DOI:
10.1039/C7QM00204A
(Research Article)
Mater. Chem. Front., 2017,
1, 2519-2526
Improved microwave absorption and electromagnetic interference shielding properties based on graphene–barium titanate and polyvinylidene fluoride with varying content†
Received
9th May 2017
, Accepted 23rd June 2017
First published on 29th June 2017
Abstract
Being lightweight, broadband, thin and highly efficient are the key desirable properties for microwave absorbers. We have successfully prepared reduced graphene oxide (rGO) coated with barium titanate (BT) using a simple method and a series of measurements were carried out to determine its morphology and structure. The composites of rGO@BT/poly(vinylidene fluoride) (PVDF) with filler amounts of 10, 15, 20 and 25 wt% exhibit broad frequency bands (<−10 dB) and the highest reflection coefficients of up to −33.2, −45.3, −32.9 and −22.8 dB, respectively, in the frequency range of 2–18 GHz with thicknesses of 1–5 mm. Furthermore, the electromagnetic interference (EMI) shielding effectiveness was measured and the theoretical calculations of SEA and SER are displayed for comparison. The electrical conductivity and skin depth are shown as well for further investigation.
1 Introduction
With the rapid development of science and technology, a large number of electronics, instruments and devices, such as cell phones, microwave ovens, printers and so on, are playing increasingly significant roles in our daily life and military affairs, leading to a huge threat to our health. Over the past few decades, researchers have carried out a great deal of studies on microwave absorbers, which can efficiently protect human beings from electromagnetic irradiation. Lightweight, broadband, thin and highly efficient microwave absorbers are much needed in the long term. Moreover, researchers have made considerable endeavours to design microwave absorbers by adjusting the permittivity, permeability and dielectric loss parameters in pursuit of a larger reflection loss.
Carbon nanomaterials, including carbon nanotubes,1,2 carbon black,3–5 carbon nanofibers6 and graphite,7 are expected to display obvious EM attenuation owing to their superior mechanical and electrical properties.8 On the one hand, graphene, with an electrical conductivity of more than 6000 S cm−1 and a high aspect ratio, is favourable for the formation of an effective network inside graphene composites at low graphene content, making it a fascinating candidate in microwave absorption fields.9 On the other hand, pure graphene suffers from inferior interfacial interference impedance from improper conductivity and a limited loss mechanism.10 Reduced graphene oxide contains oxygen functional groups, for example epoxy, hydroxyl, carboxy and carbonyl groups, which means that it can overcome its easily irreversible agglomeration or even restacking as a result of strong van der Waals interactions and the out-of-plane p bond between the individual graphene nanosheets.11 Consequently, a series of materials has been chosen to tune permittivity and permeability values independently to match the requirements of the microwave absorption mechanism.12–14 For example, Vivek et al.15 revealed that 10 wt% of graphene oxide in an NBR matrix exhibits high values of reflection loss (>10 dB) over a wide frequency range (7.5–12 GHz) and its maximum loss is 57 dB at 9.6 GHz at a thickness of 3 mm. Zhang et al.16–19 have studied a great many novel microwave absorption composites which display relatively higher reflection loss values and wider wavelength coverage over a frequency of 2–18 GHz. Both the dielectric loss and magnetic loss of CoFe@RGO,16 FeNR@RGO,17 CoNS@RGO18 are responsible for their superior microwave absorption properties.
Barium titanate (BT), a type of dielectric material, is widely applied in ceramic capacitors, transducers, thermistors and electro-optic devices. According to Chen, the dielectric constant (ε) value of BT decreases with increasing frequency and a peak in the dielectric loss (tan
δ) appears at gigahertz (GHz) frequency, indicating that a relaxation phenomenon occurs in BT materials. Thus, it is natural to speculate that BT might be useful as a microwave absorber.20 Zhu and co-workers21 discovered that the microwave absorption properties of BT nanotubes should be studied at microwave frequencies of between 0.5 and 15 GHz. The maximum reflection loss of the 70 wt% BT nanotubes/paraffin wax composite reached 21.8 dB at 15 GHz, and the frequency bandwidth less than −10 dB was from 13.3 to 15 GHz.
In this work, instead of conventional paraffin, poly(vinylidene fluoride) (PVDF) was chosen as a special matrix owing to its properties of good strength and resistance to solvents, acids, bases and heat.22 The synergistic effect between fillers and matrices may be helpful to enhance absorption abilities. On the other hand, the introduction of PVDF, which makes the composite flexible allowing its shape to be tailored as needed, would greatly expand the applications of the sample, especially for materials and devices with transformable or complicated structures. Polymer and inorganic composites are expected to possess outstanding microwave absorption properties. Herein, rGO@BT/PVDF composites with homogeneous structures were prepared using a simple method described in this paper. We investigated the properties of wave absorption in detail with different amounts of filler and the mechanism of the enhanced absorption was also studied. Furthermore, the electromagnetic interference (EMI) shielding effectiveness was measured and the theoretical calculations of SEA and SER are displayed for comparison. The electrical conductivity and skin depth are shown as well for further investigation.
2 Experimental
2.1 Materials
Graphite powder was purchased from Alfa Aesar. Barium titanate powders (diameter ∼100 nm, 99.9%), potassium permanganate (KMnO4), sulfuric acid (H2SO4, 98%), hydrogen peroxide (H2O2) and N,N-dimethylformamide (DMF) were purchased from Beijing Chemical Works; PVDF powders.
2.2 Composite preparation
The surface hydroxylation of barium titanate.
15 g of barium titanate nanoparticles and 350 mL of H2O2 (30%) were combined in a round-bottomed flask and sonicated for 20 min. The mixture was refluxed at 106 °C for 2 h and the nanoparticles were recovered by centrifugation. The obtained BT nanoparticles were washed with deionized water and then were dried in an oven at 60 °C for 12 h.23
Fabrication of the GO material.
Graphite oxide (GO) was synthesized by a modified Hummers method, as reported previously by our group.24
Fabrication of the rGO@BT composites.
rGO@BT hybrids were obtained by combining 40 mg of GO powder and 20 mg of the BT nanospheres in 60 mL of deionized water under ultrasonication for 1 h. The above suspension and hydrazine hydrate were transferred into a 100 mL flask and vigorously stirred at 90 °C for 2 h, followed by sonicating for 1 h. The precipitate was washed with water and ethanol sequentially several times to obtain rGO@BT composites with uniform morphology, followed by drying in an oven at 60 °C for 24 h for further characterization.
The preparation of the rGO@BT/PVDF films.
The membranes of rGO@BT/PVDF were obtained by a simple casting procedure. rGO@BT nanoparticles with different PVDF mass content were acquired as follows: the solution consisting of a certain amount of PVDF and N,N-dimethylformamide (DMF) was stirred for 30 min before the rGO@BT nanoparticles were added. After stirring for 10 min and ultrasonicating for 1 h, the suspension was transferred into glass petri dishes and dried in an oven at 70 °C for 4 h. Pieces of films were acquired for further measurement.
The samples for microwave-absorption testing were made via a simple blending and hot-molding procedure. The membranes were pressed into a cylindrical shaped compact (Φout = 7.00 mm and Φin = 3.04 mm) under 220 °C and 4 MPa.
2.3 Characterization
A series of measurements was conducted to determine the microstructure of the sample. Scanning electron microscopy (SEM, JSM-7500F) was carried out to observe the morphology and size of the sample. The crystal structure of the sample was determined by X-ray diffraction (XRD) (D/MAX-1200, Rigaku Denki Co. Ltd, Japan) with Cu Kα radiation at λ = 0.15406 nm at 40 kV and 40 mA were recorded in the range of 2θ = 10–80°. Raman spectra were recorded by exciting the sample from 500 to 2000 cm−1 on a LabRAM HR800 Laser Raman spectroscope (HORIBA Jobin Yvon Co. Ltd, France) using a 632.5 nm argon ion laser. The weight percentage of wrapping GO on the BT particles was measured using a Pyris Diamond TG analyzer (PerkinElemer Inc., USA) in air from room temperature to 750 °C at a rate of 5 °C min−1.
2.4 Evaluation of microwave absorption
Microwave absorption is evaluated by calculating the value of the reflection loss. The dependence of the microwave absorption property on the permittivity and permeability can be expressed by the following equation:25 | 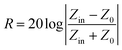 | (1) |
where Z0 is the impedance of free space and Zin is the input characteristic impedance which can be described by |  | (2) |
where εr and μr are the complex permittivity and permeability of the composite medium, respectively; f is the frequency; d is the thickness of the absorbent and c is the velocity of light in a vacuum.
2.5 The assessment of the EMI shielding property
The EM parameters of the composites were measured by the transmission/reflection coaxial line method. The values of complex permittivity and permeability were measured in the 2–18 GHz frequency range by an Agilent N5244a network analyzer for further calculation.
3 Results and discussion
3.1 Morphology and structure
The morphology of the hybrid products were examined using an SEM instrument. The BT image indicates that BT particles are spherical with an average diameter of 100 nm (Fig. 1a). According to Fig. 1b, the BT nanospheres are uniformly scattered among the rGO sheet and keep the same morphology and structure. Fig. 1c presents the FESEM image of the fracture surface of the rGO@BT/PVDF membrane. An Energy Dispersive Spectrometer (EDS) was used to investigate the dispersion of the rGO@BT particles in PVDF. From the element mapping images of Ba, O, F and Ti, we can conclude that the rGO@BT hybrids are well distributed among the PVDF membrane. The good dispersion of Ba, O, F and Ti atoms on the surface may be beneficial to the dielectric and absorption performance.
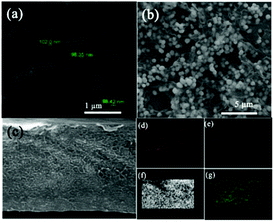 |
| Fig. 1 SEM pictures of (a) the pure BT powder and (b) the rGO@BT composites; (c) FESEM image of the fracture surface of the rGO@BT/PVDF membrane and the corresponding element mapping images of (d) Ba, (e) O, (f) F and (g) Ti. | |
Fig. 2a reveals the XRD patterns of the pure BT particles and the hybrid rGO@BT composites. The main diffraction peaks of rGO@BT are located at 2θ values of 22.3°, 31.6°, 38.9°, 45.4°, 56.2°, 65.8°, 70.4°, 75.1° and 79.5°, corresponding to the (100), (110), (111), (200), (211), (202), (212), (301) and (311) planes, respectively. These peaks match the documented standard XRD pattern of BT (JCPDS No. 05-0626). The absence of a peak at 24° indicates that there are no stacked sheets in the rGO@BT composites, that is, the BT particles disperse in rGO without discernible aggregation.26 The TGA curves provide a quantitative analysis of the wrapping GO content. It can be estimated from the curves shown in Fig. 2b that the weight ratio of rGO in the rGO@BT sample is approximately 60 wt%, which is basically identical to the calculated value, where the rGO@BT composites were obtained using 40 mg of GO and 20 mg of BT particles. The rGO@BT curve experiences two sharp mass losses. The first one began at almost 200 °C, accompanied by the absence of oxygen-containing groups. The other began at around 400 °C on account of the pyrolysis of carbon.27Fig. 2c presents the Raman spectra of the samples. For the GO powder, two peaks at 1336.4 and 1598.9 cm−1 were observed, corresponding to the documented D and G bands.28 While, compared to GO, the peaks for the rGO@BT composites appear at 1327.4 and 1599.3 cm−1. This is mainly caused by charge doping with electron-withdrawing components. The intensity of ID/IG increases from 1.15 to 1.28, indicating a decrease in the average size of the sp2 carbon domains, which could be the loss of sp2 carbon after the reduction.29,30
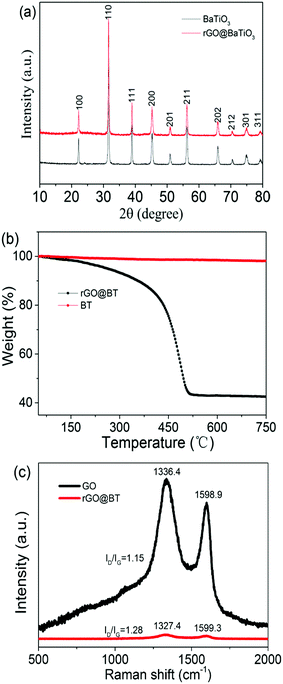 |
| Fig. 2 The (a) XRD patterns, (b) TG curves and (c) Raman spectra of the rGO@BT composites, as marked. | |
3.2 The MA performance with different content
The reflection loss versus frequency with varying amounts of filler (5, 10, 15, 20, 25 and 30 wt%) are shown in Fig. 3a–f. In our work, we calculated different thicknesses among 1–5 mm in the frequency range of 2–18 GHz. It is obvious that the composites with a loading of 10, 15, 20 and 25 wt% display excellent microwave attenuation, with a high value of reflection loss and a wide frequency bandwidth (<−10 dB). The composite with 15 wt% rGO@BT has the highest reflection coefficient of up to −45.3 dB at 5.44 GHz at a thickness of 4.5 mm with a frequency bandwidth of 4–18 GHz. At a thickness of 3.5 mm, the 15 wt% composite exhibits a maximum reflection loss of −39.0 dB in 7.76 GHz, which means almost 99.99% of the microwaves are dissipated. When the content increases to 20 wt%, the maximum reflection loss of a 2.0 mm thick sample can reach −30.0 dB. According to our results, the maximum reflection loss of the 10 wt% composite at a thickness of 3.0, 3.5, 4.0 and 4.5 mm is −22.1 dB, −32.7 dB, −33.2 dB and −27.5 dB, respectively. The peaks of reflection loss are ascribed to the influence of quarter-wavelength attenuation.31,32 Basically, a qualified impedance with air for the sample is greatly needed for excellent absorption properties, which is connected to the permittivity, permeability, frequency and thickness parameters. Barium titanate, a ceramic material, possesses high permittivity and graphene can dissipate energy until it disappears completely in the loose frame. rGO@BT mixed with wax composites was prepared for comparison. The reflection loss with a thickness range of 1–5 mm and filler amounts of 5 and 15 wt% over a frequency of 2–18 GHz is showed in Fig. S1 (ESI†). The maximum reflection loss value shifts to lower frequency with an increase in thickness and the maximum reflection loss value just reaches −8.3 dB for 15 wt% composites. The wave absorption properties have been largely improved after mixing with PVDF. This is consistent with previous results in our group.33–35
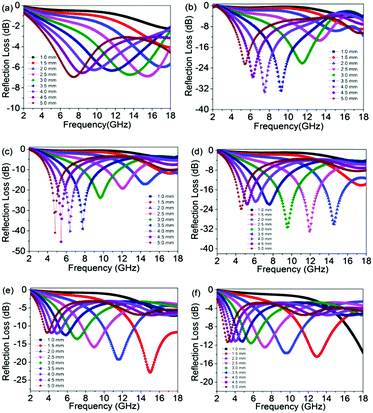 |
| Fig. 3 The reflection loss of rGO@BT/PVDF composites with a thickness range of 1–5 mm and a filler content of (a) 5, (b) 10, (c) 15, (d) 20, (e) 25 and (f) 30 wt% over the frequency range of 2–18 GHz. | |
As we all know, the electromagnetic attenuation of a sample is determined based on its experimentally complex permittivity and permeability. The permittivity of the sample was measured by a waveguide method. The frequency dependence of the dielectric properties, including the real and imaginary parts of the permittivity and dielectric loss of the composites containing varying amounts, were investigated and are shown in Fig. 4. As can be seen, the real part of the permittivity increases dramatically as the concentration increases from 5 to 30 wt%, while it decreases with frequency, especially for lower frequency values. In the frequency range of 12–18 GHz, the real part of the permittivity has little dependence on the frequency. For the 5 and 30 wt% hybrids, the values of ε′ drop from 4.4 to 3.3 and 20.9 to 11.8. The introduction of the rGO@BT hybrids dramatically raises the real part of the permittivity, to almost 4 times higher than before. Basically, the existence of polarization has a pronounced influence on the real part of the permittivity. The periodic reversal of the electric field occurs too quickly so that there is no excess diffusion in the field direction because of the increasing frequency in the field. Consequently, polarization due to charge accumulation decreases, giving rise to a decrease in the permittivity value with increasing frequency.36,37 The value of ε′ follows the Debye theory as38
| 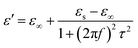 | (3) |
where
f,
τ,
εs and
ε∞ are the frequency, polarization relaxation time, static permittivity and the relative dielectric permittivity at infinite frequency, respectively. The observed increase in the real permittivity in the results is likely to increase the content of rGO and BT particles in the composites. Therefore, the higher ratios coupled with higher polarization lead to the enhancement of the real permittivity. It is curious to observe that the imaginary permittivity displays complex fluctuation with respect to the frequency. The imaginary permittivity follows the Debye theory and can be described as
| 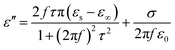 | (4) |
where
σ is the electrical conductivity. The composites show a higher carrier mobility of graphene with increasing content, which would give rise to the electron polarization.
8
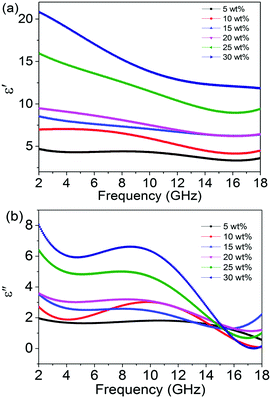 |
| Fig. 4 (a) Real and (b) imaginary parts of the relative complex permittivity. | |
Dielectric loss can be described as tan
δ = ε′′/ε′. The value of tan
δ provides an indication of the ability of the material to dissipate electromagnetic energy into heat and materials with a moderately high dielectric loss are inclined to be applied in the field of stealth technology. Fig. S2 (ESI†) shows a plot of tan
δ versus frequency of the rGO@BT/PVDF composites as a function of rGO@BT loading. There is a peak in the dielectric loss in the frequency of 7–15 GHz, indicating that the composite may show excellent microwave absorption.
The dielectric loss may arise from the relaxation process. Based on Debye relaxation, the relationship between ε′ and ε′′ can be deduced from eqn (3) and eqn (4) and is given as below:
| 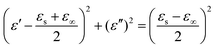 | (5) |
In which
εs and
ε∞ are the static dielectric constant and the constant at infinite frequency, respectively. Therefore, the plot of
ε′′
versus ε′ should be a single semicircle, denoted as a Cole–Cole semicircle.
39,40 Every single semicircle corresponds to one Debye relaxation process. According to Fig. S3 (ESI
†), there is more than one relaxation process observed in the samples. To the best of our knowledge, a delay in polarization along with a change in the electrical field in a dielectric medium can induce the relaxation processes.
41 The existence of interfaces between the BT particles and rGO sheets, as well as between the rGO@BT composites and PVDF matrix, would cause interfacial polarization.
3.3 EMI shielding property
The EMI shielding property is evaluated by the measured S parameters (S11 and S21). The efficiency of EMI shielding consists of the reflected power (R), absorbed power (A) and transmitted power (T), which can be calculated using the relationships as below:The plots of the calculated values of R, T and A versus frequency are shown in Fig. S4 (ESI†). The total EMI shielding effectiveness SEtotal, which can be defined as the logarithmic ratio between the incident power PI and the transmitted power PT, can be calculated by the following equations: | SEtotal = −10 log(PT/PI) = SER + SEA + SEM | (9) |
| SEA = −10 log[T/(1 − R)] | (10) |
| SER = −10 log(1 − R) | (11) |
The plots of SEtotal, SEA and SER are presented in Fig. 5 to give a detailed analysis of the shielding mechanism. It can be seen that the efficiency of the SEtotal increases with increasing rGO@BT concentration. The sample with a filler of 30 wt% demonstrates a maximum SEtotal of up to 9.8 dB, which is 2 times higher than that of the 5 wt% sample.
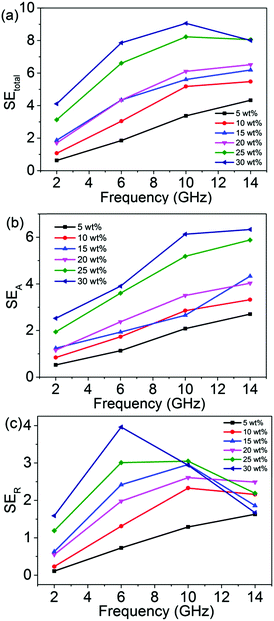 |
| Fig. 5 The frequency dependence of (a) SEtotal, (b) SEA and (c) SER of the samples. | |
The absorption effectiveness is related to skin depth and thickness as:42
| 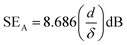 | (12) |
where
d is the shielding thickness and
δ is the skin depth, which can be defined as
δ = (π
fμσ)
−1/2 when
σ ≫ 2π
fε
0,
μ =
μ0μr (
μ0 = 4π × 10
−7 H m
−1,
μr = 1 in this case).
On the basis of the EMI shielding mechanism, the electrical conductivity (Fig. 6) of the sample can be evaluated in accordance with the relation as follows43
where
ε0 is the permittivity of free space and is 8.854 × 10
−12 F m
−1 and
ε′′ is the imaginary part of the permittivity. The corresponding calculated values of skin depth are shown in
Fig. 7. Obviously, the skin depth of 30 wt% rGO@BT/PVDF is dramatically lower than that of the 5 wt% sample with the same thickness, indicating superior absorption effectiveness values, which is consistent with the experimental results shown in
Fig. 5b.
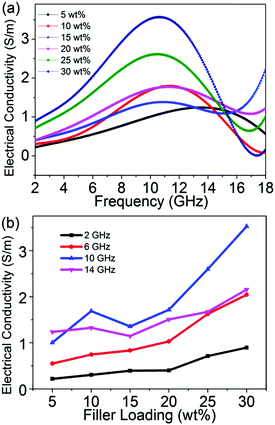 |
| Fig. 6 Calculated electrical conductivity versus frequency (a) and the filler percentage (b) of the composites over the alternating electric field. | |
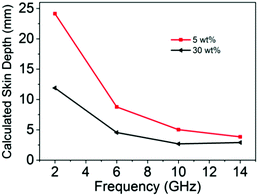 |
| Fig. 7 Calculated skin depth curves for rGO@BT/PVDF with 5 and 30 wt% content. | |
The reflection effectiveness is associated with impedance and can be expressed as:44
| 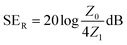 | (14) |
where
Z0 = (
μ0/
ε0)
1/2 and
Z1 is the impedance of the sample. Apparently, lower impedance is in favor of reflection. In other words, either increasing the conductivity or decreasing the permeability can promote the EMI shielding performance.
Plots showing the calculated SEA and SER values as a function of frequency are shown in Fig. 8. Compared to the measurement results of the SEA values, the two curves display a similar trend. Furthermore, according to Fig. 9a, the relationship of SEA (dB) as a function of σ1/2 for 5 wt% rGO@BT/PVDF shows linear dependence, confirming the validity of eqn (12). Similarly, the plot of SER (dB) as a function of log(σ) shows linear dependence as well demonstrating the validity of eqn (14) and (15). However, the plot of the calculated SER shows higher values but has a different trend to the experimental results. The mechanism is not clear yet. Carbonized phenolic foam C-PFCs with MWCNT amounts of 0.5, 1, 2, 3, 4 and 5 wt% at a frequency of 8 GHz exhibit the same phenomenon with respect to SER.45 Li et al. also reported that the SER as a function of frequency of PFC2Fs decreases with Fe3O4 loading.45
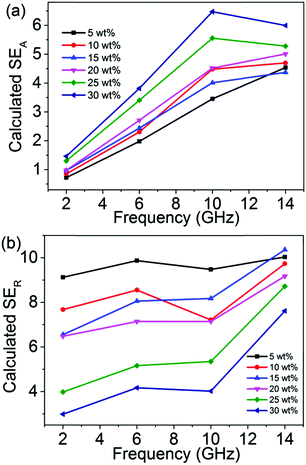 |
| Fig. 8 Plots of (a) calculated SEA and (b) SERversus frequency. | |
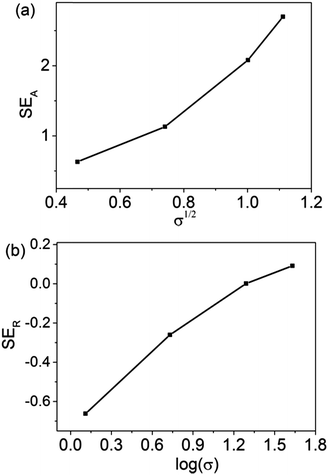 |
| Fig. 9 Plots of experimental measurements of (a) SEAversus σ1/2 and (b) SERversus log(σ) for 5 wt% rGO@BT/PVDF. | |
Conclusions
In conclusion, barium titanate (BT) coated rGO hybrids were successfully prepared by a simple method and the rGO@BT/PVDF composites with filler amounts of 10, 15, 20 and 25 wt% exhibit a broad frequency band (<−10 dB) and the highest reflection coefficient of up to −33.2, −45.3, −32.9 and −22.8 dB, respectively, in the frequency range of 2–18 GHz. The electromagnetic interference (EMI) shielding effectiveness was measured and the theoretical calculations of SEA and SER were displayed for comparison. The electrical conductivity and skin depth were showed as well for further investigation. Hence, the composites of rGO@BT/PVDF are expected to be used as an effective lightweight microwave absorbing material.
Acknowledgements
This project is supported by the National Science Foundation of China (No. 51472012). A special thanks to the engineer Ye for helping with the measurements.
Notes and references
- B. Wen, M. S. Cao, Z. L. Hou, W. L. Song, L. Zhang, M. M. Lu, H. B. Jin, X. Y. Fang, W. Z. Wang and J. Yuan, Carbon, 2013, 65, 124 CrossRef CAS.
- N. Li, Y. Huang, F. Du, X. B. He, X. Lin, H. J. Gao, Y. F. Ma, F. F. Li, Y. S. Chen and P. C. Eklund, Nano Lett., 2006, 6, 1141 CrossRef CAS PubMed.
- X. Liu, Z. Zhang and Y. Wu, Composites, Part B, 2011, 42, 326 CrossRef.
- J. H. Oh, K. S. Oh, C. G. Kim and C. S. Hong, Composites, Part B, 2004, 35, 49 CrossRef.
- M. H. Al-Saleh, W. H. Saadeh and U. Sundararaj, Carbon, 2013, 60, 146 CrossRef CAS.
- G. Li, T. Xie, S. Yang, J. Jin and J. Jiang, J. Phys. Chem. C, 2012, 116, 9196 CAS.
- P. B. Liu, Y. Huang and X. Sun, ACS Appl. Mater. Interfaces, 2013, 5, 12355 CAS.
- K. S. Novoselov, A. K. Geim, S. V. Morozov, D. Jiang, Y. Zhang, S. V. Dubonos, I. V. Grigorieva and A. A. Firsov, Science, 2004, 306, 666 CrossRef CAS PubMed.
- X. Bai, Y. Zhai and Y. Zhang, J. Phys. Chem. C, 2011, 115, 11673 CAS.
- A. P. Guo, X. J. Zhang, S. W. Wang, J. Q. Zhu, L. Yang and G. S. Wang, ChemPlusChem, 2016, 81, 1305 CrossRef CAS.
- X. J. Zhang, G. S. Wang, W. Q. Cao, Y. Z. Wei, M. S. Cao and L. Guo, RSC Adv., 2014, 4, 19594 RSC.
- Y. F. Pan, G. S. Wang and Y. H. Yue, RSC Adv., 2015, 5, 71718 RSC.
- D. Chen, G. S. Wang, S. He, J. Liu, L. Guo and M. S. Cao, J. Mater. Chem. A, 2013, 1, 5996 CAS.
- G. S. Wang, Y. Wu, Y. Z. Wei, X. J. Zhang, Y. Li, L. D. Li, B. Wen, P. G. Yin, L. Guo and M. S. Cao, ChemPlusChem, 2014, 79, 375 CrossRef CAS.
- V. K. Singh, A. Shukla, M. K. Patra, L. Saini, R. K. Jani, S. R. Vadera and N. Kumar, Carbon, 2012, 50, 2202 CrossRef CAS.
- Y. Ding, Q. Liao, S. Liu, H. Guo, Y. Sun, G. Zhang and Y. Zhang, Sci. Rep., 2016, 6, 32381 CrossRef CAS PubMed.
- Y. Ding, L. Zhang, Q. Liao, G. Zhang, S. Liu and Y. Zhang, Nano Res., 2016, 9, 2018–2025 CrossRef CAS.
- Y. Ding, Z. Zhang, B. Luo, Q. Liao, S. Liu, Y. Liu and Y. Zhang, Nano Res., 2017, 10, 980–990 CrossRef CAS.
- L. Zhang, X. Zhang, G. Zhang, Z. Zhang, S. Liu, P. Li, Q. Liao, Y. Zhao and Y. Zhang, RSC Adv., 2015, 5, 10197–10203 RSC.
- X. Chen, G. Wang, Y. Duan and S. Liu, J. Alloys Compd., 2008, 453, 433 CrossRef CAS.
- Y. F. Zhu, L. Zhang, T. Natsuki, Y. Q. Fu and Q. Q. Ni, ACS Appl. Mater. Interfaces, 2012, 4, 2101 CAS.
- Z. M. Dang, Y. H. Lin and C. W. Nan, Adv. Mater., 2003, 15, 1625 CrossRef CAS.
- T. Zhou, J. W. Zha, R. Y. Cui, B. H. Fan, J. K. Yuan and Z. M. Dang, ACS Appl. Mater. Interfaces, 2011, 3, 2184 CAS.
- D. Chen, L. Li and L. Guo, Nanotechnology, 2011, 22, 325601 CrossRef PubMed.
- Z. Liu, G. Bai, Y. Huang, F. Li, Y. Ma, T. Guo, X. He, X. Lin, H. Gao and Y. Chen, J. Phys. Chem. C, 2007, 111, 13696 CAS.
- D. Wang, Y. Bao, J. W. Zha, J. Zhao, Z. M. Dang and G. H. Hu, ACS Appl. Mater. Interfaces, 2012, 4, 6273 CAS.
- G. Wang, J. Yang, J. Park, X. Gou, B. Wang, H. Liu and J. Yao, J. Phys. Chem. C, 2008, 112, 8192 CAS.
- B. Shen, Y. Li, D. Yi, W. Zhai, X. Wei and W. Zheng, Carbon, 2016, 102, 154 CrossRef CAS.
- S. Stankovich, D. A. Dikin, R. D. Piner, K. A. Kohlhaas, A. Kleinhammes, Y. Jia, Y. Wu, S. T. Nguyen and R. S. Ruoff, Carbon, 2007, 45, 1558 CrossRef CAS.
- Y. Zhou, Q. Bao, L. A. L. Tang, Y. Zhong and K. P. Loh, Chem. Mater., 2009, 21, 2950 CrossRef CAS.
- M. S. Cao, R. R. Qin, C. J. Qiu and J. Zhu, Mater. Des., 2003, 24, 391 CrossRef.
- W. l. Song, M. s. Cao, Z. l. Hou, J. Yuan and X. y. Fang, Scr. Mater., 2009, 61, 201 CrossRef CAS.
- X. J. Zhang, G. S. Wang, Y. Z. Wei, L. Guo and M. S. Cao, J. Mater. Chem. A, 2013, 1, 12115 CAS.
- X. J. Zhang, G. S. Wang, W. Q. Cao, Y. Z. Wei, J. F. Liang, L. Guo and M. S. Cao, ACS Appl. Mater. Interfaces, 2014, 6, 7471 CAS.
- X. J. Zhang, G. C. Lv, G. S. Wang, T. Y. Bai, J. K. Qu, X. F. Liu and P. G. Yin, RSC Adv., 2015, 5, 55468 RSC.
- V. Raja, A. K. Sharma and V. Rao, Mater. Lett., 2004, 58, 3242 CrossRef CAS.
- A. Gupta and V. Choudhary, Compos. Sci. Technol., 2011, 71, 1563 CrossRef CAS.
- M. S. Cao, W. L. Song, Z. L. Hou, B. Wen and J. Yuan, Carbon, 2010, 48, 788 CrossRef CAS.
- P. Xu, X. Han, C. Wang, D. Zhou, Z. Lv, A. Wen, X. Wang and B. Zhang, J. Phys. Chem. B, 2008, 112, 10443 CrossRef CAS PubMed.
- X. L. Dong, X. F. Zhang, H. Huang and F. Zuo, Appl. Phys. Lett., 2008, 92, 013127 CrossRef.
- L. Kong, X. Yin, X. Yuan, Y. Zhang, X. Liu, L. Cheng and L. Zhang, Carbon, 2014, 73, 185 CrossRef CAS.
- M. H. Al-Saleh and U. Sundararaj, Carbon, 2009, 47, 1738 CrossRef CAS.
- J. G. Park, J. Louis, Q. Cheng, J. Bao, J. Smithyman, R. Liang, B. Wang, C. Zhang, J. S. Brooks, L. Kramer, P. Fanchasis and D. Dorough, Nanotechnology, 2009, 20, 415702 CrossRef PubMed.
- W. L. Song, M. S. Cao, L. Z. Fan, M. M. Lu, Y. Li, C. Y. Wang and H. F. Ju, Carbon, 2014, 77, 130 CrossRef CAS.
- Q. Li, L. Chen, J. Ding, J. Zhang, X. Li, K. Zheng, X. Zhang and X. Tian, Carbon, 2016, 104, 90 CrossRef CAS.
Footnote |
† Electronic supplementary information (ESI) available. See DOI: 10.1039/c7qm00204a |
|
This journal is © the Partner Organisations 2017 |
Click here to see how this site uses Cookies. View our privacy policy here.