DOI:
10.1039/C6RA28051J
(Paper)
RSC Adv., 2017,
7, 10791-10797
Amidoximated poly(vinyl imidazole)-functionalized molybdenum disulfide sheets for efficient sorption of a uranyl tricarbonate complex from aqueous solutions†
Received
11th December 2016
, Accepted 30th January 2017
First published on 9th February 2017
Abstract
It is of strategic importance to capture uranium(VI) from aqueous solutions from the points of environment and energy. A new method is reported herein for efficient uranium(VI) capture from aqueous solutions by amidoximated poly(vinyl imidazole)-functionalized MoS2 sheets. Specifically, the sorbent is prepared by grafting amidoximated poly(vinyl imidazole) onto MoS2-sheets through covalent bonds. The sorption follows pseudo-second-order kinetics and the equilibrium can be reached within 30 s. There is a large sorption capacity of 348.4 mg g−1 at pH 8.0 and 298.15 K. The sorbent shows good selectivity towards uranium(VI) over the coexisting ions in comparison with bare MoS2-sheets. In addition, the sorbent exhibits remarkable salt-resistant stability and can be regenerated efficiently after five cycles with high cycle efficiency. To the best of our knowledge, this is the first report on MoS2 sheets for uranium(VI) sorption with high efficiency from aqueous solution.
Introduction
Nowadays there are 436 nuclear power units with a capacity of about 370 million kilowatts in the world. Uranium is one of the primary fuel sources in the generation of nuclear energy. As estimated by the International Atomic Energy Agency, the total identified uranium resources on land can only last for about a century.1 The quantity of uranium in the oceans (i.e. ∼4.5 billion tons) is 1000 times higher than that in terrestrial ores.2,3 If uranium in seawater can be economically extracted, it will be enough for sustainable utilization of nuclear energy over the next few centuries. Therefore, it is a critical subject to efficiently capture uranium from aqueous solution including seawater.4,5
Over the past years, many researchers have studied the recovery of uranium from aqueous solutions, such as extraction,3 ion exchange,6 co-precipitation,7 flotation,8 membrane filtration,9 and sorption.3,10 Among them, sorption is widely utilized for uranium separation due to easy operation and low operating cost. Many sorbents of various matrix have been investigated, such as mesoporous carbon,11,12 chelating polymers,13,14 graphene oxides,15–17 metal sulfides,18,19 engineered proteins,20 and metal–organic frameworks.21 Although considerable achievements have been made, it is still a challenge to effectively extract uranium from aqueous solutions due to the complexity of environment, such as low concentration, a large number of coexisting ions, and high salinity.2,22 Therefore, it is still important to develop new materials for uranium capture from aqueous solutions.
Layered MoS2 is one kind of graphene-like two-dimensional materials with a structure of “S–Mo–S” sandwich. Ascribed to excellent mechanical properties and chemical stability,23–26 MoS2 has gained significant interest for several applications: electronic transistors,24,27 lubrication,26 catalysis,25 energy storage,28 sensors,29 and sorption.30,31 For instance, Chao et al.30 prepared graphene-like layered MoS2 by hydrothermal method for efficiently eliminating doxycycline antibiotic from aqueous solution; and Massey et al.31 reported hierarchical microspheres of MoS2 nanosheets for fast and efficient removal of dyes from the wastewater. Obviously, MoS2-sheets may be promising matrix materials for efficient uranium sorption from aqueous solution. However, the application of MoS2-sheets for metal sorption, especially for radionuclide treatment, has not been explored until now.
Uranyl tricarbonate complex [UO2(CO3)3]4− is the most abundant species of uranium(VI) in aqueous solutions with high [CO32−] and pH.32 Zeng et al.33 recently grafted cationic poly(amidoxime) onto polypropylene nonwoven fabric for extraction of [UO2(CO3)3]4− from aqueous solution. We noticed that positively charged sorbents can attract the negative uranium(VI) complex while repelling the other cations, subsequently leading to a higher sorption selectivity and faster sorption rate.33,34 Inspired by this finding, we herein synthesized amidoximated poly(vinyl imidazole)-functionalized MoS2 sheets (MoS2-PVIAO) for uranium(VI) capture from aqueous solutions (Scheme 1).
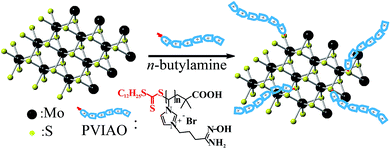 |
| Scheme 1 The schematic for synthesis of PVIAO-modified MoS2-sheets. | |
Specifically, the sorbent was prepared by one-pot reaction of amidoximated poly(vinyl imidazole) (PVIAO) and MoS2-sheets in the presence of n-butylamine. During the reaction, the terminal trithiocarbonate groups of PVIAO were converted into thiol groups by aminolysis reaction,35 and the polymers can graft onto exfoliated MoS2-sheets through disulphide bonds29,36 and Coulomb interaction.37 The high surface area of MoS2-sheets would bring about a faster sorption.31 Furthermore, the positive charges of PVIAO can improve the sorption selectivity of uranyl tricarbonate complex.33,34 Therefore, it is expected that the sorbent MoS2-PVIAO could be used for effective uranium(VI) sorption from aqueous solutions.
Experimental
Materials and methods
1-Vinylimidazole (VI, CP), 2,2′-azoisobutyronitrile (AIBN, CP), sodium sulfite anhydrous (Na2SO3, AR) and n-butylamine (99%, AR) were bought from Sinopharm Chemical Reagent Co., Ltd. 4-Bromobutyronitrile (97%) and hydroxylamine hydrochloride (NH2OH·HCl, 99%) were obtained from J&K Chemical Co., Ltd. n-Butyllithium solution (1.6 mol L−1) in hexane was purchased from Adamas Reagent Co., Ltd. MoS2 (98.5%, GR) was purchased from Acros Organics Co., Ltd.
VI was pretreated by reduced pressure distillation and stored at −18 °C prior to use. AIBN was purified by recrystallization from 95% ethanol. (UO2(NO3)2·6H2O), (Fluka, AR) was dissolved to prepare the stock solutions of uranyl ions. S-1-Dodecyl-S′-(α,α′-dimethyl-α′′-acetic acid)trithiocarbonate (DDATC) was prepared in accordance to the previous literature38 (Fig. S1, ESI†). All other reagents and chemicals were used as received. The characterization methods were shown in ESI.†
Synthesis of MoS2-PVIAO
MoS2-sheets were first exfoliated by intercalation with n-butyllithium36 (ESI†), and PVIAO (Mn = 2900 g mol−1, PDI = 1.08) was prepared by RAFT polymerization (Fig. S2, ESI†). A typical synthetic method for MoS2-PVIAO was shown as follows: specifically, MoS2-sheets (0.2 g, 1.25 mmol) were dispersed in 200 mL of ultrapure water. Then, Na2SO3 (1.0 g, 7.93 mmol) and PVIAO (0.2 g, 0.07 mmol) were added into suspension with continuously stirring under an argon atmosphere for 0.5 h at room temperature. 2.0 mL of n-butylamine was injected into the mixture and then sonicated for 2 h. After the mixture was agitated for extra 20 h, it was dialyzed against continuous water flow with dialysis membranes (MWCO = 10 K). Then it was lyophilized to give the final black powder. For comparison, MoS2-PVIAO samples with different grafting degree (GD) were also prepared by the similar experiment and the recipes were shown in Table 1.
Table 1 Synthesis recipes of MoS2-PVIAO and graft degree of PVIAO
Sample |
MoS2-sheets (g) |
Na2SO3 (g) |
n-Butylamine (mL) |
PVIAO (g) |
GDa |
Graft degree was determined by TGA curve. |
MoS2-PVIAO-1 |
0.20 |
1.0 |
2.0 |
0.10 |
10.0% |
MoS2-PVIAO-2 |
0.20 |
1.0 |
2.0 |
0.20 |
17.1% |
MoS2-PVIAO-3 |
0.20 |
1.0 |
2.0 |
0.50 |
53.7% |
Sorption experiments
The distribution of uranium(VI) species was calculated using Medusa program under different pH conditions (Fig. S3A, ESI†), and the result showed uranyl tricarbonate complex is the dominant species of uranium(VI) in aqueous solutions when pH ≥ 8.0. Therefore, all sorption experiments were conducted at pH 8.0 and 298.15 K in polyethylene tubes. The pH values were adjusted by 2.0 mol L−1 HNO3 and 2.0 mol L−1 Na2CO3 solutions. The uranyl concentrations before and after sorption were analyzed by ICP-MS. The equilibrium sorption amount (qe) and sorption efficiency (SE) were determined based on eqn (1) and (2), respectively: |
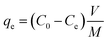 | (1) |
|
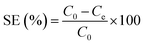 | (2) |
where C0 and Ce (mg L−1) represent the initial and final uranium concentrations, respectively. M (g) represents the desiccative sorbent weight, and V (L) denotes the volume of aqueous solution.
The first set of experiments was performed to demonstrate the effect of sorbent dose (0.03–2.00 g L−1) on uranium(VI) sorption with an initial concentration of 5.0 × 10−5 mol L−1. After shaking for 24 h until sorption equilibrium, the solid phase was separated by centrifugation.
The second set of experiments was conducted to check into the effect of contact time on the sorption of uranium(VI). Since the sorption equilibrium was achieved in a short time, the mixture was filtered immediately after sorption within different time.
The third set of experiments was carried out to examine the influence of various coexisting ions, salinity and different uranyl initial concentrations (5.0 × 10−5 to 3.3 × 10−4 mol L−1). A typical sorption procedure was described as follows: the sorbents (1.0 mg) were added into 5.0 mL of uranium(VI) solution and the mixture was shaking for 8 h until sorption equilibrium, and the sorbents were then removed by centrifugation. In addition, the total concentration of CO32− was close to 6.0 × 10−3 mol L−1 when pH was adjusted to ∼8.0 with Na2CO3 solutions. According to calculation with Medusa program, there is no precipitation of uranium(VI) in the solution under the experimental conditions (i.e. pH 8.0, [CO32−] = 6.0 × 10−3 mol L−1, and [U] = 3.3 × 10−4 mol L−1) (Fig. S3B, ESI†).
Desorption and regeneration experiment
The desorption and regeneration studies were conducted to assess the recyclability of the sorbent. The as-prepared MoS2-PVIAO (17.1%) powders (3.0 mg) were added into 10.0 mL of uranium(VI) solution under continuous oscillation. After saturated sorption, the mixture was dialyzed in HCl solution (0.1 mol L−1, 200 mL) and NaHCO3 solution (1.0 mol L−1, 200 mL) to remove uranium(VI), respectively. Ultrapure water (200 mL) was then used to wash for three times until neutral and lyophilized for reuse.
Simulated seawater experiment
Simulated seawater experiments were conducted with two models according to the ref. 12. Specifically, the simulated seawater was comprised of 0.438 mol L−1 NaCl, 2.297 mmol L−1 NaHCO3 and 0.034 mmol L−1 uranyl nitrate in ultrapure water. In addition, CaCl2 (0.01 mmol L−1) was also added into the solution to investigate the effect of calcium ions on sorption. The pH was adjusted to 8.0. MoS2-PVIAO (17.1%) (1.0 mg) and 5.0 mL of stock solution were placed in polyethylene tubes. The mixture was shaken for different period of time at room temperature. Finally the sorbents were removed by filtration.
Results and discussion
Characterization of MoS2-PVIAO
In order to synthesize MoS2-PVIAO, MoS2-sheets were first prepared by intercalating exfoliation of MoS2 powder with n-butyllithium,36 and PVIAO was synthesized by controlled polymerization of VI and subsequent reaction with 4-bromobutyronitrile and with hydroxylamine, respectively (ESI†). MoS2-PVIAO was then prepared by grafting PVIAO onto MoS2-sheets in the presence of n-butylamine. During the reaction, the terminal trithiocarbonate groups of PVIAO chains were converted into thiol groups, and the chains could connect with vacancy defects of exfoliated MoS2-sheets through disulphide bonds and Coulomb interaction.
The morphological features of MoS2-PVIAO were characterized by TEM and XRD. In comparison with MoS2 (Fig. 1A), the sheets of MoS2-PVIAO present obvious nano-sheets structure and the interlayer distance was about 1.0 nm (Fig. 1B and the inset). Furthermore, the better dispersion of MoS2-PVIAO indicated the successful graft of PVIAO onto MoS2-sheets (Fig. 1C). This point was further demonstrated by XRD patterns. After exfoliation and modification of MoS2, the characteristic peaks at 2θ = 13° and 41° for MoS2 (JCPDS card 37-1492) disappeared (Fig. 1D), suggesting the crystal structure was destroyed by polymer chains.
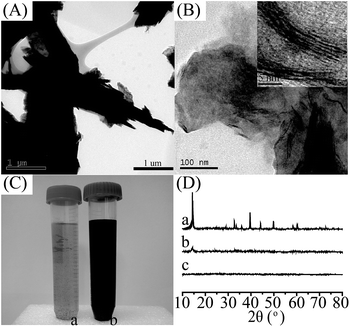 |
| Fig. 1 (A) TEM images of MoS2 (scale bar: 1 μm); (B) TEM images of MoS2-PVIAO (scale bar: 100 nm). Inset: an edge of individual MoS2-PVIAO (scale bar: 5 nm); (C) pictures of (a) MoS2-sheets and (b) MoS2-PVIAO (17.1%) (2.0 mg of solid was dispersed in 10.0 mL of water). (D) XRD powder patterns for (a) MoS2, (b) MoS2-sheets, and (c) MoS2-PVIAO (17.1%). | |
The chemical components of MoS2-PVIAO (17.1%) were characterized by XPS and FT-IR spectra. XPS scans showed the elements in MoS2-sheets and MoS2-PVIAO (17.1%), respectively (Fig. 2A). Compared with MoS2-sheets (Fig. 2A, trace a), MoS2-PVIAO (17.1%) (Fig. 2A, trace b) showed the new peak for N (1s) element, which could be curve-fitted with three peak components ascribed to C–N, C
N, and N–O species (Fig. 2B), suggesting the successful grafting of PVIAO onto MoS2-sheets. The chemical structures of MoS2-PVIAO (17.1%) were confirmed by FT-IR spectra (Fig. 2C, trace b). Compared with MoS2-sheets (Fig. 2C, trace a), the characteristic bands of C
N (ring, 1539 cm−1), N–O (835 cm−1) occurred for MoS2-PVIAO (Fig. 2C, trace b). Moreover, MoS2-PVIAO were positively charged after the graft of cationic polymer (Fig. S4, ESI†), and the more graft content, the higher zeta potential, which would be helpful for the sorption of negative uranyl complex.
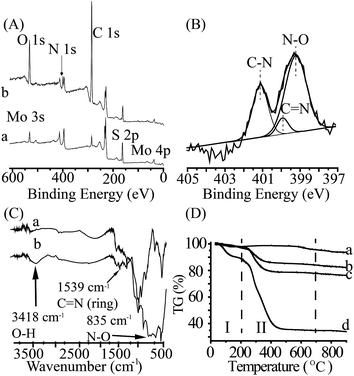 |
| Fig. 2 (A) XPS spectra of (a) MoS2-sheets and (b) MoS2-PVIAO (17.1%); (B) XPS spectra of N 1s of MoS2-PVIAO (17.1%); (C) FT-IR spectra of (a) MoS2-sheets and (b) MoS2-PVIAO (17.1%). (D) TGA curves of (a) MoS2-sheets, (b) MoS2-PVIAO (10.0%), (c) MoS2-PVIAO (17.1%) and (d) MoS2-PVIAO (53.7%). | |
In order to investigate chemical contents of MoS2-PVIAO, thermogravimetric analysis was carried out. The result was shown in Fig. 2D. There were two obvious stages during the process of mass loss: crystal water desorbed at stage I, whose weight percentage was approximately 2.2%; stage II was the process of decomposition of PVIAO, which can be used to determine the graft contents as 10.0%, 17.1% and 53.7% for MoS2-PVIAO, respectively.
Effects of sorbent dose on sorption
The influence of sorbent amount on uranium(VI) sorption was conducted at pH 8.0 and 298.15 K. The result was shown in Fig. 3A. With the increase of sorbent dose, sorption efficiency was first increased and then decreased, and there is a maximum value at 0.20 g L−1. The result may be attributed that the stacking of MoS2-sheets at high concentration, resulting in a reduction of the sorption efficiency. To confirm the speculation, SEM and particle size distributions were conducted for MoS2-PVIAO (17.1%) with different sorbent doses (Fig. S5, ESI†). The aggregation of the sorbents can be recorded obviously and particle size distributions were increasing with the sorbent dose. Therefore, the optimum sorbent dose of 0.20 g L−1 was selected for next experiments.
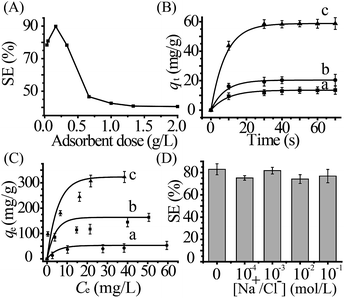 |
| Fig. 3 (A) The relationship between sorption efficiency and sorbent dose (0–60 mg L−1) in uranium(VI) sorption by MoS2-PVIAO (17.1%). (B) Effects of contact time on uranium(VI) sorption with 0.2 g L−1 sorbent dose: (a) MoS2-sheets, (b) MoS2-PVIAO (10.0%) and (c) MoS2-PVIAO (17.1%). (C) Sorption isotherm plots for uranium(VI) sorption with 0.2 g L−1 sorbent dose: (a) MoS2-sheets, (b) MoS2-PVIAO (17.1%) and (c) MoS2-PVIAO (53.7%). (D) The sorption efficiency of uranium(VI) by MoS2-PVIAO (17.1%, 0.2 g L−1 sorbent dose) in the presence of Na+/Cl− with different concentrations (experimental condition: 5.0 mL solution, pH 8.0, 5.0 × 10−5 mol L−1 uranium(VI) and 298.15 K). | |
Sorption kinetics
To investigate the sorption rate, the kinetic experiments were performed at pH 8.0 and 298.15 K with the optimum sorbent dose of 0.20 g L−1. The result was shown in Fig. 3B. The effect of contact time (t) on uranium(VI) sorption showed that all of the sorption reached equilibrium within 30 s, and the more grafting polymer, the more equilibrium amount.
Pseudo-first-order equation39 and pseudo-second-order equation40,41 were used to simulate the sorption kinetics (Fig. S6, ESI†), and the kinetics parameters were listed in Table 2. Kinetic profiles were appropriately described by pseudo-second-order model due to the larger correlation coefficients (R2) and the more accurate calculated qe. Importantly, there was a remarkably larger rate constant k2 of MoS2-PVIAO compared with the reference results (Table S1, ESI†). It was also noticed that the k2 for MoS2-PVIAO (10.0%) was slightly larger than MoS2-sheets, implying a more rapid sorption, which may be ascribed to electrostatic attraction between the positive sorbent and negative uranyl tricarbonate complex besides the good covalency between uranium and sulfur.18,19 However, the k2 for MoS2-PVIAO (17.1%) was much smaller than MoS2-PVIAO (10.0%), which may be attributed to the weaken interaction of MoS2 with uranium(VI) by relatively more polymer chains.
Table 2 Kinetic parameters for the sorption of uranium(VI) by MoS2-sheets, MoS2-PVIAO (10.0%) and MoS2-PVIAO (17.1%) (experimental condition: 5.0 mL solution, pH 8.0, 0.2 g L−1 sorbent dose, 5.0 × 10−5 mol L−1 uranium(VI) and 298.15 K)
Sample |
Pseudo-first order |
Pseudo-second order |
qe,exp (mg g−1) |
k1 (s−1) |
qe,cal (mg g−1) |
R2 |
k2 (g mg−1 s−1) |
qe,cal (mg g−1) |
R2 |
MoS2-sheets |
13.57 |
0.087 |
13.49 |
0.905 |
0.011 |
14.84 |
0.998 |
MoS2-PVIAO (10.0%) |
20.42 |
0.167 |
32.81 |
0.878 |
0.015 |
21.44 |
0.999 |
MoS2-PVIAO (17.1%) |
58.75 |
0.105 |
34.13 |
0.875 |
0.005 |
61.80 |
0.998 |
Sorption isotherm
To understand sorption capacity, the isotherm studies were carried out with the concentrations of uranium(VI) from 5.0 × 10−5 to 3.3 × 10−4 mol L−1 at 298.15 K and pH 8.0. The relationship between qe and Ce was shown in Fig. 3C. The sorption data were simulated with Langmuir and Freundlich models42,43 (Fig. S7, ESI†).
The correlation coefficients and parameters were summarized in Table 3. The sorption process was more appropriately described by Langmuir model according to the larger correlation coefficients (R2). The result may be ascribed to that active sites homogenously distribute on the surfaces, thereby leading to monolayer sorption. The capacity qmax of MoS2-PVIAO (53.7%) could reach 348.4 mg g−1, which was far larger than that of MoS2-sheets (57.97 mg g−1). In addition, there was a larger b value for MoS2-PVIAO than that for MoS2-sheets, which may be ascribed to the Coulomb force between cationic sorbents and negative uranyl complex.
Table 3 Langmuir and Freundlich parameters for uranium(VI) sorption by MoS2-sheets, MoS2-PVIAO (17.1%) and MoS2-PVIAO (53.7%) (experimental condition: 5.0 mL solution, pH 8.0, 0.2 g L−1 sorbent dose and 298.15 K)
Sorbent |
Langmuir |
Freundlich |
qmax (mg g−1) |
b (L mg−1) |
R2 |
KF (mol1−n Ln g−1) |
N |
R2 |
MoS2-sheets |
57.97 |
0.109 |
0.963 |
10.72 |
2.482 |
0.843 |
MoS2-PVIAO (17.1%) |
178.6 |
0.133 |
0.967 |
59.78 |
4.127 |
0.954 |
MoS2-PVIAO (53.7%) |
348.4 |
0.249 |
0.964 |
121.5 |
3.758 |
0.962 |
Effect of coexisting ions and salinity on uranium(VI) sorption
Considering that certain metals (Fe3+, Zn2+, etc.) may form precipitation at high [CO32−] and pH,44 the coexisting ions (Mg2+, Ca2+, K+, SO42−, Br−, BO33−, and VO3−) were selected for the experiments of uranium(VI) sorption to study the selectivity of MoS2-PVIAO (17.1%). All the coexisting ions are in the same concentration as those in the seawater.33,45 The distribution ratio (Kd) can be calculated by eqn (3): |
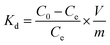 | (3) |
where C0 and Ce (mg L−1) are the concentrations of uranium(VI) in solution before and after sorption, respectively. V (L) represents the solution volume and m (g) is the mass of the sorbent.
The uranium(VI) uptake and the distribution ratio were listed in Table 4. The Kd of MoS2-PVIAO (17.1%) for uranium(VI) was remarkably larger than that of bare MoS2-sheets, indicating the higher sorption selectivity for MoS2-PVIAO (17.1%). This result may be attributed to positive charges of PVIAO that can repel the other cations, which improves the selectivity for uranium(VI). It was also noticed that the uranium(VI) uptake decreased to some extent when Mg2+, Ca2+ and VO3− existed, which may be attributed to the chemisorption of sulfur with the ions.
Table 4 Sorption of uranium(VI) on MoS2-sheets and MoS2-PVIAO (17.1%) in the presence of coexisting ions (experimental condition: 5.0 mL solution, pH 8.0, 0.2 g L−1 sorbent dose, a certain concentration of other ions, 5.0 × 10−5 mol L−1 uranium(VI) and 298.15 K)
No. |
Salt added |
Concentration mol L−1 |
Uranium(VI) uptake (mg g−1) |
Distribution ratio Kd (L g−1) |
MoS2-sheets |
MoS2-PVIAO (17.1%) |
MoS2-sheets |
MoS2-PVIAO (17.1%) |
1 |
Not added |
— |
15.52 |
51.66 |
2.431 |
142.0 |
2 |
MgCl2 |
5.2 × 10−2 |
6.701 |
39.92 |
0.856 |
17.46 |
3 |
Na2SO4 |
2.7 × 10−2 |
10.31 |
51.00 |
1.429 |
117.3 |
4 |
CaCl2 |
9.9 × 10−3 |
9.344 |
40.57 |
1.238 |
17.32 |
5 |
KCl |
9.7 × 10−3 |
13.10 |
48.38 |
1.974 |
63.66 |
6 |
KBr |
8.0 × 10−4 |
11.99 |
51.18 |
1.729 |
128.6 |
7 |
H3BO3 |
4.0 × 10−4 |
11.41 |
44.87 |
1.592 |
133.4 |
8 |
NH4VO3 |
5.1 × 10−5 |
14.84 |
30.22 |
0.924 |
43.51 |
To study the effect of salinity on uranium(VI) sorption, different concentrations of NaCl (10−4 to 10−1 mol L−1) were added for uranium(VI) sorption experiments. The result was depicted in Fig. 3D. The material remained a high sorption efficiency (76.8%) even at high salinity (0.1 mol L−1 NaCl), implying that the sorbent had a good salt resistance.
Regeneration studies
Regenerability is important for an effective and economical sorbent. In this study, the reusability of the sorbent was evaluated by five cycles of sorption/desorption using 0.1 mol L−1 HCl and 1.0 mol L−1 NaHCO3 solution as eluents, respectively. The results were shown in Fig. 4A. There was only a slight decrease of cycle efficiency (CE) after five cycles using HCl or NaHCO3 as eluents. In addition, elemental analysis was conducted for the sorbent after five cycles with 0.1 mol L−1 HCl eluent, and the result showed the nitrogen content slightly varied from 6.47% to 5.27% (Table S2, ESI†). The result might be attributed to a small amount of polymer on MoS2-sheets by Coulomb interaction, which could be washed off by HCl solution. To evaluate structure transformation of the sorbents, the sorbents after five cycles were characterized by FT-IR (Fig. S8, ESI†), and no obvious change was observed in material structure after elution with HCl and NaHCO3.
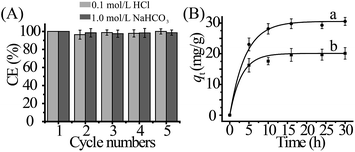 |
| Fig. 4 (A) Recycling of MoS2-PVIAO (17.1%) in the uranium(VI) sorption with different eluents: 0.1 mol L−1 HCl and 1.0 mol L−1 NaHCO3 (experimental condition: 10.0 mL solution, pH 8.0, 3.0 mg sorbent, 5.0 × 10−5 mol L−1 uranium(VI) and 298.15 K). (B) The sorption amount of uranium(VI) with different period of contact time in simulated seawater: (a) without calcium ions, and (b) with 0.01 mmol L−1 calcium ions (experimental condition: 5.0 mL solution, pH 8.0, 0.2 g L−1 sorbent dose, 0.034 mmol L−1 uranium(VI), 0.438 mol L−1 of NaCl, 2.297 mmol L−1 of NaHCO3 and 298.15 K). | |
Test with simulated seawater
Considering [UO2(CO3)3]4− is the dominant form of uranium(VI) in seawater, simulated seawater experiments were conducted in this study. The influence of contact time (t) on sorption amount (qt) was shown in Fig. 4B. It can be found that even at a lower uranium concentration with high salinity, the sorbent also can capture uranium(VI) within 10 h with a capacity of 28.6 mg g−1 at pH 8.0 and 298.15 K (Fig. 4Ba). When Ca2+ ions were present at the concentration of 0.01 mmol L−1, qmax was slightly reduced from 28.6 mg g−1 to 20.1 mg g−1 (Fig. 4Bb), which may be due to the formation of the specie Ca2(UO2)(CO3)3.46,47 The results indicated the sorbent may be used as a promising candidate for potential uranium(VI) extraction from seawater.
Conclusions
In summary, we show the amidoximated poly(vinyl imidazole)-functionalized MoS2 sheets for efficient capture of uranyl tricarbonate complex from aqueous solutions. The sorbent was prepared by grafting amidoximated poly(vinyl imidazole) onto MoS2-sheets. The effects of sorbent dose, sorption kinetics and isotherms, coexisting ions and salinity on uranium(VI) sorption were studied. The sorption follows pseudo-second-order kinetics model with larger rate constant, and the equilibrium can be reached within 30 s. The maximum sorption capacity can reach 348.4 mg g−1 at pH 8.0 and 298.15 K. The MoS2-PVIAO (17.1%) showed higher selectivity towards uranium(VI) over coexisting ions in comparison with MoS2-sheets. In addition, the sorbent exhibited remarkable salt-resistant stability and could be regenerated efficiently after five cycles with high sorption efficiency. As far as we know, this is the first report on MoS2-sheets for sorption of radionuclides from aqueous solution. This work demonstrates that MoS2-sheets can be used as the promising matrix materials for the separation of radionuclide from aqueous solution.
Acknowledgements
This work is supported by Natural Science Foundation of China (U1532111, 91326202), a Project Funded by the Priority Academic Program Development of Jiangsu Higher Education Institutions (PAPD), and Jiangsu Key Laboratory of Radiation Medicine and Protection.
References
- Uranium 2011, Resourses, Production and Demand, NEA No. 7059, Organisation for Economic Co-operation and Development/Nuclear Energy Agency, International Atomic Energy Agency, 2012 Search PubMed.
- Y. Lu, Nat. Chem., 2014, 6, 175–177 CrossRef CAS PubMed.
- R. V. Davies, J. Kennedy, R. W. Mcilroy, R. Spence and K. M. Hill, Nature, 1964, 203, 1110–1115 CrossRef.
- D. Beltrami, G. Cote, H. Mokhtari, B. Courtaud, B. A. Moyer and A. Chagnes, Chem. Rev., 2014, 114, 12002–12023 CrossRef CAS PubMed.
- F. P. Carvalho, J. M. Oliveira, I. Lopes and A. Batista, J. Environ. Radioact., 2007, 98, 298–314 CrossRef CAS PubMed.
- M. J. Manos and M. G. Kanatzidis, J. Am. Chem. Soc., 2012, 134, 16441–16446 CrossRef CAS PubMed.
- A. Baeza, M. Fernandez, M. Herranz, F. Legarda, C. Miro and A. Salas, Water, Air, Soil Pollut., 2006, 173, 57–69 CrossRef CAS.
- T. P. Rao, P. Metilda and J. M. Gladis, Talanta, 2006, 68, 1047–1064 CrossRef CAS PubMed.
- A. Dâas and O. Hamdaoui, J. Membr. Sci., 2010, 355, 214 CrossRef.
- I. Tabushi, Y. Kobuke and T. Nishiya, Nature, 1979, 280, 665–666 CrossRef CAS.
- Y. F. Yue, X. G. Sun, R. T. Mayes, J. Kim, P. F. Fulvio, Z. A. Qiao, S. Brown, C. Tsouris, Y. Oyola and S. Dai, Sci. China: Chem., 2013, 56, 1510–1515 CrossRef CAS.
- J. Gorka, R. T. Mayes, L. Baggetto, G. M. Veith and S. Dai, J. Mater. Chem. A, 2013, 1, 3016–3026 CAS.
- S. Das, S. Brown, R. T. Mayes, C. J. Janke, C. Tsouris, L. J. Kuo, G. Gill and S. Dai, Chem. Eng. J., 2016, 298, 125–135 CrossRef CAS.
- T. S. Anirudhan, A. R. Tharun, S. Rijith and P. S. Suchithra, J. Appl. Polym. Sci., 2011, 122, 874–884 CrossRef CAS.
- Y. B. Sun, C. C. Ding, W. C. Cheng and X. K. Wang, J. Hazard. Mater., 2014, 280, 399–408 CrossRef CAS PubMed.
- Y. B. Sun, D. D. Shao, C. L. Chen, S. B. Yang and X. K. Wang, Environ. Sci. Technol., 2013, 47, 9904–9910 CrossRef CAS PubMed.
- L. Shao, X. F. Wang, Y. M. Ren, S. F. Wang, J. R. Zhong, M. F. Chu, H. Tang, L. Z. Luo and D. H. Xie, Chem. Eng. J., 2016, 286, 311–319 CrossRef CAS.
- S. L. Ma, L. Huang, L. J. Ma, Y. Shim, S. M. Islam, P. L. Wang, L. D. Zhao, S. C. Wang, G. B. Sun, X. J. Yang and M. G. Kanatzidis, J. Am. Chem. Soc., 2015, 137, 3670–3677 CrossRef CAS PubMed.
- L. J. Ma, Q. Wang, S. M. Islam, Y. C. Liu, S. L. Ma and M. G. Kanatzidis, J. Am. Chem. Soc., 2016, 138, 2858–2866 CrossRef CAS PubMed.
- L. Zhou, M. Bosscher, C. S. Zhang, S. Ozcubukcu, L. Zhang, W. Zhang, C. J. Li, J. Z. Liu, M. P. Jensen, L. H. Lai and C. He, Nat. Chem., 2014, 6, 236–241 CrossRef CAS PubMed.
- M. Carboni, C. W. Abney, S. Liu and W. Lin, Chem. Sci., 2013, 4, 2396–2402 RSC.
- J. Kim, C. Tsouris, R. T. Mayes, Y. Oyola, T. Saito, C. J. Janke, S. Dai, E. Schneider and D. Sachde, Sep. Sci. Technol., 2013, 48, 367–387 CrossRef CAS.
- Y. P. V. Subbaiah, K. J. Saji and A. Tiwari, Adv. Funct. Mater., 2016, 26, 2046–2069 CrossRef.
- J. Q. Liu, Z. Y. Zeng, X. H. Cao, G. Lu, L. H. Wang, Q. L. Fan, W. Huang and H. Zhang, Small, 2012, 8, 3517–3522 CrossRef CAS PubMed.
- L. Liao, J. Zhu, X. J. Bian, L. N. Zhu, M. D. Scanlon, H. H. Girault and B. H. Liu, Adv. Funct. Mater., 2013, 23, 5326–5333 CrossRef CAS.
- L. Rapoport, N. Fleischer and R. Tenne, J. Mater. Chem., 2005, 15, 1782–1788 RSC.
- T. Y. Wang, L. Liu, Z. W. Zhu, P. Papakonstantinou, J. B. Hu, H. Y. Liu and M. X. Li, Energy Environ. Sci., 2013, 6, 625–633 CAS.
- J. N. Coleman, M. Lotya, A. O'Neill, S. D. Bergin, P. J. King, U. Khan, K. Young, A. Gaucher, S. De, R. J. Smith, I. V. Shvets, S. K. Arora, G. Stanton, H. Y. Kim, K. Lee, G. T. Kim, G. S. Duesberg, T. Hallam, J. J. Boland, J. J. Wang, J. F. Donegan, J. C. Grunlan, G. Moriarty, A. Shmeliov, R. J. Nicholls, J. M. Perkins, E. M. Grieveson, K. Theuwissen, D. W. McComb, P. D. Nellist and V. Nicolosi, Science, 2011, 331, 568–571 CrossRef CAS PubMed.
- H. Cheng, N. Dong, T. Bai, Y. Song, J. Wang, Y. Qin, B. Zhang and Y. Chen, Chemistry, 2016, 22, 4500–4507 CrossRef CAS PubMed.
- Y. H. Chao, W. S. Zhu, X. Y. Wu, F. F. Hou, S. H. Xun, P. W. Wu, H. Y. Ji, H. Xu and H. M. Li, Chem. Eng. J., 2014, 243, 60–67 CrossRef CAS.
- A. T. Massey, R. Gusain, S. Kumari and O. P. Khatri, Ind. Eng. Chem. Res., 2016, 55, 7124–7131 CrossRef CAS.
- T. Hiemstra, W. H. V. Riemsdijk, A. Rossberg and K. U. Ulrich, Geochim. Cosmochim. Acta, 2009, 73, 4437–4451 CrossRef CAS.
- Z. H. Zeng, Y. Q. Wei, L. Shen and D. B. Hua, Ind. Eng. Chem. Res., 2015, 54, 8699–8705 CrossRef CAS.
- Y. Wei, J. Qian, L. Huang and D. Hua, RSC Adv., 2015, 5, 64286–64292 RSC.
- D. B. Hua, L. J. Kuang and H. J. Liang, J. Am. Chem. Soc., 2011, 133, 2354–2357 CrossRef CAS PubMed.
- S. S. Chou, M. De, J. Kim, S. Byun, C. Dykstra, J. Yu, J. X. Huang and V. P. Dravid, J. Am. Chem. Soc., 2013, 135, 4584–4587 CrossRef CAS PubMed.
- A. S. Goloveshkin, I. S. Bushmarinov, A. A. Korlyukov, M. I. Buzin, V. I. Zaikovskii, N. D. Lenenko and A. S. Golub, Langmuir, 2015, 31, 8953–8960 CrossRef CAS PubMed.
- J. T. Lai, A. Debby Filla and R. Shea, Macromolecules, 2002, 35, 6754–6756 CrossRef CAS.
- K. Saito, K. Uezu, T. Hori, S. Furusaki, T. Sugo and J. Okamoto, AIChE J., 1988, 34, 411–416 CrossRef CAS.
- Y. S. Ho, J. Hazard. Mater., 2006, 136, 681–689 CrossRef CAS PubMed.
- Y. S. Ho and G. McKay, Chem. Eng. J., 1998, 70, 115–124 CrossRef CAS.
- M. J. Temkin and V. Pyzhev, Acta Physicochim. URSS, 1940, 12, 217–222 Search PubMed.
- H. Freundlich, Z. Phys. Chem., 1906, 57, 385–470 CAS.
- A. J. Rubin and J. D. Johnson, Anal. Chem., 1967, 39, 298–302 CrossRef CAS.
- M. Biesalski and J. Ruhe, Macromolecules, 2002, 35, 499–507 CrossRef CAS.
- A. O. Tirler and T. S. Hofer, Dalton Trans., 2016, 45, 4983–4988 RSC.
- W. H. Wu, C. Priest, J. W. Zhou, C. J. Peng, H. L. Liu and D. E. Jiang, J. Phys. Chem. B, 2016, 120, 7227–7233 CrossRef CAS PubMed.
Footnote |
† Electronic supplementary information (ESI) available: Synthesis of DDATC, PVIAO and MoS2-sheets, sorption kinetics and isotherms, comparison of rate constant, elemental analysis, distribution of U(VI) species, SEM images, zeta potential, and particles size distributions of MoS2 samples. See DOI: 10.1039/c6ra28051j |
|
This journal is © The Royal Society of Chemistry 2017 |
Click here to see how this site uses Cookies. View our privacy policy here.