DOI:
10.1039/C6RA24686A
(Paper)
RSC Adv., 2017,
7, 7758-7762
Tungsten doped VO2/microgels hybrid thermochromic material and its smart window application
Received
3rd October 2016
, Accepted 10th November 2016
First published on 23rd January 2017
Abstract
Thermal sensitive microgels were synthesized directly from hydroxypropyl cellulose (HPC) and acrylic acid (AA) in pure water. The first cloud point temperature, near 45 °C, of the inorganic/organic hybrid thermochromic material was obtained by dispersing W doped VO2 in HPCA microgels and the composite microgel is highly transparent at room temperature with Tlum = 80% when the thickness is 25 μm. The excellent solar modulating ability (ΔTsol) of 36%, high averaged Tlum of 56% and relatively high cloud point temperature of 50 °C make it suitable for smart windows applications compared with pure VO2 based materials and previously reported thermochromic hybrids.
Introduction
Due to the zero extra energy requirement and large solar spectrum wavelength blocking effect, thermochromic materials have become the cost effective smart window material. For an ideal thermochromic smart-window, a large solar modulation, ΔTsol, a suitable critical temperature (τc) and a relatively high luminous transmission (Tlum) are required.1 Vanadium dioxide (VO2) is the most studied thermochromic material in the energy-saving smart window applications. The phase transition critical temperature is around 68 °C and the VO2 can block infrared (IR) transmission (TIR) at a temperature above τc and allow IR transmission below τc.2,3 To attain good thermochromic properties, much effort, such as doping (such as Mg2+/W, and rare earth doping, such as Tb, La and Eu3+),3–7 nanoporous reconstruction,8 antireflective coating multilayers,9,10 nanoparticle-based composites,11–15 and gridded16 and biomimetic nanostructuring17,18 have been investigated. However, the intrinsic physical properties of VO2 of a high τc of 68 °C restricted it to be applied directly to smart windows applications. Doping by tungsten can significantly reduce τc of VO2 nanoparticles.3
Hydroxypropylcellulose (HPC) is a water-soluble cellulose derivative,19 which has being developed into materials such as for environmental friendly and biocompatible requirements,20 and biodegradable, non-toxic and solid polymers.21 As a renewable resource, HPC also has a number of traditional applications including in the biological, medical and pharmaceutical fields.22–28
In previous work, a thermochromic material based on HPC has been reported.29 In this paper, a facile way of preparing a HPC derivative (HPCA microgel) based thermochromic material is reported. By hybridizing with W doped-VO2 nanoparticles, HPC offers both a large visible and IR modulating ability together with a relative high Tlum. This is the first near room temperature hybrid thermochromic material.
Experimental
Materials
The chemicals used in this study were HPC (MW 100
000, 99% purification, Sigma-Aldrich), W-VO2 (Jing Cheng PTE LTD), acrylic acid (AA) (Sigma-Aldrich), Disperbyk 180 (Sigma-Aldrich) and a multipurpose sealant (Selleys All Clear). AA was dried by MgSO4 and then vacuum distilled before use. N,N′-Methylene bisacrylamide (MBA) (Fluka) was recrystallized from methanol. Cerium(IV) ammonium nitrate (CAN) (Sigma-Aldrich) was recrystallized from dilute nitric acid containing an appropriate amount of ammonium nitrate. Deionized water (18.2 MΩ) was used throughout the experiments. All chemicals were used as received, without any further purification.
Synthesis of HPCA microgel
An aqueous solution of 50 mg CAN in 2.5 mL of 0.1 mol L−1 nitric acid and 0.7 mL of AA were successively added to 100 mL of 2% aqueous solution of HPC under gentle stirring and nitrogen bubbling. The reaction proceeded at 25 °C for 1 h, then 15 mL AA and 5 g MBA were added, stirred for a further 4 h with pH kept at 1–2, followed by adjusting the pH to 7.0 with 3 mol L−1 NaOH. Excess precursors and side-products were removed over 7 days of dialysis using a membrane bag with a molecular weight cutoff of 14
000. The resultant microgels were obtained by freeze-drying the remaining solvent in the membrane bag. The synthesis procedure is illustrated in Scheme 1.
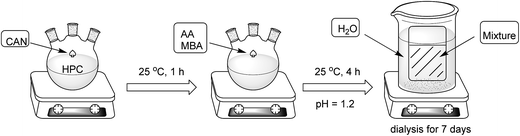 |
| Scheme 1 Scheme of synthesizing HPCA microgel. | |
Preparation of HPCA microgel films
A 0.03 g HPCA sample was mixed in 2 mL deionized water to form the HPCA microgel by ultrasonics for one hour to ensure it thoroughly dissolved and was left to stand for one day to form the microgel.
Preparation of W-doped VO2 film
The W-doped VO2/DPM solution was prepared by dilution with a DPM solution with a volume ratio of 1
:
10. Samples with a 25 μm thickness film were made, the same as W-doped VO2/HPCA microgel films.
Preparation of W-doped VO2/HPCA microgel composite films
The dispersion of W-doped VO2 (Jing Cheng PTE LTD) into hydrogel has been previously reported.30,31 0.05 g W-doped VO2 nanoparticles was added into the 0.5 mL DPM solution; after 30 minutes of ultrasonic dispersion, 5 μL BYK 180 was added into the solution, then dispersed in ultrasonics for 30 minutes more. The W-VO2 solution was mixed with the HPCA solution with a volume ratio of 1
:
10. Samples with 12.5 μm, 25 μm and 50 μm films were made and the sealant was applied at the edges to prevent mass exchange with the outside environment.
Characterization
SEM. The inner structures of the HPCA were determined by a field emission scanning electron microscope (FESEM, SUPRA 55, ZEISS) with an operating voltage of 5.00 kV. 2 mL HPCA solution was injected into 2 plastic vials separately and equally, one vial was heated to 80 °C for 5 minutes and the two vials were immersed into nitrogen liquid immediately. The 2 samples were then freeze-dried and examined by SEM.
UV-VIS-NIR. Optical properties were examined by UV-VIS-NIR (Cary 5000, Agilent, USA) spectra, with a working wavelength range of 250–2500 nm at 10 nm s−1 incidence. All the tests were run at 20 °C and 60 °C, respectively, while the temperature was controlled by a heating stage (PE120, Linkam, UK). The calculation of the integral luminous transmittance, Tlum (380–780 nm), infrared transmittance, TIR (780–2500 nm) and solar transmittance, Tsol (250–2500 nm) and ΔTlum/IR/sol can be found elsewhere.32The integral luminous transmittance Tlum (380–780 nm), IR transmittance TIR (780–2500 nm), and solar transmittance Tsol (250–2500 nm) were calculated with eqn (1):
|
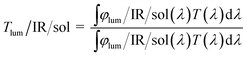 | (1) |
where
T(
λ) denotes spectral transmittance,
φlum(
λ) is the standard luminous efficiency function of photopic vision
33 in the wavelength range of 380–780 nm, and
φIR(
λ) and
φsol(
λ) are the IR/solar irradiance spectrum distribution for air mass 1.5 (corresponding to the sun standing 37° above the horizon with 1.5 atmosphere thickness and the presence of a solar zenith angle of 48.2).
34 Δ
Tlum/IR/sol is obtained by Δ
Tlum/IR/sol =
Tlum/IR/sol,20 °C −
Tlum/IR/sol,60 °C.
Results and discussion
As shown in Fig. 1a, the transmission in the visible range of pure HPCA microgel dramatically decreases when the temperature changes from 20 °C to 65 °C with a negligible IR change. The Tlum of the sample reduced from almost 85% to less than 40%, which reflected that the solution changes from transparent at 20 °C to translucent at 50 °C (see the glass film in Fig. 1b). It can be recovered to transparency within 1 min after removing the heater. The reduction in transmittance at around 1430 and 1930 nm for both high and low temperatures is due to the absorption of water at these two wavelengths.
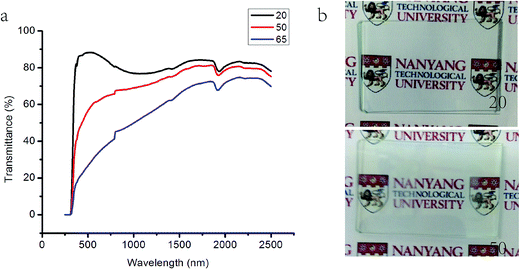 |
| Fig. 1 (a) Optical transmittance spectra of the 25 μm HPCA microgel sample with temperatures from 20 °C to 65 °C. (b) Pictures of 20 °C and 50 °C HPCA microgels. | |
The HPC hydrogels at both 20 and 80 °C were freeze-dried and the remaining solid was examined by SEM. Fig. 2 shows the difference in the structure of HPCA at 20 and 80 °C; the polymer fibers at room temperature are more elongated and uniform (Fig. 2a); however, at 80 °C, the length of the polymer fibers is reduced dramatically to around 4–5 μm, accompanied by a largely contracted pore size of less than 8 μm (Fig. 2b). The water driven out from the HPCA microgel fiber above the cloud point temperature causes the shrinkage; the much more complicated polymeric web structure acts as a scattering center to block the visible light and results in an opaque status above the cloud point temperature.
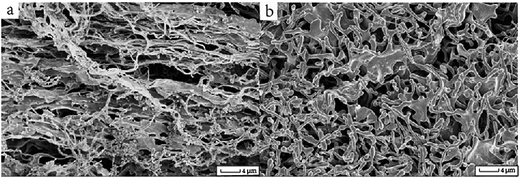 |
| Fig. 2 SEM image of the freeze-dried HPCA microgel at (a) 20 °C and (b) 80 °C. | |
The transmittance at a fixed wavelength of 580 nm in the temperature range 20 to 65 °C was recorded in a heating and cooling cycle and plotted as %T versus T/°C hysteresis loops for pure HPCA and W-VO2 HPCA composite microgels, with a fixed wavelength for W-VO2 of 2500 nm (Fig. 3). The transmission electron microscope (TEM) image of the particles is shown in the inset, indicating that the particle size is in the order of 50 nm. The cloud point temperature of pure HPCA is about 52.5 °C and τc for the W-VO2 is 40 °C on average for the cooling and heating cycle with a Tlum decrease from 70% to 45% when the temperature rose from 25 °C to 65 °C. It is of great interest that W-VO2 can reduce the cloud point temperature of HPCA to 50 °C, which indicates that W doped VO2 could influence the phase change of the HPCA microgel.
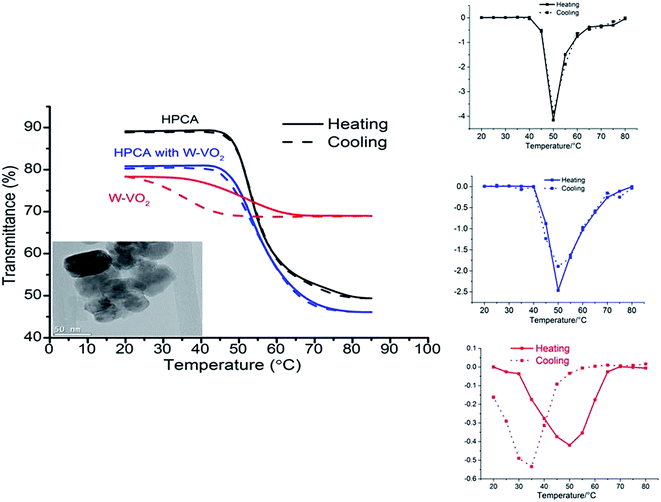 |
| Fig. 3 Hysteresis loops for the temperature-dependent transmittance of the HPCA and W-VO2 HPCA composite microgels of 12.5 μm thickness at a wavelength of 580 nm and W-VO2 nanoparticles of 12.5 μm thickness at a wavelength of 2500 nm with the corresponding derivative on the right. Inset: TEM picture of W-VO2 nanoparticles. | |
The thickness influence on ΔTsol, ΔTlum, and ΔTIR was investigated in this work. The optical transmittance spectra of 12.5, 25 and 50 μm thickness of the HPCA microgel samples are shown in Fig. 4a; and the Tlum(20,60 °C), ΔTsol, ΔTlum, and ΔTIR are shown in Fig. 4b. The Tlum of 20 °C of the HPCA film slightly decreases from 87.5% to 85.1%; however, the Tlum of 60 °C of HPCA film dramatically drops from 49.2% to 22.9%. The ΔTsol, ΔTlum, and ΔTIR increase slightly from 12.5 μm to 25 μm and increase dramatically from 25 μm to 50 μm. Although the 50 μm thick HPCA microgel exhibits a much higher ΔTsol value of 44%, its low Tlum(60 °C) of less than 25% is not favored for an ideal smart window.
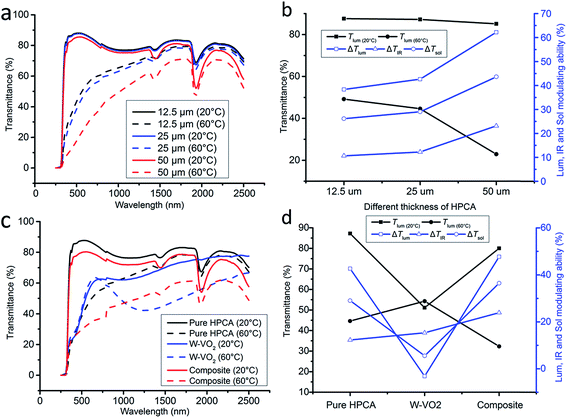 |
| Fig. 4 (a) Optical transmittance spectra of 12.5, 25 and 50 μm thick HPCA microgel samples; (b) thickness influence on ΔTsol, ΔTlum, ΔTIR and Tlum (20 and 60 °C) of HPCA samples; (c) optical transmittance spectra of 25 μm HPCA, W-VO2, and W-VO2 with HPCA microgel samples. (d) ΔTsol, ΔTlum, ΔTIR and Tlum (20 and 60 °C) of 25 μm HPCA, W-VO2, and W-VO2 with HPCA microgel samples. | |
According to the above discussion, the pure HPCA microgel of 25 μm thickness was the most suitable for smart windows application compared with 12.5 and 50 μm. The optical transmittance spectra of 25 μm thickness samples of HPCA, W-VO2, and W-VO2 HPCA composite microgel (250–2500 nm) profiles at 20 °C and 60 °C are shown in Fig. 4c. The pure HPCA film has the highest solar transmittance for a temperature of 20 °C with a Tlum of 87%. The ΔTlum of the pure HPCA microgel is 43%, which indicates that when the temperature rises up to 60 °C, the transmittance of the sample turns opaque. For pure W-VO2, the large transmission contrast remains in the IR region, the ΔTIR is up to 15% compared to a ΔTsol of 5%, so it serves as a good smart IR blocking additive in the HPCA microgel. Although adding W-VO2 lowers the transmittance at both 20 °C and 60 °C, as adding W-VO2 into HPCA thin film forms a hybrid, the ΔTIR of the hybrid increased 2 times from 12 to 24% compared with that of the pure HPCA film (Fig. 4d), while ΔTlum also increased from 42.6 to 47.5%, which led to a 25.5% increase of ΔTsol. Thus, the new hybrid has a larger modulating ability in both the visible and IR ranges compared with pure HPCA and W-VO2 film; at the same time, a dramatically high ΔTsol ∼ 36.4% was achieved with just 7.2% of visible transmittance sacrificed at room temperature. This is the first device which can effectively block both visible and IR light with a suitable phase change temperature.
Conclusion
A temperature-responsive HPCA microgel has been synthesized and W-VO2 nanoparticles were hybridized to this microgel. The 25 μm thickness composite microgel could be totally transparent at room temperature, with a high Tlum of 80%, and turned translucent at 60 °C, with an acceptable Tlum of 33%. The luminous contrast contributed from HPCA combined with the IR transmission contrast due to the W-VO2 give a high ΔTsol of 36%. The SEM of the room temperature and high temperature samples indicated that the polymer fiber became more diminished and the pores became smaller, and this polymeric web structure acts as a scattering center to block the visible light and result in an opaque status at high temperature.
Acknowledgements
This research is supported by the Singapore Minster of Education (MOE) Academic Research Fund Tier 1 RG101/13 and NRF2015NRF-POC002-019 and the fund of Wuhan Textile University (017/165006).
References
- S.-Y. Li, G. A. Niklasson and C. G. Granqvist, J. Appl. Phys., 2010, 108, 063525 CrossRef.
- Z. Zhang, Y. Gao, Z. Chen, J. Du, C. Cao, L. Kang and H. Luo, Langmuir, 2010, 26, 10738–10744 CrossRef CAS PubMed.
- N. Wang, M. Duchamp, R. E. Dunin-Borkowski, S. Liu, X. Zeng, X. Cao and Y. Long, Langmuir, 2016, 32, 759–764 CrossRef CAS PubMed.
- N. Wang, S. Y. Liu, X. T. Zeng, S. Magdassi and Y. Long, J. Mater. Chem. C, 2015, 3, 6771–6777 RSC.
- X. Cao, N. Wang, S. Magdassi, D. Mandler and Y. Long, Sci. Adv. Mater., 2014, 6, 558–561 CrossRef CAS.
- N. Mlyuka, G. Niklasson and C.-G. Granqvist, Appl. Phys. Lett., 2009, 95, 171909 CrossRef.
- N. Wang, M. Duchamp, C. Xue, R. E. Dunin-Borkowski, G. Liu and Y. Long, Adv. Mater. Interfaces, 2016, 3, 1600164 CrossRef.
- L. Kang, Y. Gao, H. Luo, Z. Chen, J. Du and Z. Zhang, ACS Appl. Mater. Interfaces, 2011, 3, 135–138 CAS.
- C. Liu, N. Wang and Y. Long, Appl. Surf. Sci., 2013, 283, 222–226 CrossRef CAS.
- Z. Chen, Y. Gao, L. Kang, J. Du, Z. Zhang, H. Luo, H. Miao and G. Tan, Sol. Energy Mater. Sol. Cells, 2011, 95, 2677–2684 CrossRef CAS.
- P. Liu, L. A. Liu, K. L. Jiang and S. S. Fan, Small, 2011, 7, 732–736 CrossRef CAS PubMed.
- Z. Chen, C. Cao, S. Chen, H. Luo and Y. Gao, J. Mater. Chem. A, 2014, 2, 11874–11884 CAS.
- Z. Chen, Y. Gao, L. Kang, C. Cao, S. Chen and H. Luo, J. Mater. Chem. A, 2014, 2, 2718–2727 CAS.
- Y. F. Gao, S. B. Wang, L. T. Kang, Z. Chen, J. Du, X. L. Liu, H. J. Luo and M. Kanehira, Energy Environ. Sci., 2012, 5, 8234–8237 CAS.
- C. Liu, X. Cao, A. Kamyshny, J. Y. Law, S. Magdassi and Y. Long, J. Colloid Interface Sci., 2014, 427, 49–53 CrossRef CAS PubMed.
- C. Liu, I. Balin, S. Magdassi, I. Abdulhalim and Y. Long, Opt. Express, 2015, 23, A124–A132 CrossRef PubMed.
- X. Qian, N. Wang, Y. Li, J. Zhang, Z. Xu and Y. Long, Langmuir, 2014, 30, 10766–10771 CrossRef CAS PubMed.
- A. Taylor, I. Parkin, N. Noor, C. Tummeltshammer, M. S. Brown and I. Papakonstantinou, Opt. Express, 2013, 21, A750–A764 CrossRef PubMed.
- S. Fortin and G. Charlet, Macromolecules, 1989, 22, 2286–2292 CrossRef CAS.
- D. Klemm, B. Heublein, H.-P. Fink and A. Bohn, Angew. Chem., Int. Ed., 2005, 44, 3358–3393 CrossRef CAS PubMed.
- Y. Nishio, Cellul. Polym., Blends Compos., 1994, 5 Search PubMed.
- D. Richardson, E. J. Lindley, C. Bartlett and E. J. Will, Am. J. Kidney Dis., 2003, 42, 551–560 CrossRef CAS PubMed.
- R. a. Rodríguez, C. Alvarez-Lorenzo and A. Concheiro, J. Controlled Release, 2003, 86, 253–265 CrossRef.
- J. Siepmann, K. Podual, M. Sriwongjanya, N. Peppas and R. Bodmeier, J. Pharm. Sci., 1999, 88, 65–72 CrossRef CAS PubMed.
- Y. Suzuki and Y. Makino, J. Controlled Release, 1999, 62, 101–107 CrossRef CAS PubMed.
- M. F. Francis, M. Piredda and F. M. Winnik, J. Controlled Release, 2003, 93, 59–68 CrossRef CAS PubMed.
- C. R. Park and D. L. Munday, Int. J. Pharm., 2002, 237, 215–226 CrossRef CAS PubMed.
- J. Siepmann and N. Peppas, Adv. Drug Delivery Rev., 2012, 64, 163–174 CrossRef.
- Y.-S. Yang, Y. Zhou, F. B. Y. Chiang and Y. Long, RSC Adv., 2016, 6, 61449–61453 RSC.
- C. Liu, X. Cao, A. Kamyshny, J. Law, S. Magdassi and Y. Long, J. Colloid Interface Sci., 2014, 427, 49–53 CrossRef CAS PubMed.
- Y. Zhou, Y. Cai, X. Hu and Y. Long, J. Mater. Chem. A, 2015, 3, 1121–1126 CAS.
- Y. Zhou, Y. Cai, X. Hu and Y. Long, J. Mater. Chem. A, 2014, 2, 13550–13555 CAS.
- G. Wyszecki and W. S. Stiles, Color Science: Concepts and Methods, Quantitative Data and Formulae, Wiley, New York, 2000 Search PubMed.
- ASTM G173 Standard Tables of Reference, Solar Spectral Irradiances: Direct Normal and Hemispherical a 37° Tilted Surface, Annual Book of ASTM Standards, American Society for Testing and Materials, Philadelphia, PA, USA, 2012, vol. 14.04, http://rredc.nrel.gov/solar/spectra.am1.5 Search PubMed.
Footnote |
† The authors equally contributed to this paper. |
|
This journal is © The Royal Society of Chemistry 2017 |
Click here to see how this site uses Cookies. View our privacy policy here.