DOI:
10.1039/C6RA28290C
(Paper)
RSC Adv., 2017,
7, 10748-10756
Synthesis of environmentally friendly, efficient and highly recyclable Lewis acid-type calix[4]arene catalysts containing flexible or bulky groups for the Mannich reaction†
Received
16th December 2016
, Accepted 1st February 2017
First published on 8th February 2017
Abstract
Two new calix[4]arene-based Lewis acid-type catalysts containing flexible and bulky groups (2 and 3) were successfully synthesized. Their structures were characterized using FTIR, 1H-NMR, COSY-NMR, 13C-NMR, APT-NMR, and elemental analysis techniques. Moreover, to provide easily recyclable, environmentally benign, cost effective and reusable catalysts, these calix[4]arene-based Lewis acid-type catalysts were grafted onto epoxyl-functionalized silica-coated Fe3O4 magnetic nanoparticles (EPPTMS-MN) to afford their corresponding calixarene-grafted magnetic nanoparticles (TMAC[4]But-MNP and P(Ph)3C[4]But-MNP). FTIR, TGA, TEM, VSM and elemental analysis techniques were performed to confirm their structures. In addition, the catalytic capabilities of four Lewis acid-type calixarene catalysts were evaluated in a three component Mannich reaction of benzaldehyde with aniline and acetophenone in aqueous media without co-solvents. The association of the effects of functional groups (flexible and bulky), molar equivalents, amount of water used, and reaction durations on their catalytic efficacies were also evaluated.
Introduction
The Mannich-type reaction and other related reactions requiring a catalyst are known as simple and substantial approaches for producing β-amino carbonyl compounds, which are potential intermediates for the fabrication of pharmaceutical agents and synthetically utilizable N-containing substances, via the reactions involving carbon–carbon bond formation.1–5 Numerous catalysts such as Brönsted-type acids, Lewis-type acids and several organic or inorganic catalysts have been developed to catalyze Mannich-type reactions.3,6–11 However, generally organic solvents or organic solvent–water mixtures are chosen as a medium for Mannich-type reactions due to the drastic solubility of most of the developed catalysts.9–13 In view of the toxicological effects of any organic solvent containing mixtures to the environment as well as human beings, developing a method for C–C bond construction via Mannich reactions in water is a challenging but important task in the field of green chemistry.14 Therefore, researchers continue to focus more attention on developing efficient catalysts that can be run in an aqueous media for Mannich-type reactions producing β-amino carbonyl compounds.
Calixarenes, produced via a condensation reaction of p-substituted phenols and formaldehyde, represent very useful building blocks.15–17 Because of their simple structural modification and unique structural properties, such as having different-sized cavities and comprising both hydrophilic and hydrophobic sites, a large number of applications of calixarenes in supramolecular chemistry have been reported.18–21 More recently, chemists are devoting greater attention to developing efficient calixarene-based catalysts to facilitate C–C bond formation in water.4,22,23
In our previous study, we designed a calix[4]arene-based quaternary ammonium salt containing 1-(2-furoyl)piperazine groups as a catalyst for three component Mannich reactions of benzaldehyde with aniline and acetophenone in water.4 We found that this calix[4]arene salt efficiently catalyzed the Mannich reaction to synthesize corresponding β-aminocarbonyl compounds in high yield during a short period of time.4 In this study, we have preliminarily adopted a strategy that involves the introducing flexible and bulky groups to Lewis acid-type calix[4]arene derivatives in order to elucidate their effects on the catalytic properties of calixarene-based catalysts. Thus, we successfully achieved the syntheses of two new calix[4]arene derivatives involves the introduction of flexible and bulky groups at the lower rim of calixarene. In addition, for the first time, these Lewis acid-type calix[4]arene derivatives were grafted onto an epoxysilica-coated Fe3O4 magnetic nanoparticle surface. From the standpoint of green chemistry, this method provides them with easily recyclable, environmentally benign, cost effective and reusable catalysts. Furthermore, their catalytic activities were investigated for the first time in the three component Mannich reaction of benzaldehyde with aniline and acetophenone in water without co-solvents.
Results and discussion
Synthesis of new Lewis acid-type calix[4]arene derivatives (2, 3), and their corresponding magnetic nanoparticles (TMAC[4]But-MNP, P(Ph)3C[4]But-MNP)
In our previous study, the catalytic capability of a calix[4]arene-based quaternary ammonium salt containing 1-(2-furoyl)piperazine groups were investigated in three component Mannich reactions of benzaldehyde with aniline and acetophenone in water.4 We found that 1-(2-furoyl)piperazine-functionalized calix[4]arene-based quaternary ammonium salt exhibited efficient catalytic behavior for the synthesis of a β-aminocarbonyl compound.4 Inspired by our results,4 in this study we aimed to extend the variety of Lewis acid-type calixarenes and to exploit the effects of flexible (containing alkyl quaternary-ammonium) and bulky (containing triphenylphosphonium) groups of Lewis acid-type calix[4]arene derivatives (2, 3) on their catalytic abilities in three component Mannich reactions of benzaldehyde with aniline and acetophenone in an aqueous media. Furthermore, these Lewis acid-type calix[4]arene derivatives (2, 3) were grafted for the first time onto silica-modified Fe3O4 magnetic nanoparticles in order to apply them as a Lewis acid-type catalyst in three component Mannich reactions in water without co-solvents. For this purpose, p-tert-butylcalix[4]arene (1) was synthesized according to literature procedure.24 The substitution of p-tert-butylcalix[4]arene (1) at the lower rim was conducted with (5-bromopentyl)trimethylammonium bromide and (3-bromopropyl)triphenylphosphonium bromide to form 25,27-bis[5-(trimethylammonium bromide)pentyloxyl]-26,28-dihydroxyl-5,11,17,23-tetra-tert-butylcalix[4]arene (2) and 25,27-bis[3-(triphenylphosphonium bromide)propyloxyl]-26,28-dihydroxyl-5,11,17,23-tetra-tert-butylcalix[4]arene (3) with a yield of 85% and 91%, respectively (Scheme 1). FTIR, 1H-NMR, 13C-NMR, COSY-NMR, 13C-APT NMR, and 31P-NMR spectroscopy and elemental analysis techniques were performed for the structural elucidation of Lewis acid-type calix[4]aren derivatives. The FTIR spectra of Lewis acid-type calix[4]arene derivatives (2 and 3) (Fig. S1 and S2; see ESI†) gives compatible information about their structures to the NMR spectra.
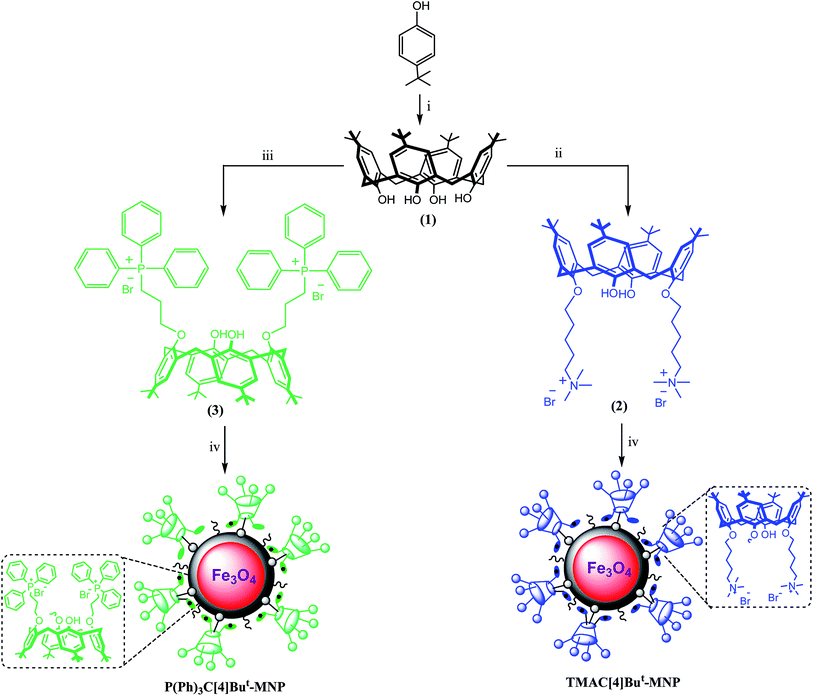 |
| Scheme 1 Synthesis of Lewis acid-type calix[4]arene derivatives. Reaction conditions; (i) HCHO, NaOH; (ii) (5-bromopentyl)trimethylammonium bromide, K2CO3; (iii) (3-bromopropyl)triphenylphosphonium bromide, K2CO3; (iv) EPPTMS-MN, NaH. | |
1H-NMR spectrum and two dimensional COSY-NMR spectrum, which leads to assign the interactions between the peaks (Fig. S3 and S4; see ESI†), of Lewis acid-type calixarene 2, indicated that the quaternary-ammonium with long alkyl subunits were successfully attached to at the lower rim of p-tert-butylcalix[4]arene, which resonances at 1.75–2.07 (12H), 3.45 (18H), 3.76 (4H), and 3.96 (4H) ppm for the protons of the –CH2, –CH3, –OCH2, and –NCH2 groups, respectively (Fig. S3; see ESI†). Moreover, a 13C-NMR spectra of the derivative 2 also contributed to our determination of its structure through the appearance of characteristic peaks at 22.8, 22.9 and 29.2 ppm for –CH2–, as well as at 33.9, 53.5, and 66.6 ppm for the –N+–CH3, –N+–CH2–, and –O–CH2– groups (Fig. S5; see ESI†).
The appeared peaks at 2.03 (4H), 3.93 (4H), 4.07 (4H) and 7.60–7.77 (30H) ppm for the protons of the –CH2, –OCH2, –+PCH2, and aromatic units of triphenylphosphonium groups, respectively in the 1H-NMR spectra of Lewis acid-type calix[4]arene bearing bulky groups 3 clearly indicate that the substitution of p-tert-butylcalix[4]arene with triphenylphosphonium moieties was successfully achieved (Fig. S6; see ESI†).
The 13C-NMR (Fig. S7; see ESI†) and APT-13C-NMR spectra of Lewis acid-type calix[4]arene 3 also contributed to determine its structure. The peaks belonging to the –CH3 (two peaks at 30.8 and 31.1 ppm for tert-butyl groups) and –CH (five peaks at 125.2, 125.5, 130.6, 133.6 and 135.3 ppm for aromatic units) groups appeared at the positive region, while the peaks for the –CH2 (four peaks at 19.6, 22.7, 31.6 and 74.5 ppm) and quaternary –C (eight peaks at 33.7, 33.8, 117.3, 118.1, 127.8, 131.7, 142.2, 147.1 and 148.7 ppm) groups assigned at the negative region (Fig. S8; see ESI†). The peaks in the 31P-NMR spectra of derivative 3 also confirmed that Lewis acid-type calix[4]arene 3 contained phosphonium groups (Fig. S9; see ESI†).
Preparation of Fe3O4 nanoparticles and [3-(2,3-epoxypropoxy)-propyl]-trimethoxysilane-coated Fe3O4 nanoparticles (EPPTMS-MN) was carried out according to literature procedures25 in order to fabricate Lewis acid-type calix[4]arenes-grafted Fe3O4 magnetic nanoparticles that were employed as a catalyst in three component Mannich reactions in water without co-solvents. For this goal, 25,27-bis[5-(trimethylammonium bromide)pentyloxyl]-26,28-dihydroxyl-5,11,17,23-tetra-tert-butylcalix[4]arene (2) and 25,27-bis[3-(triphenylphosphonium bromide)propyloxyl]-26,28-dihydroxyl-5,11,17,23-tetra-tert-butylcalix[4]arene (3) were grafted onto EPPTMS-MN to afford their corresponding nanoparticles, TMAC[4]But-MNP and P(Ph)3C[4]But-MNP (see Scheme 1). Their structures were estimated using a combination of FTIR spectroscopy, TEM, TGA and elemental analysis.
The FTIR spectra presented in ESI† and the appearance of notably characteristic IR peaks at 1482, 1439 and 1412 cm−1 for TMAC[4]But-MNP and 1479, 1458 and 1408 cm−1 for P(Ph)3C[4]But-MNP all of which are stretching vibrations of aromatic C
C bands of Lewis acid-type calix[4]arenes 2 and 3 clearly implied the accuracy of their structures (Fig. S1 and S2; see ESI†). Additional peaks indicating the stretching vibrations of the Si–O groups of the silica part of EPPTMS-MN appeared at 1072, 955 and 794 cm−1 for TMAC[4]But-MNP and 1152, 1081, 953 and 793 cm−1 for P(Ph)3C[4]But-MNP.
To determine the particle size and morphological distribution of the Fe3O4, TMAC[4]But-MNP and P(Ph)3C[4]But-MNP, a Transmission Electron Microscopy (TEM) was used (see Fig. 1). Fig. 1a indicates that the Fe3O4 nanoparticle had a particle size of 8 ± 3 nm. It also indicates that due to the magnetite properties of the particles, the particles created a magnetic field, resulting with piled up particles. However, the immobilization of calixarene derivatives, which have no magnetic features, worked to decrease this magnetic field and caused the distribution of the particles. Indeed, the electrostatic repulsion force and steric hindrance between the calixarenes also contributed to the particles' distribution. In addition, we found the size of particles that were 8–10 nm larger than the typical particle size of the Fe3O4 nanoparticle. The reason was probably the intermolecular interaction between calixarene parts of the nanoparticles caused the particles to aggregate.
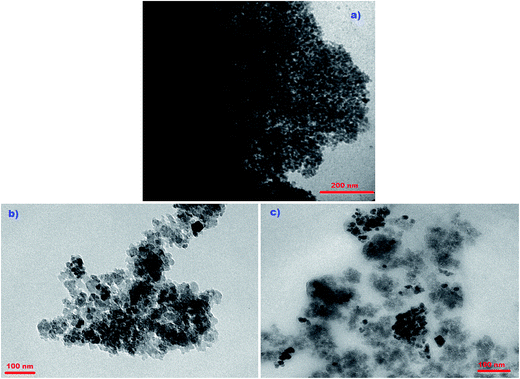 |
| Fig. 1 TEM images of (a); Fe3O4 magnetic nanoparticles, (b); TMAC[4]But-MNP, (c); P(Ph)3C[4]But-MNP. | |
To explore the amount of grafted calixarene derivatives onto the silica-coated magnetic nanoparticles, the elemental analysis technique was carried out. The results for TMAC[4]But-MNP, depicted in Table 1, show that 25,27-bis[5-(trimethylammonium bromide)pentyloxyl]-26,28-dihydroxyl-5,11,17,23-tetra-tert-butylcalix[4]arene (2 or Calix-2) was successfully grafted onto EPPTMS-MN. It was also found that TMAC[4]But-MNP contained 0.19% nitrogen, corresponding to 0.28 mmol of derivative 2 per g of support.
Table 1 Elemental analysis results of EPPTMS-MN and TMAC[4]But-MNP
|
C (%) |
H (%) |
N (%) |
Bounded amounta (mmol g−1) |
Calculated according to the N content. Ref. 26. |
EPPTMS-MNb |
13.20 |
2.61 |
|
|
TMAC[4]But-MNP |
16.24 |
3.51 |
0.19 |
0.28 |
We performed thermogravimetric analyses (TGA) of TMAC[4]But-MNP and P(Ph)3C[4]But-MNP to confirm the presence of Lewis acid-type calixarene derivatives on the surface of the Fe3O4 nanoparticles, as well as to investigate their thermal stabilities (Fig. 2). In the graph, the observed weight loss at 50–225 °C may be the result of the evaporated water and the other solvents (see Fig. 2). For TMAC[4]But-MNP, the weight loss of 17.2% at 250–650 °C can be attributed to the degradation of calixarene units (2 or Calix-2) and 3-(2,3-epoxypropoxy)-propyl groups (see Fig. 2a) while the weight loss of EPPTMS-MN was about 5% within a broad temperature range of 250 to 650 °C. For P(Ph)3C[4]But-MNP, thermal degradation of calixarene units (3 or Calix-3) and 3-(2,3-epoxypropoxy)-propyl groups resulted with the weight loss of 25.5% at 275–900 °C (see Fig. 2b).
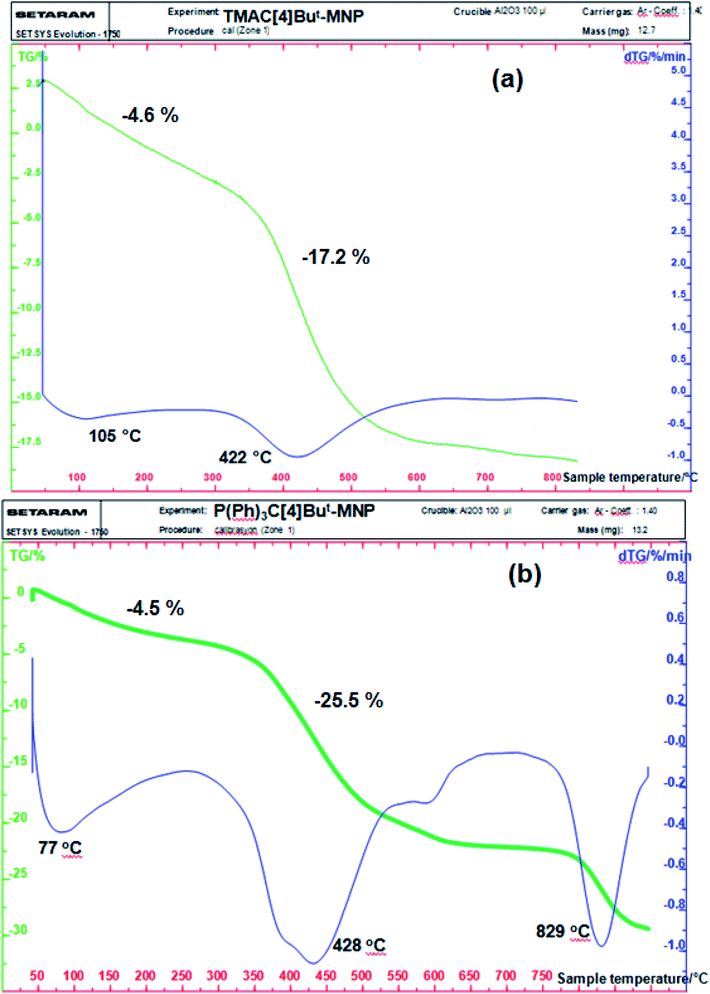 |
| Fig. 2 TGA curves of (a) TMAC[4]But-MNP, and (b) P(Ph)3C[4]But-MNP. | |
The magnetic properties of EPPTMS-MN, TMAC[4]But-MNP, and P(Ph)3C[4]But-MNP were investigated using VSM (Lake Shore 7407) analysis at room temperature (see Fig. 3). Fig. 3 indicates that all the sample had a superparamagnetic behavior and saturation magnetization of EPPTMS-MN, TMAC[4]But-MNP, and P(Ph)3C[4]But-MNP were found to be 44, 32 and 23 emu g−1, respectively. It can be clearly seen that immobilization of calixarene derivatives reduced the saturation magnetization value. However, it was an expected result because organic units onto the surface of magnetic nanoparticles were increased.
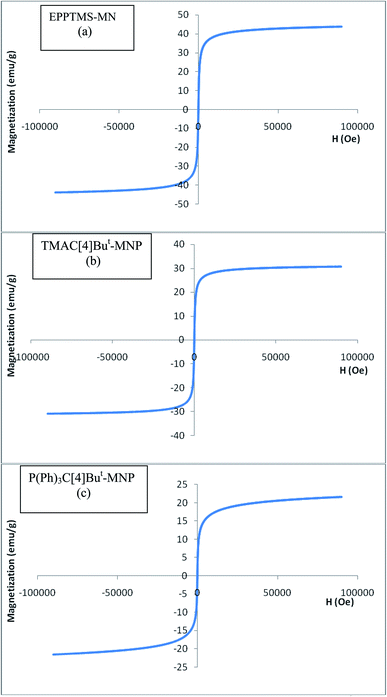 |
| Fig. 3 Magnetization curves of (a) EPPTMS-MN, (b) TMAC[4]But-MNP, and (c) P(Ph)3C[4]But-MNP. | |
Evaluation of catalytic efficiencies of Lewis acid-type calix[4]arene derivatives (Calix-2 and Calix-3), and their corresponding magnetic nanoparticles (TMAC[4]But-MNP and P(Ph)3C[4]But-MNP)
In our previous study, a calix[4]arene-based quaternary ammonium salt containing 1-(2-furoyl)piperazine groups was used as a Lewis acid-type catalyst in the three component Mannich reaction of benzaldehyde with aniline and acetophenone in water without co-solvents.4 It was observed that calix[4]arene-quaternary ammonium salt exhibited significant catalytic efficacy for the Mannich reactions. This study aimed to elucidate the effects of flexible and bulky groups on the catalytic efficacy of Lewis acid-type calix[4]arene catalysts (Calix-2 and Calix-3) for the three component Mannich reaction in an aqueous media. Thus, for the first time, we conducted an experiment to study the catalytic capabilities of Lewis acid-type calix[4]arene derivatives containing flexible or bulky groups. In addition, their corresponding magnetic nanoparticles were also employed as Lewis acid-type catalysts in a Mannich type reaction of benzaldehyde with aniline and acetophenone. From the experimental results depicted in Table 2, it can be seen that the corresponding Mannich product 4 were not produced even after 7 days when the commercial tetrabutylammonium bromide (TBAB) was chosen as the catalyst. However, by increasing the reaction time to 19 days, the target compound 4 was produced in a trace yield in the presence of TBAB (Table 2, entry 2). When Lewis acid-type calix[4]arene derivatives (Calix-2 or Calix-3) were used as catalysts, good yields of the corresponding Mannich product 4 were afforded in a shorter reaction time (see Table 2). On our first attempt, the addition of 0.5 mol% of Calix-2 and Calix-3 containing flexible and bulky groups (Table 2, entries 3 and 9) as catalysts in water (16 mL) to the three component Mannich reaction produced the β-aminocarbonyl compound 4 with the isolated yields of 52% for both of them after 6 h (Fig. S10 and S11; see the 1H and 13C NMR spectra of the Mannich adduct in the ESI†). The molar equivalent of Calix-2 and Calix-3 was used as 1 mol% under the same reaction conditions, and an increase on the value of the isolated yields of compound 4 was observed (Table 2, entries 4 and 10). However, using a 2 mol% molar equivalent of Calix-2 and Calix-3 in 16 mL of H2O (Table 2, entries 5 and 11) gave lower yields of the corresponding product 4, 35 and 27%, respectively after 6 h. This decrease on the isolated yields led us to consider that changing the other parameters such as the amount of water used or the reaction duration might increase the isolated yield of 4. Indeed, we found that increasing the reaction time resulted with higher yields (Table 2, entries 8 and 15). Moreover, using 3.5 mL of H2O in the presence of Calix-2 provided the highest recorded yield, 69% (Table 2, entry 6), whereas the lowest yield of the corresponding product 4 was obtained using 1.8 mL of H2O in the presence of Calix-2 (Table 2, entry 7). Changing the amount of water used with Calix-3 as the catalyst yielded similar results. However, the highest yield of Mannich product 4, as high as 77%, using catalyst Calix-3 (2 mol%) was obtained when we applied a 24 h reaction parameters using 1.8 mL of H2O (Table 2, entry 15). This finding suggests that the bulky groups contained in Calix-3 exhibited better catalytic efficacy than the flexible units contained Calix-2.
Table 2 Three component Mannich type reaction in the presence of Lewis acid-type calix[4]arene derivatives
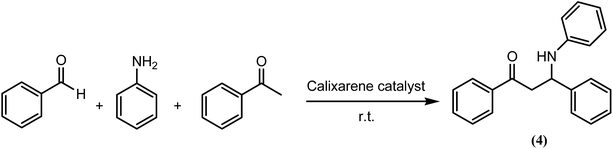
|
Entry |
Catalyst |
Catalyst loading |
H2O (mL) |
Time (h) |
Isolated yield (%) |
Ref. 4. Benzaldehyde (0.23 g, 2.19 mmol), aniline (2.19 mmol), acetophenone (2.19 mmol), NaOH (11 mmol), 170 rpm. Benzaldehyde (51 μL, 0.5 mmol), aniline (0.5 mmol), acetophenone (1.0 mmol), 170 rpm. Second reuse of calixarene-based catalysts. Third reuse of calixarene-based catalysts. |
1 |
None |
— |
2 |
168 |
Tracea,b |
2 |
TBAB |
10 mol% |
2 |
456 |
Tracea,b |
3 |
Calix-2 |
0.5 mol% |
16 |
6 |
52b |
4 |
Calix-2 |
1 mol% |
16 |
6 |
56b |
5 |
Calix-2 |
2 mol% |
16 |
6 |
35b |
6 |
Calix-2 |
2 mol% |
3.5 |
6 |
69b |
7 |
Calix-2 |
2 mol% |
1.8 |
6 |
34b |
8 |
Calix-2 |
2 mol% |
1.8 |
24 |
54b |
9 |
Calix-3 |
0.5 mol% |
16 |
6 |
52b |
10 |
Calix-3 |
1 mol% |
16 |
6 |
64b |
11 |
Calix-3 |
2 mol% |
16 |
6 |
27b |
12 |
Calix-3 |
2 mol% |
3.5 |
6 |
43b |
13 |
Calix-3 |
2 mol% |
1.8 |
6 |
68b |
14 |
Calix-3 |
2 mol% |
0.9 |
6 |
44b |
15 |
Calix-3 |
2 mol% |
1.8 |
24 |
77b |
16 |
P(Ph)3C[4]But-MNP |
10 mg |
4 |
6 |
59c |
17 |
P(Ph)3C[4]But-MNP |
5 mg |
4 |
6 |
36c |
18 |
P(Ph)3C[4]But-MNP |
5 mg |
2 |
6 |
38c |
19 |
P(Ph)3C[4]But-MNP |
5 mg |
1 |
6 |
62c, 62d, 62e |
20 |
P(Ph)3C[4]But-MNP |
5 mg |
0.5 |
6 |
43c |
21 |
P(Ph)3C[4]But-MNP |
5 mg |
1 |
72 |
99.9c |
22 |
TMAC[4]But-MNP |
10 mg |
4 |
6 |
44c |
23 |
TMAC[4]But-MNP |
5 mg |
4 |
6 |
36c |
24 |
TMAC[4]But-MNP |
5 mg |
2 |
6 |
46c |
25 |
TMAC[4]But-MNP |
5 mg |
1 |
6 |
68c, 68d, 68e |
26 |
TMAC[4]But-MNP |
5 mg |
0.5 |
6 |
42c |
27 |
TMAC[4]But-MNP |
5 mg |
1 |
72 |
99.9c |
28 |
EPPTMS-MN |
5 mg |
1 |
72 |
12c |
The catalytic properties of EPPTMS-MN, TMAC[4]But-MNP and P(Ph)3C[4]But-MNP were evaluated for the first time using the three component Mannich reaction of benzaldehyde with aniline and acetophenone in an aqueous media at room temperature. The corresponding Mannich product 4 was isolated in an excellent yield (∼100%). Optimization experiments, such as changing the amounts of catalyst and water, as well as altering the reaction time, revealed that the yield of Mannich product 4 increased as the amount of TMAC[4]But-MNP or P(Ph)3C[4]But-MNP increased (Table 2, entries 16, 17, 22 and 23). Furthermore, the influence of the amount of water on yields was investigated. We found that using 1 mL of H2O in the presence of P(Ph)3C[4]But-MNP or TMAC[4]But-MNP gave the highest yields as 62 and 68% (Table 2, entries 19 and 25), respectively, whereas the lowest yields of Mannich product 4 were afforded when 4 mL of H2O was used in the presence of P(Ph)3C[4]But-MNP or TMAC[4]But-MNP (Table 2, entries 17 and 23). Although EPPTMS-MN catalyzed this Mannich reaction in 1 mL of water to produce product 4 with a yield of 12% after 72 h (Table 2, entry 28), this percentage is the lowest percentage yield considering the long reaction time. When P(Ph)3C[4]But-MNP and TMAC[4]But-MNP were employed as catalysts in 1 mL of water, the highest yields for Mannich product 4 were obtained as 99.9 and 99.9%, respectively after 72 h (Table 2, entries 21 and 27). It can be concluded that P(Ph)3C[4]But-MNP and TMAC[4]But-MNP exhibited excellent catalytic efficacy with yields ∼100% for Mannich product in an aqueous media.
It is noteworthy that P(Ph)3C[4]But-MNP and TMAC[4]But-MNP are readily recycled and easily recovered from the reaction mixture due to their magnetic properties. As shown in Table 2 (entries 19 and 25), the reusability results reflected that P(Ph)3C[4]But-MNP and TMAC[4]But-MNP continued to produce high yields of 62 and 68%, respectively after third runs, which represents efficient sustainable catalytic activity.
Conclusion
In summary, two novel Lewis acid-type calix[4]arene derivatives (2 and 3) containing flexible or bulky groups as well as their corresponding magnetic nanoparticles (P(Ph)3C[4]But-MNP and TMAC[4]But-MNP) were synthesized and characterized. Moreover, these four Lewis acid-type calix[4]arene derivatives were applied as catalysts in the three component Mannich reaction of benzaldehyde with aniline and acetophenone in an aqueous media. It was found that these calix[4]arene-catalysts were quite capable to catalyze Mannich reaction to form corresponding Mannich product 4 in high yields over a short time. Moreover, catalytic efficacy of bulky groups-substituted calix[4]arene catalyst (Calix-3) was found higher than flexible groups-substituted calix[4]arene catalyst (Calix-2) under same reaction conditions. In addition, worthy of note is the fact that this effective catalysis system using a catalyst with magnetic property may open potential prospects in ‘green’ synthesis.
Experimental section
General
A TLC plate DC Alufolien Kieselgel 60 F254 (Merck) was used for TLC analyses. An Ez-Melt apparatus in a sealed capillary was employed to determine melting points of the synthesized compounds. 1H-NMR, 13C-NMR, 13C-APT, and 31P-NMR spectra were recorded on a Varian 400 MHz spectrometer. FTIR spectra, and elemental analyses were performed using a Perkin-Elmer 100 spectrometer, and a Leco CHNS-932 analyzer, respectively.
Synthesis of Lewis acid-type calix[4]arene derivatives
A literature procedure24 for the synthesis of p-tert-butylcalix[4]arene (1) was applied. Syntheses of 25,27-bis[5-(trimethylammonium bromide)pentyloxyl]-26,28-dihydroxyl-5,11,17,23-tetra-tert-butylcalix[4]arene (2 or Calix-2), 25,27-bis[3-(triphenylphosphonium bromide)propyloxyl]-26,28-dihydroxyl-5,11,17,23-tetra-tert-butylcalix[4]arene (3 or Calix-3), 25,27-bis[5-(trimethylammonium bromide)pentyloxyl]-26,28-dihydroxyl-5,11,17,23-tetra-tert-butylcalix[4]arene-grafted Fe3O4 nanoparticles (TMAC[4]But-MNP) and 25,27-bis[3-(triphenylphosphonium bromide)propyloxyl]-26,28-dihydroxyl-5,11,17,23-tetra-tert-butylcalix[4]arene-grafted Fe3O4 nanoparticles (P(Ph)3C[4]But-MNP) were reported here for the first time.
Synthesis of 25,27-bis[5-(trimethylammonium bromide)pentyloxyl]-26,28-dihydroxyl-5,11,17,23-tetra-tert-butylcalix[4]arene (2 or Calix-2). A mixture of p-tert-butylcalix[4]arene 1 (1.0 g, 1.541 mmol) and K2CO3 (0.53 g, 3.853 mmol) in anhydrous acetonitrile (25 mL) was degassed with N2 at 60 °C for 10 min. To this mixture, (5-bromopentyl)trimethylammonium bromide (0.94 g, 3.267 mmol) was added. The reaction mixture was stirred under reflux for 19 h. Then, the solvent was evaporated to dryness, and the residue was dissolved in CHCl3 (100 mL). This solution was washed with water to adjust pH 7.0. By evaporation of the solvent, obtained crude product was recrystallized from MeOH, and dried. Yield: 85%, melting point: 241–242 °C. 1H-NMR (400 MHz, CHCl3): δ 0.91 (s, 18H, But), 1.26 (s, 18H, But), 1.75–1.79 (m, 4H, –CH2–), 2.04–2.07 (m, 8H, –CH2–), 3.30 (d, 4H, J = 13.2 Hz, Ar-CH2-Ar), 3.45 (s, 18H, –CH3), 3.76 (t, 4H, J = 8.0 Hz, O–CH2–), 3.96 (t, 4H, J = 5.6 Hz, N–CH2–), 4.17 (d, 4H, J = 13.2 Hz, Ar-CH2-Ar), 6.73 (s, 4H, ArH), 7.03 (s, 4H, ArH), 7.11 (s, 2H, –OH). 13C-NMR (100 MHz, CDCl3): δ 22.8 (–CH2–), 23.0 (–CH2–), 29.2 (–CH2–), 31.0 (ButCH3), 31.7 (Ar-CH2-Ar), 33.8 (ButC), 33.9 (N+–CH3), 53.5 (N+–CH2–), 66.6 (O–CH2–), 125.3, 125.5, 127.8, 132.1, 142.0, 147.0, 149.7, 150.1. Anal. calcd for C60H92Br2N2O4 (%): C, 67.65; H, 8.71; N, 2.63. Found (%); C, 67.59; H, 8.76; N, 2.49.
Synthesis of 25,27-bis[3-(triphenylphosphonium bromide)propyloxyl]-26,28-dihydroxyl-5,11,17,23-tetra-tert-butylcalix[4]arene (3 or Calix-3). To a mixture of p-tert-butylcalix[4]arene (1 g, 1.541 mmol) and potassium carbonate (0.53 g, 3.853 mmol) in dry acetonitrile (25 mL) was added (3-bromopropyl)triphenylphosphonium bromide (1.52 g, 3.267 mmol). The mixture was stirred under reflux for 38 h. The reaction process was monitored by TLC (CH2Cl2/hexane, 1/1, (v/v)). After completion of the reaction, the solvent of the mixture was removed under reduced pressure. Remaining product was dissolved in chloroform, and washed with water to adjust pH 7.0. Organic phase was dried over MgSO4, filtered and evaporated to dryness. The crude was recrystallized from MeOH, and dried. Yield: 91%, melting points: 191–193 °C. FTIR (ATR): 1481 cm−1 (VP-Ar, sharp peak). 1H-NMR (400 MHz, CHCl3): δ 0.76 (s, 18H, But), 1.23 (s, 18H, But), 2.03 (brs, 4H, –CH2–), 3.07 (d, 4H, J = 13.2 Hz, Ar-CH2-Ar), 3.93 (d, 8H, J = 13.2 Hz, Ar-CH2-Ar and O–CH2–), 4.07 (brs, 4H, P–CH2–), 6.31 (s, 2H, –OH), 6.54 (s, 4H, ArH), 6.94 (s, 4H, ArH), 7.60–7.77 (m, 30H, ArH). 13C-NMR (100 MHz, CDCl3): δ 19.6 (P+–CH2–), 22.7 (–CH2–), 30.8 (–CH3, But), 31.1 (–CH3, But), 31.6 (Ar-CH2-Ar), 33.7 (–C, But), 33.8 (–C, But), 74.5 (O–CH2), 117.3 (–C, ArC), 118.1 (–C, ArC), 125.2 (–CH, ArC), 125.5 (–CH, ArC), 127.8 (–C, ArC), 130.7 (–CH, ArC), 131.7 (–C, ArC), 133.6 (–CH, ArC), 135.3 (–CH, ArC), 142.2 (–C, ArC), 147.1 (O–CAr), 148.7 (O–CAr). 31P-NMR (121 MHz, CHCl3): δ 24.1, 24.3, 24.4, 25.1, 29.0. Anal. calcd for C86H96Br2O4P2 (%): C, 72.98; H, 6.84. Found (%); C, 72.85; H, 6.89.
General procedure for the immobilizations of Calix-2 and Calix-3 onto EPPTMS-MN
NaH (0.05 g) and EPPTMS-MN (0.4 g) were added to a solution of calixarene derivative (0.4 g) in a mixture of THF/DMF (3/1, v/v), respectively. The mixture was stirred under reflux for 72 h. Then, calixarene-grafted nanoparticles was easily separated from the reaction mixture using a simple external magnet, and washed with chloroform in order to remove excess calixarene derivative from the calixarene-grafted magnetic nanoparticles. Obtained calixarene-grafted magnetic nanoparticles were washed with water until neutral pH was obtained, and dried in an oven.
25,27-Bis[5-(trimethylammonium bromide)pentyloxyl]-26,28-dihydroxyl-5,11,17,23-tetra-tert-butylcalix[4]arene-grafted Fe3O4 nanoparticles (TMAC[4]But-MNP). FTIR (KBr disk) cm−1: 3411 (VOH), 1657 (VH2O), 1479, 1414 (VC
C), 1084 (VSi–O), 796 (VSi–O) ve 563 (VFe–O). Anal. Found (%); C, 16.24; H, 3.51; N, 0.19.
25,27-Bis[3-(triphenylphosphonium bromide)propyloxyl]-26,28-dihydroxyl-5,11,17,23-tetra-tert-butylcalix[4]arene-grafted Fe3O4 nanoparticles (P(Ph)3C[4]But-MNP). FTIR (KBr disk) cm−1: 3405 (VOH), 1657 (VH2O), 1479 (VP-Ar), 1447, 1411 (VC
C), 1207 (VC–O–C), 1091 (VSi–O), 796 (VSi–O) ve 557 (VFe–O).
Typical procedure for Mannich reaction in the presence of Lewis acid-type calix[4]arene catalysts
A reported literature procedure was applied.4,27,28 Typically, calixarene-based catalysts was treated with NaOH in water (as listed in the table), and were added aldehyde, anilin and acetophenone, respectively. The reaction mixture was stirred at room temperature for the period of time (as given in the table). The crude was then purified by column chromatography (SiO2, ethylacetate/hexane). Melting points: 171–172 °C. 1H-NMR (400 MHz CDCl3): δ 3.27 (dd, 1H, J = 4.8, 12.4 Hz, –CH2–), 3.62 (dd, 1H, J = 9.2, 8.0 Hz, –CH2–), 4.95–5.00 (m, 1H, –CH–), 6.22 (d, 1H, J = 7.6 Hz, Ar-NH–), 6.42–6.49 (m, 3H, ArH), 6.95 (t, 2H, J = 8.4 Hz, ArH), 7.16 (t, 1H, J = 7.6 Hz, ArH), 7.27 (t, 2H, J = 7.6 Hz, ArH), 7.44 (d, 2H, J = 7.2 Hz, ArH), 7.50 (t, 2H, J = 8.0 Hz, ArH), 7.62 (t, 1H, J = 7.6 Hz, ArH), 7.95 (d, 2H, J = 6.8 Hz, ArH) (Fig. S10; see ESI†). 13C-NMR (100 MHz, CDCl3): δ 46.2 (–CH), 54.9 (–CH2), 114.0 (ArC), 118.0 (ArC), 126.4 (ArC), 127.4 (ArC), 128.2 (ArC), 128.7 (ArC), 128.8 (ArC), 129.1 (ArC), 133.5 (ArC), 136.6 (ArC), 142.8 (ArC), 146.7 (N–CAr), 198.2 (C
O) (Fig. S11; see ESI†). Anal. calcd for C21H19NO (%): C, 83.69; H, 6.35; N, 4.65. Found (%): C, 83.59; H, 6.48; N, 4.51.
References
- M. Arend, B. Westermann and N. Risch, Angew. Chem., Int. Ed., 1998, 37, 1044–1070 CrossRef.
- W.-B. Yi and C. Cai, J. Fluorine Chem., 2006, 127, 1515–1521 CrossRef CAS.
- Q. Tang, H.-J. Quan, S. Liu, L.-T. Liu, C.-F. Chow and C.-B. Gonga, J. Mol. Catal. A: Chem., 2016, 421, 37–44 CrossRef CAS.
- S. Sayin and M. Yilmaz, RSC Adv., 2014, 4, 2219–2225 RSC.
-
(a) B. List, P. Pojarliev, W. T. Biller and H. J. Martin, J. Am. Chem. Soc., 2002, 124, 827–833 CrossRef CAS PubMed;
(b) P. Hermange, M. E. T. H. Dau, P. Retailleau and R. H. Dodd, Org. Lett., 2009, 11, 4044–4047 CrossRef CAS PubMed.
- H. Ishitani, M. Ueno and S. Kobayashi, J. Am. Chem. Soc., 2000, 122, 8180–8186 CrossRef CAS.
- R. Müller, H. Goesmann and H. Waldmann, Angew. Chem., Int. Ed., 1999, 38, 184–187 CrossRef.
- H. Wu, Y. Shen, L. Y. Fan, Y. Wan, P. Zhang, C. F. Chen and W. X. Wang, Tetrahedron, 2007, 63, 2404–2408 CrossRef CAS.
- Y. Wu, C. Chen, G. Jia, X. Y. Zhu, H. M. Sun, G. F. Zhang, W. Q. Zhang and Z. W. Gao, Chem.–Eur. J., 2014, 20, 8530–8535 CrossRef CAS PubMed.
- K. Nagata, Y. Kuga, A. Higashi, A. Kinoshita, T. Kanemitsu, M. Miyazaki and T. Itoh, J. Org. Chem., 2013, 78, 7131–7136 CrossRef CAS PubMed.
- H. G. O. Alvim, G. A. Bataglion, L. M. Ramos, A. L. de Oliveira, H. C. B. de Oliveira, M. N. Eberlin, J. L. de Macedo, W. A. da Silva and B. A. D. Neto, Tetrahedron, 2014, 70, 3306–3313 CrossRef CAS.
- L. Mengozzi, A. Gualandi and P. G. Cozzi, Chem. Sci., 2014, 5, 3915–3921 RSC.
- S. Kobayashi, T. Hamada and K. Manabe, J. Am. Chem. Soc., 2002, 124, 5640–5641 CrossRef CAS PubMed.
- T. Akiyama, J. Takaya and H. Kagoshima, Adv. Synth. Catal., 2002, 344, 338–347 CrossRef CAS.
- S. Sayin, E. Akoz and M. Yilmaz, Org. Biomol. Chem., 2014, 12, 6634–6642 CAS.
-
(a) T. Klejch, J. Slavíček, O. Hudeček, V. Eigner, N. A. Gutierrez, P. Cuřínová and P. Lhoták, New J. Chem., 2016, 40, 7935–7942 RSC;
(b) Y. Yeon, S. Leem, C. Wagen, V. M. Lynch, S. K. Kim and J. L. Sessler, Org. Lett., 2016, 18, 4396–4399 CrossRef CAS PubMed.
-
(a) S. Memon, A. A. Bhatti, A. A. Bhatti and I. B. Solangi, Desalin. Water Treat., 2016, 57, 1844–1856 CrossRef CAS;
(b) S. Akın, M. Gülen, S. Sayin, H. Azak, H. B. Yıldız and S. Sönmezoğlu, J. Power Sources, 2016, 307, 796–805 CrossRef.
- P. Sarkar and C. Mukhopadhyay, Green Chem., 2016, 18, 6556–6563 RSC.
- A. Casnati, Chem. Commun., 2013, 49, 6827–6830 RSC.
- S. Sayin, H. Azak, H. B. Yildiz, P. Camurlu, G. U. Akkus, L. Toppare and M. Ersoz, Phys. Chem. Chem. Phys., 2015, 17, 19911–19918 RSC.
-
(a) V. Bagnacani, V. Franceschi, L. Fantuzzi, A. Casnati, G. Donofrio, F. Sansone and R. Ungaro, Bioconjugate Chem., 2012, 23, 993–1002 CrossRef CAS PubMed;
(b) F. Özcan, M. Bayrakcı and Ş. Ertul, J. Inclusion Phenom. Macrocyclic Chem., 2016, 85, 49–58 CrossRef;
(c) R. Salvio, S. Volpi, R. Cacciapaglia, F. Sansone, L. Mandolini and A. Casnati, J. Org. Chem., 2016, 81, 9012–9019 CrossRef CAS PubMed;
(d) E. Ozyilmaz, M. Bayrakci and M. Yilmaz, Bioorg. Chem., 2016, 65, 1–8 CrossRef CAS PubMed;
(e) S. Memon, A. A. Bhatti, A. A. Bhatti, Ü. Ocak and M. Ocak, J. Fluoresc., 2016, 26, 1591–1599 CrossRef CAS PubMed.
-
(a) S. Sayin and M. Yilmaz, Tetrahedron, 2016, 72, 6528–6535 CrossRef CAS;
(b) M. De Rosa, P. La Manna, A. Soriente, C. Gaeta, C. Talotta and P. Neri, RSC Adv., 2016, 6, 91846–91851 RSC;
(c) S. Sayin and M. Yilmaz, Tetrahedron, 2014, 70, 6669–6676 CrossRef CAS.
- M. Rahman, I. Ling, N. Abdullah, R. Hashim and A. Hajra, RSC Adv., 2015, 5, 7755–7760 RSC.
- C. D. Gutsche, Single step synthesis and properties of calixarenes In Calixarenes: A Versatile Class of Macrocyclic Compounds, ed. Vicens, Topics in Inclusion Science, 1990, vol. 3, pp. 3–37 Search PubMed.
-
(a) L. Zeng, K. Luo and Y. Gong, J. Mol. Catal. B: Enzym., 2005, 38, 24–30 CrossRef;
(b) Y. Yong, Y. Bai, Y. Li, L. Lin, Y. Cui and C. Xia, J. Magn. Magn. Mater., 2008, 320, 2350–2355 CrossRef CAS.
- S. Sayin and M. Yilmaz, J. Chem. Eng. Data, 2011, 56, 2020–2029 CrossRef CAS.
- W. Shen, L.-M. Wang and H. Tian, J. Fluorine Chem., 2008, 129, 267–273 CrossRef CAS.
-
(a) K. Manabe and S. Kobayashi, Org. Lett., 1999, 1, 1965–1967 CrossRef CAS;
(b) T. Ollevier and E. Nadeau, J. Org. Chem., 2004, 69, 9292–9295 CrossRef CAS PubMed;
(c) T. Akiyama, K. Matsuda and K. Fuchibe, Synlett, 2005, 322–324 CrossRef CAS;
(d) N. Azizi, L. Torkiyan and M. R. Saidi, Org. Lett., 2006, 8, 2079–2082 CrossRef CAS PubMed.
Footnote |
† Electronic supplementary information (ESI) available. See DOI: 10.1039/c6ra28290c |
|
This journal is © The Royal Society of Chemistry 2017 |
Click here to see how this site uses Cookies. View our privacy policy here.