadecene (ODE), and then on the seed-mediated growth of 5–6 nm sized monodispersed hexagonal NaGdF4:Yb/Ln@NaGdF4 core@shell UCNCs through thermolysis of sodium and gadolim trifluoacetate in the same mixture of OA/ODE. We found that growth of an ∼1 nm thin NaGdF4 shell layer is able to efficiently suppress the surface-related quenching mechanism, and thus enhance the UCPL of the core UCNCs by up to ∼1000 fold.
2. Experimental section
2.1 Materials
Rare-earth (RE) chloride hexahydrate (GdCl3·6H2O, YbCl3·6H2O, ErCl3·6H2O, HoCl3·6H2O, TmCl3·6H2O 99.9%, Aladdin), oleic acid (OA, AR, Sinopharm Chemical Reagent Co., Ltd), 1-octadecene (ODE, 90%, Aladdin), sodium hydroxide (NaOH 96.0%, Xi Long Chemical Co., Ltd China), ammonium fluoride (NH4F 96.0%, Tianjin Xinbote Chemical Co., Ltd China), methanol (MeOH AR, XiLong Chemical Co., Ltd China), gadolinium oxide (99.9%, Aladdin), trifluoroacetic acid (TFA 99%, Energy Chemical), sodium trifluoroacetic acid (NaTFA 97%, Aladdin), hexane (C6H14 AR, Xi Long Chemical Co., Ltd China), and ethanol were used as received without further purification.
2.2 Synthesis of monodisperse β-NaGdF4:Yb/Ln core nanocrystals
The β-NaGdF4:x%Yb/y%Ln (Ln = Er, Tm, and Ho) UCNCs were synthesized via a co-precipitation procedure adapted from the literature,31 involving the nucleation of NaGdF4 at a low temperature via diffusion of metallic ions, and then the Ostwald -ripening process at a higher temperature. The combined molar amount of GdCl3·6H2O (100 − x/100 − y/100 mmol), YbCl3·6H2O (x/100 mmol), and LnCl3·6H2O (y/100 mmol) precursors was set to a constant of 1 mmol. The x and y values vary with the intended doping amount of the sensitizer Yb and the activator Ln. Considering the synthesis of NaGdF4:30%Yb/2%Er for example, GdCl3·6H2O (0.68 mmol), YbCl3·6H2O (0.3 mmol), ErCl3·6H2O (0.02 mmol), oleic acid (9 ml), and 1-octadecene (15 ml) were loaded together into a three-necked flask (250 ml). The mixture was heated to 160 °C for 1 h under gentle Ar gas purge with constant stirring to remove H2O and O2, and then cooled to 50 °C. Subsequently, 10 mL methanol solution containing 4 mmol NH4F and 2.5 mmol NaOH was added drop by drop into the mixture, which was stirred for ∼30 min, and then heated to 100 °C for 30 min to evaporate the methanol. After this, the temperature of this mixture was increased to 240–300 °C at a rate of 10 °C min−1, and then maintained at this temperature for a defined time period (10–40 min) under Ar gas protection. The final solution was cooled to room temperature; the resulting nanoparticles were collected by adding an excess amount of ethanol and then centrifuged at 6000 rpm for 5 min. The precipitate was washed with an appropriate amount of ethanol two more times, and finally dispersed in 10 mL of hexane for further use.
2.3 Synthesis of monodisperse β-NaGdF4:Yb/Ln@NaGdF4 core/shell nanocrystals
The β-NaGdF4:x%Yb/y%Ln@NaGdF4 (Ln = Er, Tm, and Ho) core/shell nanocrystals were prepared using a seed-mediated growth protocol. The seed β-NaGdF4:x%Yb/y%Ln core nanocrystals were synthesized using the above-mentioned procedure at a fixed temperature of 240 °C for 20 min. In a typical seed-mediated protocol for synthesizing core/shell UCNCs, first, the shell precursor of Gd(CF3COO)3 (1 mmol) was prepared via dissolving Gd2O3 (0.5 mmol) in 50% trifluoroacetic acid (10 mL) at 95 °C in a 250 mL three-necked flask, evaporating to dryness under argon gas protection, and then cooling down to room temperature. Second, the as-prepared seed β-NaGdF4:x%Yb/y% core (1 mmol, counted according to the amount of metallic ions) in 10 ml hexane, oleic acid (10 ml), 1-octadecene (15 ml), and sodium trifluoroacetic acid (1 mmol) was added into the flask. The temperature of the solution was then increased to 120 °C and maintained at this temperature for 30 min to remove hexane, water and oxygen. Finally, the resulting solution was heated to 240 °C and maintained at this temperature for 20 min, before naturally cooling down to room temperature. Finally, a sufficient amount of ethanol (∼20 mL) was added into the flask and the mixed solution was centrifuged at 6000 rpm for 5 min to get the precipitate. The collected precipitate was further washed with ethanol twice, and finally dispersed in 10 mL of hexane for further use.
2.4 Ligand exchange of oleic acid with polyacrylic acid
In a typical procedure, a mixture of DEG (8.0 mL) and PAA (0.1 g) was heated to 110 °C with vigorous stirring under N2 flow. Then, 2 mL chloroform solution of NaGdF4:30%Yb/2%Er@NaGdF4 (50 mg mL−1) were injected and the system was heated to 240 °C for 10 min. During this process, the initial milky solution gradually became clear. After cooling to room temperature, an excess of dilute aqueous hydrochloric solution was added into the solution until a white precipitate is seen. The precipitate was collected by centrifugation, washed three times with pure water, and neutralized with a diluted solution of sodium hydroxide. Finally, the NaGdF4:30%Yb/2%Er@NaGdF4 UCNCs were dispersed in deionized water.
2.5 Characterization
The size and morphology of NaGdF4:Yb/Ln (Ln = Er, Tm, and Ho) nanocrystals and NaGdF4:Yb/Ln@NaGdF4 core/shell nanocrystals were characterized by transmission electron microscopy (TEM) using a JEOL JEM-2010 microscope at an acceleration voltage of 200 kV. The powder X-ray diffraction (XRD) patterns were recorded by using a Siemens D500 diffractometer, using Cu Kα radiation (λ = 0.15418 nm). The 2θ angle of the XRD spectra was recorded at a scanning rate of 5° min−1. The UCPL spectra were obtained using a Zolix monochoromator (Beijing Zolix Instruments Co., Ltd) under excitation at 976 nm using a fiber-coupled laser diode (BWT Beijing Ltd). The decay profiles at 540 nm for the core and the core/shell UCNCs were measured by square-wave-modulation of the electric current input to the laser diode and by recording the signals via a Tektronix TDS 5052 digital oscilloscope with a lock-in preamplifier (Stanford Research System Model SR830 DSP) employing a chopping rate of 3000 rps. Photographic UCPL images of colloidal nanocrystals were taken by using a digital camera (Canon Power Shot SX100 IS) without adding any filter.
3. Results and discussion
3.1 Control of the size of the hexagonal NaGdF4 core nanoparticles
To precisely control the resulting size of the hexagonal NaGdF4:Yb/Ln core nanoparticles, we have explored systematic variation of two synthetic parameters, i.e., the reaction temperature and the reaction time period, during their growth process in solution. The effect of synthetic temperature on the resulting nanoparticle size is revealed in TEM images shown in Fig. 1. As one can see, along with a decrease of temperature from 300 to 240 °C, the size of NaGdF4:30%Yb/2%Er nanocrystals gradually decreased from 10.50 to 3.63 nm (see Fig. S1† for the corresponding histograms of size distribution). Moreover, nanoparticles prepared at different temperatures are all shown to be monodispersed and have a nearly spherical shape. This observation suggests that the low temperature reaction thermodynamically favors the generation of NaGdF4 nanoparticles with a smaller size. It is known that the formation of NaGdF4 nanocrystals typically includes two growth stages: nucleation and crystal growth, while the growth stage typically plays the most significant role in defining the size of the resulting nanoparticle. Providing low temperature to the reaction system will kinetically decrease the odds of collision of the nuclei with diffused monomers (the NaGdF4 molecule) in the solution system for growth, thus confining the size of the synthesized nanoparticle to be smaller. The role of controlling the growth process to confine the nanoparticle size was also confirmed by the result of the synthesized NaGdF4:30%Yb/2%Er nanoparticles at fixed temperature of 240 °C, yet with different reacting time periods (Fig. 2, and S2 in the ESI†). As one can see in Fig. 2, the size of NaGdF4 can be effectively reduced from 4.44, 3.90, 3.86, to 3.63 nm when shortening the reaction time from 50, 40, 30 to 20 min, respectively.
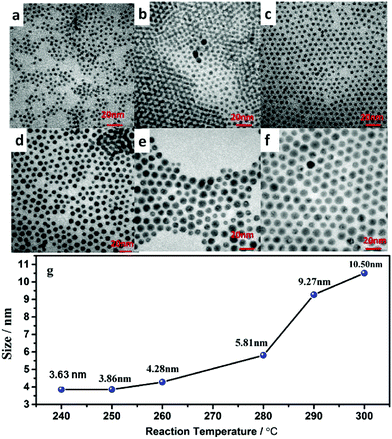 |
| Fig. 1 Transmission electron microscopy (TEM) images of NaGdF4:30%Yb/2%Er nanoparticles obtained at different reaction temperatures of (a) 240 °C, (b) 250 °C, (c) 260 °C, (d) 280 °C, and (e) 290 °C, and (f) 300 °C, respectively. The reaction time is fixed at ∼20 min. | |
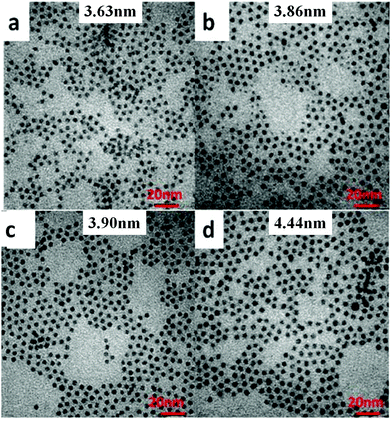 |
| Fig. 2 Transmission electron microscopy (TEM) images of NaGdF4:30%Yb/2%Er nanoparticles obtained at different reaction times, (a) 20 min, (b) 30 min, (c) 40 min and (d) 50 min. (Reaction temperature, 240 °C). | |
3.2 Control of ultrasmall NaGdF4:30%Yb/2%Er@NaGdF4 core/shell nanocrystals
The size and morphology of the synthesized NaGdF4:30%Yb/2%Er core nanoparticles (Fig. 3a, synthesized at 260 °C for 20 min) and the core/shell NaGdF4:30%Yb/2%Er@NaGdF4 UCNCs (Fig. 3c, synthesized at 240 °C for 20 min for the shell growth) were examined by transmission electron microscopy (TEM). As one can see in Fig. 3a, the prepared NaGdF4:30%Yb/2%Er nanoparticles appear nearly monodisperse and uniform, while Fig. 3d reveals that the resulting core/shell NaGdF4:30%Yb/2%Er@NaGdF4 nanocrystals retain the morphology and uniformity of the core nanocrystals. The histograms of the size distribution of the synthesized core (Fig. 3b) and core/shell nanocrystals (Fig. 3e) indicate that the average diameter is about 4.28 nm with a standard deviation of 0.51 nm for the core UCNCs, while being ∼5.81 nm with a standard deviation of 0.67 nm for the core/shell UCNCs. The data for the size distribution were obtained from the TEM images of over 200 nanocrystals. The increase in the particle size and the retainment of the uniformity for the core/shell UCNCs compared to the core implies the successful growth of a core/shell nanostructure, and the thickness of the shell is estimated to be about 0.77 nm. Moreover, the energy-dispersive X-ray spectra (EDX) show that the core/shell nanoparticles have a stronger signal of Gd3+ ions compared to the core nanoparticles, also implying the formation of the core/shell structure (Fig. S3, ESI†). The selected area electron diffraction (SAED) patterns indicate that both the core (Fig. 3c) and the core/shell (Fig. 3f) nanocrystals have a crystallographic structure of a hexagonal phase. The formation of a low-symmetry hexagonal phase is beneficial for the UCPL output, as the hexagonal phase can typically be about one order more efficient than the cubic phase.32
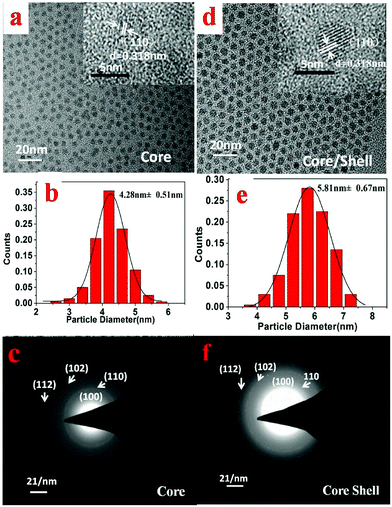 |
| Fig. 3 Transmission electron microscopy images of the NaGdF4:30%Yb/2%Er core nanoparticles (a), and the NaGdF4:30%Yb/2%Er@NaGdF4 core/shell nanoparticles (d), insets in (a) and (d) show the high-magnification TEM images of NaGdF4:30%Yb/2%Er core and NaGdF4:30%Yb/2%Er@NaGdF4 core/shell. (b) and (e) show the size distribution histograms of the core NaGdF4:30%Yb/2%Er and the core/shell NaGdF4:30%Yb/2%Er@NaGdF4 nanoparticles, respectively. The selected area electron diffraction (SAED) patterns of the NaGdF4:30%Yb/2%Er core nanocrystals and NaGdF4:30%Yb/2%Er@NaGdF4 core/shell nanoparticles are presented in (c) and (f), respectively. | |
The crystallinity and crystal phase of both the NaGdF4:30%Yb/2%Er core and the NaGdF4:30%Yb/2%Er@NaGdF4 core/shell UCNCs were further confirmed by their XRD patterns (Fig. 4). It is evident from the intensities of the diffraction peaks that both the core and core/shell UCNCs are highly crystalline in nature. Moreover, the peak positions from the XRD patterns of both the core and the core/shell UCNCs match well with the standard JCPDS 27-0699 pattern of β-NaGdF4, further substantiating that the resulting core and core/shell UCNCs are of the hexagonal phase. Note that no peaks from the other phases or impurities have been observed. These observations are in good agreement with the SAED results shown in Fig. 3c and f. We would like to point out that a slight shift of the XRD peaks towards a high-angle was observed for the NaGdF4:30%Yb/2%Er core and the NaGdF4:30%Yb/2%Er@NaGdF4 core/shell nanocrystals when compared to that of the standard pattern. This is mainly attributed to the decrease of a unit-cell volume, resulting from the replacement of Gd3+ (0.938 Å) by Yb3+ with smaller ion radii (0.868 Å).
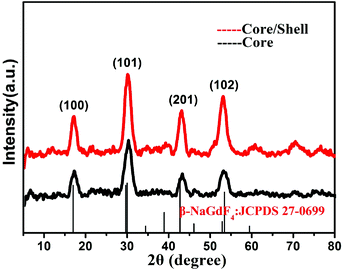 |
| Fig. 4 The XRD patterns of the NaGdF4:30%Yb/2%Er@NaGdF4 core/shell nanocrystals, and the NaGdF4:30%Yb/2%Er core, in reference to the standard diffraction pattern of the hexagonal phase NaGdF4 (JCPDS 27-0699). | |
3.3 Optical characterization of the NaGdF4:30%Yb/2%Er core and NaGdF4:30%Yb/2%Er@NaGdF4 core/shell nanocrystals
As one can see from Fig. 5a, when excited at 980 nm, two UC bands with maxima at 525/540 nm and 660 nm can be observed for both the NaGdF4:30%Yb/2%Er core and the NaGdF4:30%Yb/2%Er@NaGdF4 core/shell UCNCs, which correspond to the 2H11/2/4S3/2 → 4I15/2 and 4F9/2 → 4I15/2 transitions of Er3+ ions. Moreover, the intensity of UCPL for the core/shell UCNCs is dramatically higher than that of the core nanoparticles. The intensity of UCPL bands at 525, 540 and 660 nm for the NaGdF4:30%Yb/2%Er@NaGdF4 core/shell nanocrystals was found to be about 918, 1185 and 266 times higher than that of NaGdF4:30%Yb/2%Er core nanocrystals, respectively. The integrated UCPL intensity (over spectral range of 400–700 nm) for the core/shell UCNCs was determined to be about ∼977 folds higher than that of the 4.28 nm sized core nanocrystal. We also compared the UCPL intensity of the 5.81 nm sized NaGdF4:30%Yb/2%Er@NaGdF4 core/shell nanoparticles with 10.50 nm sized NaGdF4:30%Yb/2%Er core nanocrystals (Fig. S8†). We found that the integrated UCPL intensity of the 5.8 nm sized core/shell UCNCs was measured to be about ∼128 folds higher than that of the 10.5 nm sized core NaGdF4:30%Yb/2%Er nanocrystals. This result further confirms that the core/shell strategy is able to largely suppress surface-related quenching effects of the core, resulting in significant UCPL enhancement.
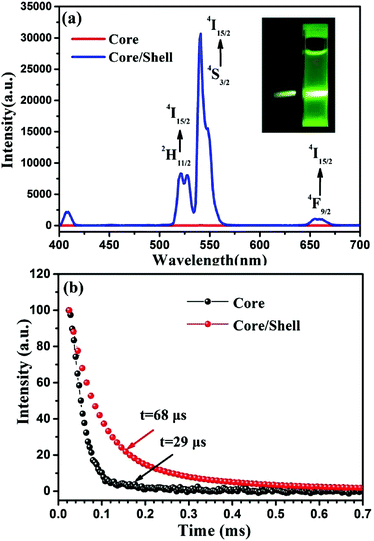 |
| Fig. 5 (a) The UCPL spectra of the NaGdF4:30%Yb/2%Er core and the NaGdF4:30%Yb/2%Er@NaGdF4 core/shell nanocrystals (hexane suspension; excited at 980 nm of 10 W cm−2); the inset shows the corresponding UCPL photographic images of the core and core/shell nanocrystals. (b) PL decays at 540 nm for the NaGdF4:30%Yb/2%Er core and the NaGdF4:30%Yb/2%Er@NaGdF4 core/shell nanoparticles (hexane dispersion; excited at 980 nm). | |
The exceptionally high surface/volume ratio expose a majority of doped lanthanide ions to surface quenching centers (such as surface impurities, ligands, and solvents), eliciting nonraditaive transfer of the upconverted energy to the surface quenching centers and thus quenching UCPL heavily (as confirmed by the observation in Fig. 5a).22,33–35 The significant enhancement of the intensity of UCPL indicates that the growth of a thin layer successfully suppresses such surface-quenching effects, making these ultrasmall nanocrystals much more efficient for potential use in bioapplications. To confirm this, we acquired the PL decays at 540 nm (corresponding to the 4S3/2 → 4I15/2 transition of Er) for nanocrystals of the NaGdF4:Yb30%/Er2% core and the NaGdF4:Yb30%/Er2%@NaGdF4 core/shell. A longer lifetime of 68 μs was observed for the core/shell nanocrystals in comparison with the 29 μs for core nanocrystals (Fig. 5b), confirming that the nonradiative pathways to dissipate upconverted energy at the nanocrystal surface indeed has been hampered by the shell layer through spatial isolation of doped lanthanide ions in the core from the surrounding quenching sites. In addition, these ultrasmall UCNCs can be successfully transferred from the organic phase to the aqueous phase utilizing a polyacrylic acid ligand exchange procedure described in our previous work.36 The UCPL intensity was found to be retained merely with a slight decrease after the ligand exchange (Fig. S9 and S10†).
To investigate the upconversion mechanism for the core/shell NaGdF4:30%Yb/2%Er@NaGdF4 UCNCs, the pump power-dependence of green and red UC emissions was measured and is presented in Fig. 6a. It is known that for an unsaturated state, the number of photons that are required to populate the upper emitting state can be obtained by the relationship If ∝ Pn, where If is the fluorescent intensity, P is the pump laser power and n is the number of the laser photons required. A logarithmic plot of Ifversus P enables retrieving the n values from the slope of the linear fitting of the experimental data. According to Fig. 6a, n = 2.23, 2.31 and 1.93 were obtained for the 2H11/2 → 4I15/2, 4S3/2 → 4I15/2 and 4F9/2 → 4I15/2 transitions, respectively. These values suggest that two-photon processes are involved to populate the 2H11/2, 4S3/2 and 4F9/2 states, respectively. Note that n = 2.23, 2.31 is slightly larger than the theoretical value of two; this might suggest that higher-lying energy levels are involved to populate the 2H11/2 and the 4S3/2 states. The upconverting pathways for green and red UCPL are shown in Fig. 6b. Two energy transfers from the sensitizer Yb to the activator Er excite it from the ground state via the intermediate 4I11/2 state to the 4F7/2 state, which can then efficiently populate the green-emitting 2H11/2/4S3/2 state through a multiphonon-mediated nonradiative decay. The red emitting level of the 4F9/2 state can be populated via nonradiative decay from the 4S3/2 state, or by exciting the Er ions at the 4I13/2 state (populated by multiphonon-involved nondiative decay from the 4I11/2 state) through an energy transfer from the excited Yb ion.
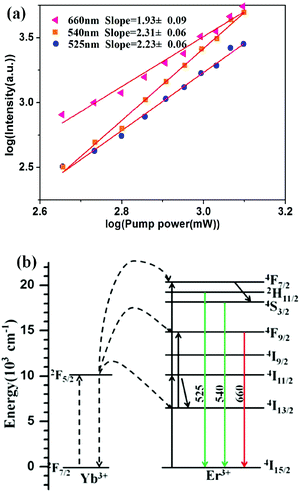 |
| Fig. 6 (a) Logarithmic plots of the dependence of the intensity of green and red emissions from NaGdF4:30%Yb/2%Er@NaGdF4 nanocrystals on the pumping laser power. (b) Energy level diagrams of Yb and Er ions and the proposed upconversion mechanism in NaGdF4:30%Yb/2%Er@NaGdF4 nanocrystals. | |
3.4 Optical characterization of NaGdF4:20%Yb/2%Ho@NaGdF4 core/shell and NaGdF4:30%Yb/1%Tm@NaGdF4 core/shell nanoparticles
To test whether the core/shell strategy for UCPL enhancement can be validated for sub-6 nm nanocrystals doped with other types of activators, we synthesized hexagonal phase NaGdF4:20%Yb/2%Ho core, NaGdF4:20%Yb/2%Ho@NaGdF4 core/shell, NaGdF4:30%Yb/1%Tm core, and NaGdF4:30%Yb/1%Tm@NaGdF4 UCNCs, respectively. The TEM images of NaGdF4:20%Yb/2%Ho core, NaGdF4:20%Yb/2%Ho@NaGdF4 core/shell, NaGdF4:30%Yb/1%Tm core, and NaGdF4:30%Yb/1%Tm@NaGdF4 core/shell UCNCs are shown in Fig. S4 and S6 in the the ESI.† The corresponding XRD results demonstrated that the as-synthesized core and core/shell UCNCs with varying types of dopants (Ho or Tm) were also of the hexagonal NaGdF4 structure (Fig. S5 and S7, ESI†). Measured TEM results indicate that the mean size is about 3.15 and 5.05 nm for the NaGdF4:20%Yb/2%Ho core and the NaGdF4:20%Yb/2%Ho@NaGdF4 core/shell nanocrystals, respectively; and about 3.90 and 4.75 nm for the NaGdF4:30%Yb/1%Tm core, and the NaGdF4:30%Yb/1%Tm@NaGdF4 core/shell nanocrystals, respectively. A comparison of UCNPL of the core and the corresponding core/shell nanocrystal with emitters Ho or Tm is shown in Fig. 7. As illustrated in Fig. 7a, three UCPL peaks at 485, 540 and 645 nm were observed, corresponding to the transitions 5F3 → 5I8, 5S2 → 5I8, and 5F5 → 5I8 of Ho ions, respectively. The growth of a thin shell (0.95 nm) on the top of the NaGdF4:20%Yb/2%Ho core nanoparticles enhances its UCPL by about 21, 42 and 63 times for the band at 485, 540 and 645 nm, respectively. This results in a total UCPL enhancement of about 44 times for the spectral range of 400–700 nm. In a similar but a more pronounced way, the growth of a thin NaGdF4 shell onto the NaGdF4:30%Yb/1%Tm core nanocrystals enhances its UCPL by about 249, 1476, and 99 times for the bands at 450 (corresponding to the 1D2 → 3F4), 475 (1G4 → 3H6) and 800 nm (3H4 → 3H6) of Tm ions, respectively (Fig. 5b). This results in a total 579 times UCPL enhancement for the NaGdF4:30%Yb/1%Tm@NaGdF4 core/shell than the NaGdF4:30%Yb/Tm1% core UCNCs in the spectral range of 400–900 nm. The involved photon processes as well as mechanisms for the generation of UCPL shown in Fig. 7a and b are described in detail in Fig. S11 and S12 in the ESI.† Considering the results in Fig. 5 and 7, the core/shell strategy can enhance the UCPL of sub-6 nm UCNCs thousands of times, and is independent of the type of the doped activators in the core nanocrystals.
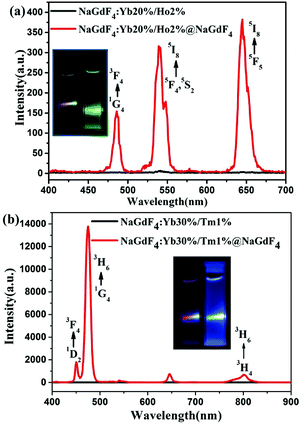 |
| Fig. 7 UCPL spectra from (a) the NaGdF4:20%Yb/2% Ho core and the NaGdF4:20%Yb/2%Ho@NaGdF4 core/shell nanocrystals, and (b) the NaGdF4:30%Yb/1%Tm core and the NaGdF4:30%Yb/1%Tm@NaGdF4 core/shell nanocrystals. The insets show the corresponding UCPL photographic images of the core and core/shell UCNCs. Hexane suspension, excited at 980 nm of 10 W cm−2. | |
4. Conclusions
In summary, the size of the resulting NaGdF4:Yb/Ln core UCNCs can be precisely confined to be 3–4 nm through a simple variation of reaction temperature and time that can explicitly regulate the growth process in the solution phase. Moreover, a seed-mediated growth protocol with selected parameters favorable for thin shell growth leads to the preparation of ultrasmall core/shell NaGdF4:Yb/Ln@NaGdF4 (Ln = Er, Ho, Tm) UCNCs of ∼5–6 nm. Moreover, we found that the growth of the inert thin shell (∼1 nm) of NaGdF4 onto the NaGdF4:Yb/Ln (Ln = Er, Ho, Tm) core UCNCs could enhance its UCPL by up to ∼1000 times. The prolonged PL lifetime for the core/shell nanocrystal compared to that for the core UCNCs demonstrates that such a significant UCPL enhancement arises from an effective suppression of surface-related quenching effects through spatial isolation of the core from the surrounding environment. Sub-6 nm monodisperse core/shell NaGdF4 UCNCs with significantly improved UCPL hold promise for high contrast UCPL optical imaging and multimodal bioimaging with the ability to allow rapid renal clearance from the body.
Acknowledgements
This work was supported by National Natural Science Foundation of China (51672061), National Natural Science Fund for Distinguished Young Scholars (51325201), the Fundamental Research Funds for the Central Universities, China (AUGA5710052614, AUGA8880100415, and HIT.BRETIV.201503). We acknowledge Ms Leipeng Sun and Prof. Zhiguo Zhang from Condensed Matter Science and Technology Institute, Harbin Institute of Technology, for their kind help in measuring the lifetime.
Notes and references
- G. Y. Chen, H. L. Qiu, P. N. Prasad and X. Y. Chen, Chem. Rev., 2014, 114, 5161–5214 CrossRef CAS PubMed.
- F. Wang and X. Liu, Chem. Soc. Rev., 2009, 38, 976–989 RSC.
- F. Auzel, Chem. Rev., 2004, 104, 139–173 CrossRef CAS PubMed.
- J. C. Boyer, N. J. J. Johnson and F. C. J. M. Veggel, Chem. Mater., 2009, 21, 2010–2012 CrossRef CAS.
- J. Wild, A. Meijerink, J. K. Rath, W. G. J. H. M. van Sark and R. E. I. Schropp, Energy Environ. Sci., 2011, 4, 4835–4848 Search PubMed.
- C. Wang, L. Cheng and Z. Liu, Biomaterials, 2011, 32, 1110–1120 CrossRef CAS PubMed.
- D. E. Achatz, R. J. Meier, L. H. Fisher and O. S. Wolfbeis, Angew. Chem., Int. Ed., 2011, 50, 260–263 CrossRef CAS PubMed.
- P. Zhang, W. Steelant, M. Kumar and M. Scholfield, J. Am. Chem. Soc., 2007, 129, 4526–4527 CrossRef CAS PubMed.
- H. B. Na and T. Hyeon, J. Mater. Chem., 2009, 19, 6267–6273 RSC.
- H. B. Na, I. C. Song and T. Hyeon, Adv. Mater., 2009, 21, 2133–2148 CrossRef CAS.
- Y. S. Liu, D. T. Tu, H. M. Zhu, R. F. Li, W. T. Luo and X. Y. Chen, Adv. Mater., 2010, 22, 3266–3271 CrossRef CAS PubMed.
- F. Wang, J. Wang and X. G. Liu, Angew. Chem., Int. Ed., 2010, 49, 7456–7460 CrossRef CAS PubMed.
- F. T. You, Y. X. Wang and J. H. Lin, Chin. J. Inorg. Chem., 2001, 17, 27–31 CAS.
- R. F. Naccache, F. Vetrone and V. Mahalingam, Chem. Mater., 2009, 21, 717–723 CrossRef CAS.
- J. Zhou, Y. Sun, L. Q. Xiong, H. Hu and F. Y. Li, Biomaterials, 2010, 31(12), 3287–3295 CrossRef CAS PubMed.
- R. Kumar, M. Nyk, T. Y. Ohulchanskyy, C. A. Flask and P. N. Prasad, Adv. Funct. Mater., 2009, 19(6), 853–859 CrossRef CAS.
- J. Zhou, M. X. Yu and Y. Sun, Biomaterials, 2011, 32(4), 1148–1156 CrossRef CAS.
- S. Jiang, K. Y. Win and S. H. Liu, Nanoscale, 2013, 5(8), 3127–3148 RSC.
- G. Y. Chen, H. L. Qiu, R. E. Fan and S. W. Hao,
et al.
, J. Mater. Chem., 2012, 22, 20190–20196 RSC.
- X. Y. Jin, F. Fang and J. H. Liu,
et al.
, Nanoscale, 2015, 38, 15680–15688 RSC.
- G. Y. Chen, T. Y. Ohulchanskyy, R. Kumar, H. Ågren and P. N. Prasad, ACS Nano, 2010, 4(6), 3163–3168 CrossRef CAS PubMed.
- T. Rinkel, A. N. Raj, S. Duehnen and M. Haase, Angew. Chem., Int. Ed., 2016, 55, 1164–1167 CrossRef CAS PubMed.
- T. Rinkel, J. Nordmann, A. N. Raj and M. Haase, Nanoscale, 2014, 6, 14523–14530 RSC.
- G. S. Yi and G. M. Chow, Chem. Mater., 2007, 19, 341–343 CrossRef CAS.
- N. J. J. Johnson, A. Korinek, C. H. Dong and F. C. J. M. van Veggel, J. Am. Chem.
Soc., 2012, 134, 11068–11071 CrossRef CAS PubMed.
- J. C. Boyer and F. C. J. M. van Veggel, Nanoscale, 2010, 2, 1417–1419 RSC.
- Y. P. Du, X. Sun, Y. W. Zhang, Z. G. Yan, L. D. Sun and C. H. Yan, Cryst. Growth Des., 2009, 9(4), 2013–2019 CAS.
- D. Q. Chen, M. Xu and P. Huang, Sens. Actuators, B, 2016, 231, 576–583 CrossRef CAS.
- D. Q. Chen, L. Liu, P. Huang, M. Ding, J. Zhong and Z. G. Ji, J. Phys. Chem. Lett., 2015, 6, 2833–2840 CrossRef CAS PubMed.
- D. Q. Chen and P. Huang, Dalton Trans., 2014, 43, 11299–11304 RSC.
- Z. Q. Li and Y. Zhang, Nanotechnology, 2008, 19, 345606 CrossRef PubMed.
- K. W. Krämer, D. Biner, G. Frei, H. U. Güdel, M. P. Hehlen and S. R. Lüthi, Chem. Mater., 2004, 16(7), 1244–1251 CrossRef.
- F. Vetrone, R. Naccache, V. Mahalingam, C. G. Morgan and J. A. Capobianco, Adv. Funct. Mater., 2009, 19(18), 2924–2929 CrossRef CAS.
- H. Schäfer, P. Ptacek, O. Zerzouf and M. Haase, Adv. Funct. Mater., 2008, 18(19), 2913–2918 CrossRef.
- H. X. Mai, Y. W. Zhang, L. D. Sun and C. H. Yan, J. Phys. Chem. C, 2007, 111(37), 13721–13729 CAS.
- J. Shen, G. Chen, T. Y. Ohulchanskyy, S. Kesseli, S. Buchholz, Z. Li, P. N. Prasad and G. Han, Small, 2013, 9, 3213–3217 CrossRef CAS PubMed.
Footnote |
† Electronic supplementary information (ESI) available. See DOI: 10.1039/c6nr08675f |
|
This journal is © The Royal Society of Chemistry 2017 |
Click here to see how this site uses Cookies. View our privacy policy here.