DOI:
10.1039/C7RA01568B
(Paper)
RSC Adv., 2017,
7, 26420-26427
Synthesis and properties of fluorinated polyimides with multi-bulky pendant groups
Received
7th February 2017
, Accepted 23rd April 2017
First published on 17th May 2017
Abstract
A new aromatic diamine monomer, 2,2′-bis[3,5-di(4-trifluoromethylphenyl)-4-(4-aminophenoxy)phenyl]sulfone, containing four pendant trifluoromethylphenyl group substituents was successfully synthesized via a three-step reaction from readily available reagents. A new series of fluorinated polyimides with multi-bulky pendant groups was prepared from the diamine monomer with three commercially available aromatic tetracarboxylic dianhydrides using one-step high-temperature polycondensation. The incorporation of multi-bulky pendant fluorinated groups and large non-coplanar structures brought about a great improvement in their properties. The resulting polyimides had excellent solubility and the maximum solubility even reached 20 wt% in NMP, CHCl3, and THF at room temperature. Their film displayed a light color and high optical transparency with the cut-off wavelengths ranging from 327 to 343 nm. They also possessed intrinsically low dielectric constant values of 2.69–2.85 (at 1 MHz) and low water absorption (0.59–0.68%). Moreover, these polyimides showed high glass transition temperatures (259–281 °C) and excellent thermal stability with 5% weight loss at temperatures over the range of 551–561 °C and 515–520 °C under nitrogen and air atmospheres, respectively. Compared to some trifluoromethyl-substituted polyimides, these polyimides with multi-bulky pendant groups possessed better solubility and lower cut-off wavelength and dielectric constants.
Introduction
Aromatic polyimides (PIs) are considered to be one of the most successful high performance polymers and have shown important application value in the fields of aerospace and microelectronics due to their excellent thermal, mechanical, and electrical properties.1–7 However, most polyimides like other aromatic polymers are insoluble and infusible in the fully imidized form because of their high molecular rigidity and strong intermolecular interaction, thus leading to processing difficulties. Moreover, the commercial polyimide films generally possess relatively high dielectric constant (3.5 at 1 MHz) and have strong absorption in the ultraviolet-visible region. With the development of miniaturization of electronic devices, the performances of polyimides are required to be further optimized in terms of their electronic and optical properties. To overcome these problems, structural design and modification become very necessary and significant. A variety of methods for the chemical modification of polyimides have been attempted to improve their solubility and dielectric and optical properties.8 Incorporation of bulky pendant groups along the polymer backbone is regarded as one of the effective strategies.9–15 For example, Chern reported a series of polyimides with di-tert-butyl pendant groups in the repeating unit, prepared by one-step polycondensation.9 These polyimides had good solubility in some aprotic solvents and exhibited low dielectric constants with the values of 2.78–3.02 at 1 MHz. Swage prepared a series of triptycene polyimides based on 2,6-diaminotriptycene derivatives and five- and six-membered ring anhydrides.13 These completely aromatic polyimides were soluble in some organic solvents and their films possessed high surface areas and low refractive indices and dielectric constants. However, the incorporation of these bulky alkyl or alicyclic groups generally degrades the thermal stabilities of the obtained polyimides. In addition, the preparation of polyimides with bulky pendant alkyl groups is usually very complicated, and the key issue is how to design and develop new suitable monomers.
In recent years, significant attention has been focused on fluorinated polyimides, especially on trifluoromethyl-substituted polyimides.16 These fluorinated polyimides display reduced dielectric property as well as improved solubility and optical transparency as compared to their non-fluorinated counterparts.17–24 The pendant trifluoromethyl groups played a key role in improving the performance of polyimides because of their bulky free volume, low polarizability, and high hydrophobicity. They are believed to be a new generation of microelectronic and optoelectronic materials and have important application value. However, some of these fluorinated polyimides derived from BPDA still showed a limited solubility and were nearly insoluble in all the tested solvents.17–20 From the point of view of structural design, polyimides will possess better solubility and lower dielectric properties with the introduction of more pendant trifluoromethyl groups or more bulky fluorinated groups. Generally, it is convenient to obtain fluorinated polyimides with one or two trifluoromethyl groups in the repeating unit; however, it is difficult to obtain polyimides with multiple trifluoromethyl groups or bulky fluorinated groups due to difficulty in the preparation of fluorinated monomers.
As part of the efforts made to obtain highly soluble PIs with eminent dielectric and optical properties for microelectronic and photoelectric applications, the present study reports a new aromatic diamine and a series of fluorinated polyimides containing four pendant trifluoromethylphenyl groups in the repeating unit prepared via one-step high-temperature polycondensation. These polymers are expected to show excellent solubility and dielectric and optical properties due to the incorporation of multi-bulky fluorinated pendant groups. To the best of our knowledge, there are very few reports about fluorinated polyimides with multi-bulky pendant fluorinated groups in the repeating unit. The structures, solubility, dielectric constant, optical transparency, and thermal/mechanical properties of the obtained polyimides were investigated and discussed in detail.
Experimental
Materials and measurements
4,4′-Difluorodiphenyl sulfone (DFDPS), N-bromosuccinimide (NBS), and 4-aminophenol were purchased from TCI company and used as received. 4-(Trifluoromethyl)benzeneboronic acid was purchased from Soochiral Chemical Co. and used as received. Tetrakis(triphenylphosphine) palladium (0) was purchased from Shanxi Kaida Chemical Co. and used as received. Aromatic tetracarboxylic dianhydrides such as 3,3′,4,4′-biphenyltetracarboxylic dianhydride (BPDA or 4a), 3,3′,4,4′-benzophenonetetracarboxylic dianhydride (BTDA or 4b), and 4,4′-oxydiphthalic dianhydride (ODPA or 4c) were purchased from TCI company and further purified by recrystallization from acetic anhydride. Commercially available N-methyl-2-pyrrolidinone (NMP), m-cresol, N,N-dimethylacetamide (DMAc), N,N-dimethylformamide (DMF), dimethyl sulfoxide (DMSO), tetrahydrofuran (THF), and other reagents were used as received.
NMR spectra were obtained using a Bruker AV400 instrument with dimethyl sulfoxide-d6 (DMSO-d6) or CDCl3 as the solvent and tetramethylsilane (TMS) as the internal standard. FTIR spectra were obtained using a Nicolet NEXUS 670 spectrometer. Gel permeation chromatography (GPC) on soluble polymers was performed using a Waters 1515 system equipped with a triple detector array and a packing column (StyragelHT4 WAT044210, 7.8 × 300 mm) using tetrahydrofuran (THF) as an eluent at 25 °C and the flow rate of 1 mL min−1. The glass transition temperatures Tg of the membranes were characterized using differential scanning calorimetry (DSC, PE diamond DSC instrument). The samples were tested for two consecutive scans at the heating rate of 20 °C min−1. The first cycle of ramping and cooling was carried out to eliminate any thermal history of the samples. The Tg of each sample was obtained based on the mid-point transition temperature of the second heating curve. Thermogravimetric analysis (TGA) of the polymer samples was performed using a Netzsch TG 209F1 instrument at the heating rate of 20 °C min−1 under a nitrogen and air atmosphere. The tensile properties were obtained using an Instron 5565 Tensile Apparatus with a 5 kg load cell at the crosshead speed of 5 mm min−1 on the strips approximately 60–80 μm thick and 0.5 cm wide with a 2 cm gauge length. An average of at least three individual determinations was used. Ultraviolet-visible (UV-vis) spectra of the polymer films were obtained using a PerkinElmer Lambda35 UV-vis spectrophotometer at room temperature. The equilibrium water absorption was determined by measuring the weight changes in the vacuum-dried film specimens before and after immersion in deionized water at 25 °C for 3 days. The dielectric property of the polymer films was measured by the parallel-plate capacitor method using a TH2826 LCR Meter. Gold electrodes were vacuum-deposited on both surfaces of the dried films before testing, and the experiments were performed in a dry chamber.
Synthesis of 2,2′-bis(3,5-dibromo-4-fluorophenyl)sulfone (1). 2,2′-Bis(3,5-dibromo-4-fluorophenyl)sulfone (mp: 180–182 °C) was conveniently synthesized according to a procedure reported in our previous study.25
Synthesis of 2,2′-bis[3,5-di(4-trifluoromethylphenyl)-4-fluorophenyl]sulfone (2). Herein, 22.79 g (0.04 mol) of 2,2′-bis(3,5-dibromo-4-fluorophenyl)sulfone and 31.14 g (0.164 mol) of 4-(trifluoromethyl)benzeneboronic acid were dissolved in 500 mL of toluene/ethylene glycol dimethyl ether (1
:
1, v/v) in a 2 L three-necked round-bottom flask equipped with a reflux condenser, mechanical stirrer, and nitrogen inlet at 50 °C. After this, 200 mL of 15 wt% aqueous sodium carbonate solution and 3.7 g of 9% tetrakis(triphenylphosphine) palladium (0) were carefully added to the solution, and then, the reaction mixture was heated at 110 °C for 10 h. Afterward, toluene was evaporated to obtain a suspended solution, and the precipitate was obtained by filtration. The crude product was recrystallized from toluene to afford pure white crystalline 2,2′-bis[3,5-di(4-trifluoromethylphenyl)-4-fluorophenyl]sulfone. Yield: 80%, mp: 315–316 °C.FT-IR (KBr) ν/cm−1: 2926, 1329, 1168, 1120. 1H NMR (CDCl3, 400 MHz) δ: 8.07 (d, J = 6.3 Hz, 4H), 7.76 (d, J = 8.3 Hz, 8H), 7.67 (d, J = 8.0 Hz, 8H).
Synthesis of 2,2′-bis[3,5-di(4-trifluoromethylphenyl)-4-(4-aminophenoxy)-phenyl]-sulfone (3). Herein, 24.92 g (0.03 mol) of intermediate compound 2, 6.76 g (0.062 mol) of 4-aminophenol, 8.57 g (0.062 mol) of potassium carbonate, and 70 mL of DMF were added in a 250 mL four-necked round-bottom flask equipped with a reflux condenser, mechanical stirrer, and nitrogen inlet. The reaction mixture was stirred under a nitrogen atmosphere at 110 °C for 10 h. Then, the solution was poured in water to precipitate the pale-white solid, which was filtered and repeatedly washed with water. The crude product was further purified using a silica gel column with a 4
:
1 (v/v) mixture of dichloromethane and petroleum ether (60–90 °C) as the eluent. Yield: 61%; mp: 261–263 °C by DSC at the scan rate of 10 °C min−1.FT-IR (KBr) ν/cm−1: 3416, 3365, 2923, 1617, 1508, 1326, 1224, 1166, 1127, 1104. 1H NMR (CDCl3, 400 MHz) δ: 8.04 (s, 4H), 7.57 (m, 16H), 6.27 (d, J = 6.8 Hz, 4H), 6.20 (d, J = 6.8 Hz, 4H), 3.32 (s, 4H). 13C-NMR (100 MHz, CDCl3, ppm): 154.5, 150.0, 141.4, 139.5, 138.1, 136.9, 130.5, 130.2 ((q, 2JC–F = 25.8)), 129.5, 125.3, 123.8 (q, 1JC–F = 218.8), 116.7, 115.8. Elemental analysis: calcd for C52H32F12N2O4S (1008.87): C 61.91%, H 3.20%, N 2.78%. Found: C 62.00%, H 3.28%, N 2.74%.
Synthesis of fluorinated polyimides (5a–c). Polymerization was carried out by treating the synthesized diamine 3 with three structurally different aromatic dianhydride monomers. The general procedure for the polymer preparation was as follows. Diamine 3 (1.5 mmol) and dianhydride monomer 4 (1.5 mmol) were first dissolved in 12 mL of m-cresol in a completely dried 50 mL three-necked round-bottom flask equipped with a mechanical stirrer, a condenser, and a nitrogen inlet/outlet. Under mechanical stirring at room temperature for 30 min, isoquinoline (ca. 4 drops) was added. Then, the reaction solution was heated at 190 °C for about 12–20 h. Water formed during imidization was continuously removed using a stream of nitrogen. At the end of the reaction, the highly viscous polymer solution was cooled and precipitated using 300 mL ethanol. The resulting fiber-like precipitate was obtained by filtration, washing with hot water several times, and drying overnight at 120 °C under vacuum.
PI 5a. FT-IR (film) ν/cm−1: 3061, 1778, 1733, 1616, 1509, 1385, 1320, 1230, 1176, 1130, 1051, 735. 1H NMR (CDCl3, 400 MHz) δ: 8.12 (m, 6H), 8.01 (s, 4H), 7.62 (m, 16H), 7.10 (d, J = 8.0 Hz, 4H), 6.56 (d, J = 8.0 Hz, 4H). Elemental analysis: calcd for C68H34F12N2O8S (1267.05): C 64.46%, H 2.70%, N 2.21%. Found: C 63.80%, H 2.68%, N 2.18%.
PI 5b. FT-IR (film) ν/cm−1: 3061, 1778, 1733, 1615, 1508, 1383, 1321, 1231, 1159, 1123, 1050, 730. 1H NMR (CDCl3, 400 MHz) δ: 8.19 (m, 4H), 8.12 (s, 4H), 8.06 (d, J = 8.0 Hz, 2H), 7.61 (m, 16H), 7.08 (d, J = 8.0 Hz, 4H), 6.56 (d, J = 8.0 Hz, 4H). Elemental analysis: calcd for C69H34F12N2O9S (1295.06): C 63.99%, H 2.65%, N 2.16%. Found: C 63.57%, H 2.58%, N 2.11%.
PI 5c. FT-IR (film) ν/cm−1: 3070, 1778, 1733, 1608, 1509, 1383, 1320, 1275, 1240, 1168, 1123, 1050, 735. 1H NMR (CDCl3, 400 MHz) δ: 8.11 (m, 4H), 7.92 (d, J = 8.0 Hz, 2H), 7.60 (m, 16H), 7.45 (s, 2H), 7.40 (d, J = 8.0 Hz, 2H), 7.05 (d, J = 8.0 Hz, 4H), 6.54 (d, J = 8.0 Hz, 4H). Elemental analysis: calcd for C68H34F12N2O9S (1283.05): C 63.66%, H 2.67%, N 2.18%. Found: C 63.20%, H 2.58%, N 2.13%.
Results and discussion
Synthesis of the monomer
As depicted in Scheme 1, the new diamine monomer 2,2′-bis[3,5-di(4-trifluoromethylphenyl)-4-(4-aminophenoxy)phenyl]-sulfone (3) was synthesized by a three-step reaction using 4,4′-difluorodiphenyl sulfone and NBS as the starting materials. First, the precursor 1 was synthesized via a free-radical substitution reaction using concentrated sulfuric acid as the solvent and catalyst according to a reported procedure. Following this, the intermediate compound 2 was produced by a Suzuki coupling reaction of 1 with 4-(trifluoromethyl)benzeneboronic acid. Finally, the diamine monomer 3 was obtained via a nucleophilic substitution reaction of 2 and 4-aminophenol in the presence of potassium carbonate in DMF. The crude diamine 3 was purified using a silica gel column and directly used for the synthesis of PIs. The structure of diamine 3 was confirmed by FTIR and NMR spectroscopies and elemental analysis. In the FTIR spectrum, diamine 3 exhibited the characteristic N–H stretching bands at 3300–3500 cm−1, together with some strong absorption bands in the region of 1100–1300 cm−1 because of the C–O and C–F stretching (Fig. 1a). The characteristic sulfone groups and phenyl rings (Ar–H and C–C) stretching peaks appeared at 1326, 2923, 1617, and 1508 cm−1. Fig. 2a and b illustrate the 1H NMR and 13C NMR spectra of diamine monomer 3 in CDCl3, respectively. Assignments of each proton and carbon were assisted by the two-dimensional NMR spectra, as shown in Fig. 3, and these spectra agreed well with the proposed molecular structure of 3. In the 1H NMR spectrum, the primary aromatic amine protons appeared at 3.32 ppm and the protons in the aromatic rings (Ha to He) appeared at 6.20–8.04 ppm. Ha exhibited obvious deshielding due to the strong electron-withdrawing effect of the sulfone group and appeared at 8.04 ppm. In the 13C NMR spectrum, all the carbon-13 atoms resonated in the region of 115–155 ppm, and four quartets were observed because of the heteronuclear 13C–19F coupling. The large quartets centered at about 123.8 (C13) ppm are peculiar to CF3 carbons, and the one-bond C–F coupling constant in these cases is about 218.8 Hz. The CF3-attached carbons C9 also show clear quartets centered at 130.2 ppm, with a small coupling constant of about 25.8 Hz due to two-bond C–F coupling. Therefore, all the spectroscopic data provided clear evidence of the proposed structure of the target diamine monomer 3. Moreover, the elemental analysis values of diamine 3 were also in good agreement with the calculated values.
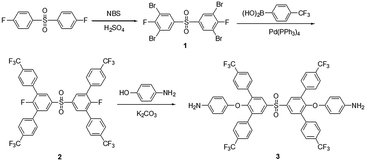 |
| Scheme 1 Synthesis of the aromatic diamine 3. | |
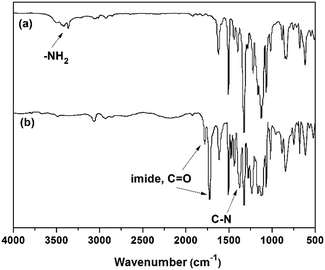 |
| Fig. 1 (a) FTIR spectrum of diamine 3 and (b) FTIR spectrum of PI 5a. | |
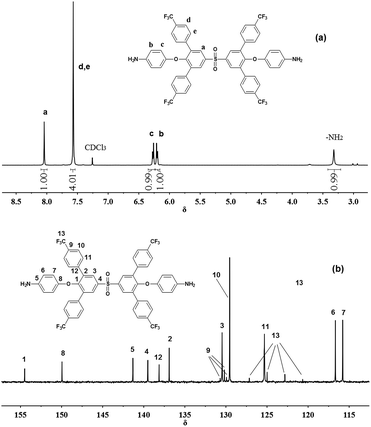 |
| Fig. 2 (a) 1H NMR spectrum of diamine 3 and (b) 13C NMR spectrum of diamine 3. | |
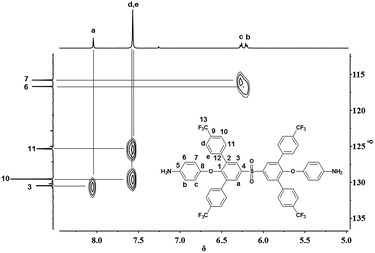 |
| Fig. 3 C13–H1 COSY spectrum of diamine monomer 3 in CDCl3. | |
Due to the introduction of multi-bulky pendant groups (trifluoromethylphenyl groups) at the ortho-position of the ether linkage of phenyl rings in diamine 3, the diamine monomer would possess a kink non-coplanar structure to maintain a minimum energy of conformation. As shown in the molecular model of diamine 3 (Fig. 4), the aromatic phenyl rings connected with the ether bonds displayed a large dihedral angle. Thus, the structure of diamine 3 was designed to achieve several desirable properties, such as good solubility, low dielectric constant, and high optical transparency, of the obtained PIs.
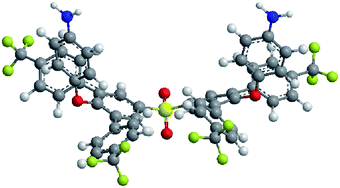 |
| Fig. 4 Minimum energy models of diamine 3, simulated by semiempirical computations using Cambridge Software. | |
Synthesis of polymers
The fluorinated PIs 5a–c could be prepared from diamine 3 and three available aromatic dianhydrides 4a–c (BPDA, BTDA, and ODPA) by one-step solution polycondensation, as shown in Scheme 2. The polymerization was carried out by treating stoichiometric amounts of diamine monomer 3 with aromatic dianhydrides at the concentration of about 10–20 wt% solids in m-cresol. In spite of the presence of multi-bulky trifluoromethylphenyl substituents, the diamine 3 was still sufficiently reactive to provide fibrous polymer resins when it was allowed to polymerize for about 12–20 h. The polymers were obtained in almost quantitative yields, and the inherent viscosities were in the range of 0.79–0.96 dL g−1 in NMP solution, as summarized in Table 1. According to the gel permeation chromatography (GPC) measurement results (Table 1), the Mn and polydispersities (Mw/Mn) values of the PIs dissolved in THF were in the range of 865
000–126
700 and 1.55–1.69, respectively. The molecular weight of PI 5b exhibited a slight decrease compared to that of PI 5a and 5c, which was mainly attributed to the activity of the dianhydride monomers.
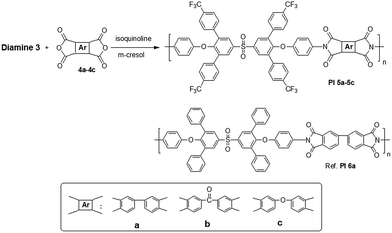 |
| Scheme 2 Synthesis of polyimides. | |
Table 1 Inherent viscositiesa and GPC molecular weights of the PIs
Polymers |
ηinh (dL g−1) |
GPC data |
Mn × 104 |
Mw × 104 |
PDI |
Measured at the concentration of 0.5 g dL−1 in NMP at 25 °C. |
5a |
0.96 |
12.67 |
19.64 |
1.55 |
5b |
0.79 |
8.65 |
14.18 |
1.64 |
5c |
0.83 |
10.38 |
17.54 |
1.69 |
The chemical structures of the polyimides were characterized by FTIR and 1H NMR analysis. All the polymers exhibited characteristic imide group absorptions around 1778 and 1733 cm−1 (typical of imide carbonyl asymmetrical and symmetrical stretch), 1385 (C–N stretch), and 1051 and 735 cm−1 (imide ring deformation), together with some strong absorption bands in the region of 1100–1300 cm−1 because of the C–O and C–F stretching. Moreover, they also showed the stretching peaks of the sulfone groups and phenyl rings (Ar–H and C–C) at about 1320, 3061, 1616, and 1509 cm−1. Representative FTIR and 1H NMR spectra of PI 5a are shown in Fig. 1b and 5, respectively. The assignments of each proton designated in the 1H NMR spectrum are in complete agreement with the proposed polymer structures. Herein, the sharp peaks at 3.32 ppm corresponding to the amine protons in the 1H NMR spectrum of diamine 3 (Fig. 2) completely disappeared, and new peaks appeared at 8.12–8.14 ppm, which corresponded to the protons in the dianhydride units. All the results indicated complete imidization between the diamine and dianhydrides.
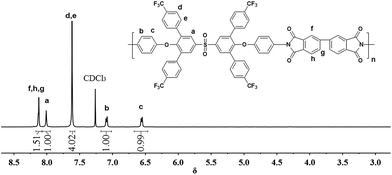 |
| Fig. 5 1H NMR spectrum of PI 5a. | |
Solubility and mechanical properties
Aromatic PIs generally show rather poor solubility upon full imidation because of their highly rigid macromolecular chains and crystallinity. Therefore, most PI films are prepared from poly(amic acid) followed by imidation at high temperature of curing.1,9 Unlike these polymers, the obtained PIs 5a–c had high solubility in most tested solvents and the maximum solubility could reach 20 wt% in NMP, CHCl3, and THF at room temperature (Table 2). The obtained PIs 5a–c had excellent solubility in NMP, DMAc, DMF, m-cresol, CHCl3, and CH2Cl2 at room temperature (Table 2). It was surprising that PI 5a, which was prepared from the very rigid dianhydride BPDA, showed a maximum solubility of even up to 20 wt% in NMP, CHCl3, and THF. Compared to the analogous PI 6a containing only four phenyl groups in the repeating units, PI 5a exhibited higher solubility due to the incorporation of trifluoromethyl groups. As was expected, PIs 5a–c also displayed improved solubility compared to some fluorinated polyimides derived from the trifluoromethyl-substituted bis(ether amine)s and corresponding dianhydrides, especially in some low-boiling-point solvents.17–20 From the view of preparing films or coatings at a relatively low processing temperature, good solubility in low-boiling-point solvents is a critical requirement for applications in advanced microelectronics manufacturing. High solubility was mainly attributed to the structural modification through the incorporation of multi-bulky trifluoromethylphenyl groups in the side chain, which rendered the polymer non-coplanar and also reduced the inter-chain interactions.
Table 2 Solubility behavior of the polymersa
Polymers |
NMP |
DMAc |
DMF |
DMSO |
CHCl3 |
CH2Cl2 |
THF |
Acetone |
Ethanol |
○, 200 mg sample dissolved in 1 mL solvent (20 wt%); ++, soluble at 10 wt%; +, soluble at 5 wt%; S, swelling; –, insoluble. The analogous PI 6a was prepared from 2,2′-bis[3,5-diphenyl-4-(4-aminophenoxy)phenyl]sulfone with BPDA via a one-step high-temperature polycondensation procedure in our laboratory. |
5a |
○ |
++ |
++ |
+ |
○ |
++ |
○ |
– |
– |
5b |
○ |
○ |
○ |
++ |
○ |
○ |
○ |
– |
– |
5c |
○ |
○ |
○ |
○ |
○ |
○ |
○ |
S |
– |
6ab |
++ |
+ |
+ |
+ |
++ |
+ |
++ |
– |
– |
Due to the excellent solubility and high-molecular weight, PI 5a–c could be easily processed into good quality and free standing films. The films were subjected to tensile tests, and the mechanical properties of the polyimides, including the tensile strength, tensile modulus as well as elongation at break, are presented in Table 3. They exhibited good mechanical properties with a tensile strength of 82–91 MPa, tensile modulus of 2.3–3.2 GPa, and elongation at break of 4.3–9.0%. Although the incorporation of multi-bulky trifluoromethylphenyl groups in PIs 5a–c leads to a non-coplanar structure in the macromolecular chain, they still show equivalent mechanical properties compared to some trifluoromethyl-substituted PIs derived from the corresponding dianhydrides.17–20 The results indicated that PIs 5a–c were tough enough for potential use in applications. The tensile modulus of the polyimides followed the order PI 5a > PI 5b > PI 5c, which mainly originated from different rigidities of the dianhydride units in the polymer structures. PI 5b exhibited slight decrease in its tensile strength and elongation at break compared to PI 5a and 5b, which were mainly due to its lower molecular weight.
Table 3 Mechanical properties of the PI films
Polymers |
Tensile strength (MPa) |
Elongation at break (%) |
Tensile modulus (GPa) |
5a |
91 |
8.6 |
3.2 |
5b |
82 |
4.3 |
2.6 |
5d |
86 |
9.0 |
2.3 |
Thermal properties
The thermal properties of the polymer films are important for their application in microelectronics and optoelectronics. The thermal properties of PIs 5a–c were determined using TGA and DSC analysis, and some characteristic data are listed in Table 4. PIs 5a–c exhibited high thermal stability with insignificant weight loss up to a temperature of approximately 550 °C under a nitrogen atmosphere. The 5% weight loss temperatures (Td5) under nitrogen and air atmospheres remained in the range of 551–561 °C and 515–520 °C, respectively. The TGA results showed that the thermal stability of these polyimides was comparable to that of other trifluoromethyl-substituted PIs derived from the corresponding dianhydrides.17–20 The representative TGA curves of PI 5a are shown in Fig. 6. The amount of carbonized residue (char yield) of these polymers under a nitrogen atmosphere was more than 55% at 800 °C. The high char yields can be ascribed to the high aromatic content of the polymers. Evidently, PIs 5a–c showed about 50–100 °C of Td5 increment compared to structural polyimides with multi-bulky alkyl groups.9–12
Table 4 Thermal properties of PIs 5a–c
Polymers |
Tga (°C) |
Td5b (°C) |
Char yieldc (%) |
In N2 |
In air |
Measured by DSC at the heating rate of 20 °C min−1. 5% weight loss temperature in TGA at 20 °C min−1 heating rate. Residual weight retention at 800 °C. |
5a |
281 |
561 |
520 |
56 |
5b |
265 |
551 |
515 |
58 |
5c |
259 |
554 |
517 |
57 |
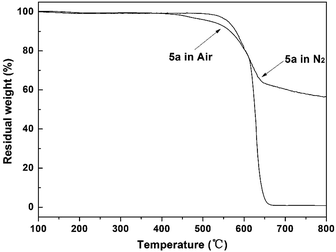 |
| Fig. 6 TGA curves of PI 5a at the heating rate of 20 °C min−1. | |
The glass transition temperatures Tg of PIs 5a–c were measured from DSC, and the values were in the range of 259–281 °C (Fig. 7). Generally, Tg is correlated with the stiffness and conformation of the polymer chain. The highest Tg value was obtained for PI 5a and it was attributed to the rigidity of the biphenyl moiety, whereas the lowest Tg value was obtained for PI 5c and this may have been because of the presence of flexible ether linkages. Moreover, compared to other trifluoromethyl-substituted PIs,17–20 the Tg values of PIs 5a–c were slightly lower because of the internal plasticization effect of the multi-bulky pendant groups. A large window between the glass transition temperature and the polymer degradation temperature also provides an opportunity for these polyimides to be melt-processed or compression molded.
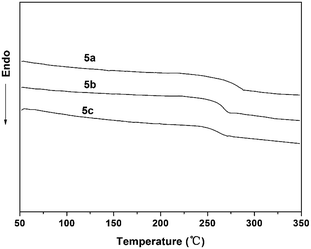 |
| Fig. 7 DSC traces of PIs 5a–c at the heating rate of 20 °C min−1. | |
Optical transparency, dielectric constants, and water absorption
Commercial PI films often show a deep color (yellow or reddish yellow) and low optical transparency in the ultraviolet-visible region due to the strongly aromatic conjugation and intermolecular charge transfer complex. Unlike the commercial PI films, these fluorinated PI films were light in color and displayed high transparency. Fig. 8 is a comparative image of PI 5a and Kapton, and the ultraviolet-visible spectra of the obtained PIs are shown in Fig. 9. Their cut-off wavelength (λcutoff) was in the range of 327–343 nm, and the transparency at 450 nm (T450) was higher than 80% (Table 5). PI 5a revealed a better optical transparency than the analogous PI 6a containing only four phenyl groups. Furthermore, PI 5a–c films exhibited lower λcutoff (20–50 nm decrease) and better optical transparency compared to other fluorinated PIs derived from the corresponding dianhydrides.17–20 The improved optical properties were mainly attributed to the structural modification of the polymer main chain. The incorporation of high non-coplanar diamine units with bulky multi-pendant groups could effectively reduce the aromatic conjugation and intermolecular charge transfer complex along the polymer backbone.
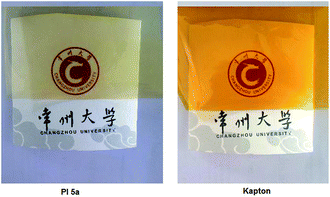 |
| Fig. 8 A comparative image of PI 5a and Kapton. | |
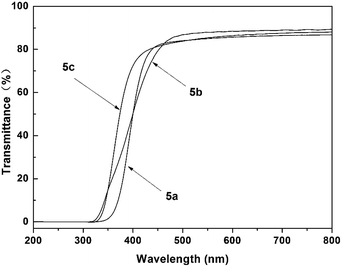 |
| Fig. 9 UV-vis spectra of the PI 5a–c films. | |
Table 5 Optical transparency, dielectric constants, and water absorption of the PI films
Polymers |
Optical properties |
Dielectric constants |
Water absorption (%) |
λcutoff (nm) |
T450 (%) |
1 kHz |
1 MHz |
5a |
343 |
82 |
2.80 |
2.69 |
0.59 |
5b |
327 |
80 |
2.97 |
2.85 |
0.68 |
5c |
329 |
81 |
2.88 |
2.77 |
0.65 |
6b |
357 |
78 |
3.17 |
3.05 |
0.98 |
Kapton |
— |
— |
3.54 |
3.48 |
2.1 |
Dielectric properties of the polymer films are vital to their application in microelectronics. Fig. 10 depicts the dielectric constant as a function of frequency, ranging from 1 kHz to 1 MHz at room temperature. PIs 5a–c revealed low dielectric constants and the values were in the range of 2.80–2.97 at 1 kHz and in the range of 2.69–2.85 at 1 MHz, which were much lower than that of commercial Kapton film under the same testing condition (Table 5). Moreover, the dielectric constants of PIs 5a–c were also lower than those of the analogous PI 6a, which did not contain four trifluoromethyl groups, and some trifluoromethyl-substituted polyimides derived from the corresponding dianhydrides.17–20 The effective reduction of the dielectric constants was mainly attributed to the introduction of multi-pendant trifluoromethylphenyl groups, which increased the free volume and loosened the polymer packing, subsequently reducing their dielectric constants. On the other hand, the strong electronegativity of fluorine results in very low polarizability of the C–F bonds, which could also decrease the dielectric constant. Low water absorption is also one of the key requirements for microelectronic materials, which ensures that the film maintains a stable dielectric performance. The water absorption of PI 5a–c films is listed in Table 5, and they are in the range of 0.59–0.68%, which are much lower than those of the analogous PI 6a (0.98%) and the standard Kapton film (2.1%) due to the high content of the hydrophobic trifluoromethyl groups.
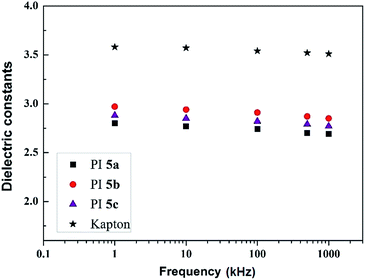 |
| Fig. 10 Dielectric constant of PIs 5a–c depending on electric frequency. | |
Conclusions
A new aromatic diamine monomer containing four pendant trifluoromethylphenyl groups was successfully synthesized from readily available reagents. A new family of fluorinated polyimides with high molecular weights was prepared from the newly synthesized diamine with various aromatic dianhydrides by a one-step chemical imidization method. The incorporation of multi-bulky pendant fluorinated groups and large non-coplanar structures brought about a great improvement in their properties. The obtained polyimides exhibited high solubility in many organic solvents and could be solution cast into flexible and strong films. They also had high thermal stability, good optical transparency, and low dielectric constants and water absorption. Compared to some trifluoromethyl-substituted polyimides, these polyimides with multi-bulky pendant groups possessed better solubility and lower dielectric constants and cut-off wavelength. As a result, these polyimides could be considered as promising processable high performance film materials for applications in microelectronic and optical devices. This study could provide a potential strategy for the preparation of high-performance fluorinated polyimides.
Acknowledgements
This work was supported by the Natural Science Foundation of Jiangsu Province (BK20141173), the Natural Science Foundation of the Jiangsu Higher Education Institutions (No. 15KJB430003), the Six Talent Peaks Project of Jiangsu Province (XCL-078), the Opening Project of State Key Laboratory for Modification of Chemical Fibers and Polymer Materials (LK1420 and LK1520), and a Project funded by the Priority Academic Program Development of Jiangsu Higher Education Institutions.
Notes and references
- M. X. Ding, Prog. Polym. Sci., 2007, 32, 623 CrossRef CAS.
- Z. H. Yang, Y. Chen and Q. H. Wang, Polymer, 2016, 88, 19 CrossRef CAS.
- J. Zhao, L. Peng, Y. L. Zhu, Y. J. Song, L. J. Wang and Y. Z. Shen, Polymer, 2016, 91, 118 CrossRef CAS.
- N. Mushtaq, G. F. Chen, L. R. Sidra, Y. Liu and X. Z. Fang, Polym. Chem., 2016, 7, 7427 RSC.
- J. H. Wu, W. C. Chen and G. S. Liou, Polym. Chem., 2016, 7, 1569 RSC.
- T. Maegawa, O. Miyashita, Y. Irie, H. Imoto and K. Naka, RSC Adv., 2016, 6, 31751 RSC.
- X. W. Peng, W. H. Xu, L. L. Chen, Y. C. Ding, T. R. Xiong, S. L. Chen and H. Q. Hou, React. Funct. Polym., 2016, 106, 93 CrossRef CAS.
- G. Maier, Prog. Polym. Sci., 2001, 26, 3 CrossRef CAS.
- Y. T. Chern, J. Y. Tsai and J. J. Wang, J. Polym. Sci., Part A: Polym. Chem., 2009, 47, 2443 CrossRef CAS.
- C. Y. Wang, X. Y. Zhao, G. Li and J. M. Jiang, Polym. Degrad. Stab., 2009, 94, 1526 CrossRef CAS.
- C. H. Lin, S. L. Chang and P. W. Cheng, J. Polym. Sci., Part A: Polym. Chem., 2011, 49, 1331 CrossRef CAS.
- G. L. Yang, R. Zhang, H. H. Huang, L. X. Liu, L. Wang and Y. M. Chen, RSC Adv., 2015, 5, 67574 RSC.
- S. A. Sydlik, Z. Chen and T. M. Swager, Macromolecules, 2011, 44, 976 CrossRef CAS.
- Y. Rogan, L. Starannikova, V. Ryzhikh, Y. Yampolskii, P. Bernardo, F. Bazzarelli, J. C. Jansen and N. B. McKeown, Polym. Chem., 2013, 4, 3813 RSC.
- W. X. Chen, Z. X. Zhou, T. T. Yang, R. X. Bei, Y. Zhang, S. W. Liu, Z. Chi, X. D. Chen and J. R. Xu, React. Funct. Polym., 2016, 108, 71 CrossRef CAS.
- M. G. Dhara and S. Banerjee, Prog. Polym. Sci., 2010, 35, 1022 CrossRef CAS.
- C. P. Yang and Y. Y. Su, J. Polym. Sci., Part A: Polym. Chem., 2004, 42, 222 CrossRef CAS.
- S. H. Hsiao, C. P. Yang and S. C. Huang, J. Polym. Sci., Part A: Polym. Chem., 2004, 42, 2377 CrossRef CAS.
- C. P. Yang, Y. Y. Su and K. L. Wu, J. Polym. Sci. Part A: Polym. Chem., 2004, 42, 5424 CrossRef CAS.
- S. H. Hsiao, W. J. Guo, C. L. Chung and W. T. Chen, Eur. Polym. J., 2010, 46, 1878 CrossRef CAS.
- Y. Liu, Y. Xing, Y. H. Zhang, S. W. Guan, H. B. Zhang, Y. Wang, Y. P. Wang and Z. H. Jiang, J. Polym. Sci., Part A: Polym. Chem., 2010, 48, 3281 CrossRef CAS.
- Y. Li, H. Xu, X. Tao, K. J. Qian, S. Fu and Y. Z. Shen, J. Mater. Chem, 2011, 21, 1810–1821 RSC.
- H. Y. Yao, Y. H. Zhang, K. Y. You, Y. Liu, Y. Song, S. Y. Liu and S. W. Guan, React. Funct.
Polym., 2014, 82, 58 CrossRef CAS.
- E. Cakmakci, A. Gungora and A. C. Goren, J. Fluorine Chem., 2016, 186, 66 CrossRef CAS.
- C. Y. Wang, D. W. Shin, S. Y. Lee, N. R. Kang, Y. M. Lee and D. M. Guiver, J. Membr. Sci., 2012, 405–406, 68 CrossRef CAS.
|
This journal is © The Royal Society of Chemistry 2017 |
Click here to see how this site uses Cookies. View our privacy policy here.