DOI:
10.1039/C7RA02175E
(Paper)
RSC Adv., 2017,
7, 25528-25534
A turn-on green channel Zn2+ sensor and the resulting zinc(II) complex as a red channel HPO42− ion sensor: a new approach†
Received
21st February 2017
, Accepted 14th April 2017
First published on 12th May 2017
Abstract
A newly synthesized and spectroscopically characterized non-fluorescent organic moiety (L′H) selectively sensed Zn2+ ions based on a chelation-enhanced fluorescence (CHEF) process at the λem of 520 nm through the formation of a new dinuclear zinc(II) complex (1). Upon the addition of Zn2+ ions to the solution of L′H in dimethyl sulphoxide at 25 °C, a systematic enhancement of fluorescence was observed, which was not affected by the presence of competitive ions. The reaction of L′H with Zn2+ ions led to the formation of a dinuclear zinc(II) complex, featuring a new in situ formed macrocyclic ligand (L), which was isolated in pure form and then characterized. The formulation of 1 was established by spectroscopic tools along with a detailed structural analysis carried out using single crystal X-ray crystallography. In addition, the complex 1 also behaved as a red-shifted, HPO42− ion-selective chemosensor at the λem of 595 nm based on a displacement approach in dimethyl sulphoxide. Interestingly, 1 showed remarkable sensitivity towards HPO42− ions via fluorescence modulation of the dinuclear zinc(II) complex (1) compared with the other anions examined herein.
Introduction
Designing highly sensitive and specific sensors for ionic species is of great interest because of biological and environmental applications of these sensors.1–3 These ions are vital because of their sustainable role in the biological processes of living beings. Zinc ion (Zn2+) is the second most abundant metal ion in the human body, and the cellular biochemistry of Zn2+ is diverse and far ranging.4 Moreover, misregulation of Zn2+ is also implicated in human health disorders. It is believed that lack of zinc ions can result in an increased risk of several disorders, such as stunted growth, mental retardation, and digestive dysfunction, because majority of biological zinc ions are tightly sequestered by proteins.5 Additionally, the presence of excess free zinc in certain cells may be related to severe neurological disorders such as Alzheimer's and Parkinson's disease.6a,b Therefore, it is necessary to obtain insight into the vital roles of Zn2+ ions in biological processes, resulting in great demand for the design and development of efficient systems that can selectively and sensitively detect Zn2+ in living systems. Several analytical methods including UV-vis-spectroscopy,6c potentiometry,6d and flame atomic absorption spectrometry6e have played a vital role in the detection of Zn2+. A variety of fluorescent sensors for Zn2+ have been reported based on various fluorophores,7–9 and continuous efforts have been dedicated to improve the effectiveness of Zn2+ sensors.
The development of selective receptors for phosphate anions and derivatives, such as HPO42−, H2PO4−, pyrophosphate (PPi, P2O74−), adenosine triphosphate (ATP), adenosine diphosphate (ADP), CTP3, IP3, and phosphoproteins,10 has been of particular interest because of the vital roles that these chemical species play in a range of life processes. These processes involve essential events such as energy storage, signal transduction, and gene construction. Especially, PPi is a biologically important target because it is the product of ATP hydrolysis under cellular conditions.11 Similarly, ATP is also a very important sensing target because it is known as a universal energy source and is also involved in many enzymatic reactions inside living organisms.11 Therefore, the development of a quick and sensitive method for phosphate anion detection is of great importance. Recently, metal-based receptors, particularly Zn(II) complexes, for fluorescence sensing of H2PO4− ions, pyrophosphate, and phosphate12 have been reported; however, a dinuclear zinc(II) complex as a HPO42− ion sensor has not been reported to date.
Due to high sensitivity, rapid response rate, and the relatively low-cost of fluorescence systems, significant attention has also been paid to developing fluorescent chemosensors capable of recognizing and sensing both cations and anions.13 In most cases, enhancement or the turn-on of fluorescence towards one species and the resulting complex acting as a quencher or turn-off for another species at the same emission wavelength, or vice versa, have been reported.14 In some cases, a change in solvent has played a key role in evaluating a sensor for two ions at different emission wavelengths.15 Herein, an organic moiety selectively sensed Zn2+ ions with green fluorescence (λem: 520 nm) at a concentration as low as 27.80 nM, and the resulting zinc(II) complex (1) effectively detected HPO42− ions with red fluorescence (λem: 595 nm) in the same solvent, DMSO, on excitation at the same wavelength (λex: 440 nm). To the best of our knowledge, to date, this type of an organic moiety and the resulting zinc(II) complex have not been explored as sensors based on the enhancement of fluorescence at different emission wavelengths through a significant amount of a red-shift of ca. 75 nm.
Herein, we present a new fluorescent and colorimetric chemosensor, 4-methyl-2,6-bis-[(5-phenyl-1H-pyrazol-3-ylimino)-methyl]-phenol (L′H), for the selective sensing of Zn2+ ions and the dinuclear zinc(II) complex of a new in situ formed macrocyclic type ligand (L); this complex resulted from the reaction of L′H with Zn2+ ions, which formulated Zn2L (1) that is selective for HPO42− anions in a DMSO solution. The L′H selectively senses Zn2+ ions based on a chelation-enhanced fluorescence (CHEF) process, whereas the structurally characterised zinc(II) complex (1) performs as a red-shifted, HPO42− ion selective chemosensor based on a displacement approach in dimethyl sulphoxide. The complex 1 showed selective UV-vis and fluorescence responses for the biologically relevant HPO42− anion in DMSO with a low detection limit even in the presence of several other anions.
Experimental
Synthesis of 4-methyl-2,6-bis-[(5-phenyl-1H-pyrazol-3-ylimino)-methyl]-phenol (L′H)
A solution of 3-amino-5-phenylpyrazole (1.59 g, 10.0 mmol) in absolute methanol (10 mL) was added dropwise to a stirred solution of 2,6-diformyl-4-methyl-phenol (0.82 g, 5.0 mmol) in the same solvent (20 mL). The resulting solution was gently refluxed under magnetic stirring for ca. 6 h. The yellow powder precipitate that formed was filtered off, washed several times with absolute methanol, dried under vacuum, and characterized as compound L′H.
L′H. C27H22N6O. Calcd C, 72.63; H, 4.97; N, 18.82; O, 3.58; anal. found: C 72.34, H 4.39, N 18.95. Mp 292 ± 2 °C. ESI-MS: [L + H+], m/z, 447.1939 (obs. with 100% abundance) [(calcd: m/z, 447.198; where L′H = molecular weight of organic moiety (L′H))]; 1H NMR (DMSO-d6, 400 MHz, δ ppm): 2.32 (s, 3H), 6.72–6.77 (s, 2H), 7.23–7.43 (m, 8H), 7.73–7.96 (m, 4H), 9.18 (s, 2H), 10.40 (s, 1H) and 13.42 (broad, 2H). 13C NMR (DMSO-d6): 162.87, 159.86, 150.05, 144.39, 140.03, 137.83, 129.85, 129.48, 125.98, 124.18, 121.27, 94.91 and 20.87. IR (KBr, cm−1): νOH: 3411.46, νCH
N: 1561.08, νNH: 1455.53, 1426.74. Yield, 97%.
Synthesis of dinuclear zinc(II) complex (1)
To the dimethylformamide (DMF) solution of L′H (446.0 mg, 1.0 mmol), a water solution of zinc nitrate hexahydrate (149.00 mg, 0.50 mmol) was very slowly added under vigorous stirring. Then, the reaction mixture was stirred at ambient temperature for another 6.0 h. The resulting solution was kept aside for slow evaporation at room temperature. After a few days, a red-coloured crystalline solid was obtained. Single crystals suitable for X-ray analysis were obtained from the solution of the crude product in DMF–H2O on slow evaporation.
[Zn2L]·3H2O. C90H72N18O7Zn2. Anal. found: C, 66.15; H, 4.53; N, 15.76; calc.: C, 66.30; H, 4.33; N, 15.46. IR (cm−1): νNH = 3427.45; νCH
N = 1577.07. 1H NMR (500 MHz, DMSO-d6): 10.18 (s, 1H); 8.85 (d, 1H; j = 50.5 Hz); 8.70 (s, 3H); 7.48 (m, 9H); 7.40 (m, 11H); 7.20 (m, 24H); 6.88 (b, 3H); 6.55 (s, 2H); 5.76 (s, 1H); 5.56 (b, 3H); 2.27 (s, 8H). ESI-MS (in methanol): [M + 2H]2+, m/z, 798.12 (100%) [(calcd: m/z, 798.21; where M = molecular weight of C90H66N18O4Zn2)] yield, 63%.
X-ray crystallography
Data collection of compound 1 was performed at the X-ray diffraction beamline (XRD1) of the Elettra Sincrotrone Trieste (Italy), with a Pilatus 2M image plate detector. The complete dataset was obtained at 100 K (nitrogen stream supplied by an Oxford Cryostream 700) with a monochromatic wavelength of 0.700 Å through the rotating crystal method. The crystals were dipped in N-paratone and mounted on the goniometer head with a nylon loop. The diffraction data were indexed, integrated, and scaled using XDS.16 The structure was solved by direct methods using SIR2014
17 and subsequent Fourier analysis, and refinement with the full-matrix least-squares method based on F2 was performed with SHELXL.18 Anisotropic thermal motion was allowed for all the non-H atoms. Hydrogen atoms were placed at the calculated positions, except those of the coordinated water molecules. The void in the unit cell (18.5%) was taken into account using the Squeeze program (Platon) since the solvent molecules could not be suitably modelled and refined. Graphics were drawn using the Cameron program.19 The dinuclear zinc(II) complex (1) crystallized in the monoclinic space group Pc. Crystal data and details are tabulated in Table S1,† and some selected bond distances and angles are listed in Table S2.†
General method of UV-vis and fluorescence titration
For UV-vis and fluorescence titrations, stock solutions of L′H and dinuclear zinc(II) complex (1) were prepared in DMSO and a stock solution of Zn2+ and HPO42− was prepared in HPLC water at 25 °C. Fluorescence measurements were carried out using a 2.5 nm x 2.5 nm slit width. To the 100 μL (5 μM) stock solution of L′H, 0–300 μL (0–15 μM) Zn2+ solution was gradually added, and the total volume was adjusted to 2.0 mL by adding the required amount of DMSO to obtain the absorbance and fluorescence spectra. Similarly, a HPO42− solution (0–150 μL) (0–75 μM) was gradually added to the 50 μL (25 μM) stock solution of the dinuclear zinc(II) complex (1), and the total volume was adjusted to 2 mL by the addition of DMSO. All the spectral data were acquired after 10 minutes of mixing to obtain the optimized spectra.
Result and discussion
Synthesis and structural characterisation
The organic moiety 4-methyl-2,6-bis-[(5-phenyl-1H-pyrazol-3-ylimino)-methyl]-phenol (L′H) was synthesized by condensing a methanolic solution of 3-amino-5-phenylpyrazole with 2,6-diformyl-4-methyl-phenol in a 2
:
1 ratio (Scheme 1). The formulation of L′H, as shown in Scheme 1, was established by physicochemical and spectroscopic tools. The peaks obtained in the 1H NMR spectrum of L′H were assigned, and these were in agreement with the structural formula of this ligand in solution (Fig. S1†). The ESI mass spectrum of the compound in methanol showed a peak at m/z 447.1939 with 100% abundance assignable to [L′H + H+] (calculated value at m/z 447.198), where L′H = molecular weight of the organic moiety (Fig. S2†). The IR spectrum of L′H showed the characteristic stretching of O–H, N–H, and C
N bonds (Fig. S3†), and the characteristic peaks were observed in the 13C NMR spectrum (Fig. S4†).
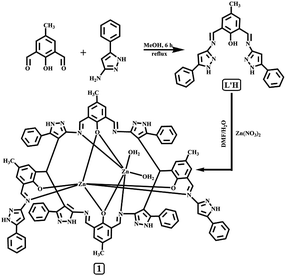 |
| Scheme 1 Synthetic strategy of L′H and Zn2L-complex (1). | |
The dinuclear zinc(II) complex (1) was isolated in the solid state from the reaction mixture of L′H with zinc nitrate hexahydrate in a 2
:
1 molar ratio in a DMF/water medium at room temperature under stirring condition. Upon reaction of L′H with Zn2+ ions, a new in situ macrocyclic type ligand (L) was formed and red-colored single crystals of Zn2L·H2O were obtained on slow evaporation. The well-resolved ESI mass spectrum (Fig. S5†), IR spectrum (Fig. S6†), and 1H NMR spectrum (Fig. S7†) of the dinuclear zinc(II) complex (1) support the formulation and the structure established by single crystal X-ray crystallographic analysis. Of the two zinc ions, Zn1 exhibited an octahedral coordination sphere realized through two phenolato oxygen and two imine nitrogen atoms, completing its coordination geometry with two aqua ligands. Zn2 presented a highly distorted octahedral environment by being chelated by two oxygen and two imine nitrogen donors (Fig. 1).
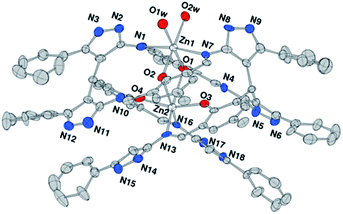 |
| Fig. 1 ORTEP drawing (ellipsoids at 30% probability) of the dinuclear complex Zn2L (H atoms omitted for clarity). | |
UV-vis spectroscopy studies of L′H
The UV-vis absorption spectrum of L′H obtained in DMSO solution exhibited intense absorption bands at energy levels below 400 nm, corresponding to π → π* (275 nm, ε = 2.1 × 105 L mol−1 cm−1; 315 nm, ε = 1.61 × 105 L mol−1 cm−1) and n → π* (380 nm, ε = 2.4 × 104 L mol−1 cm−1) transitions. To evaluate the binding affinity of L′H towards Zn2+ ions, the UV-vis spectral changes upon the addition of Zn2+ ions to the solution of L′H in DMSO were investigated. The incremental addition of Zn2+ ions led to a gradual decrease in the absorbance along with the appearance of a lower-energy metal-to-ligand charge-transfer (MLCT) band at around 440 nm. The presence of an isosbestic point at 410 nm indicated the existence of an equilibrium between two species: L′H and the resulting dinuclear zinc(II) complex (1) (Fig. 2).
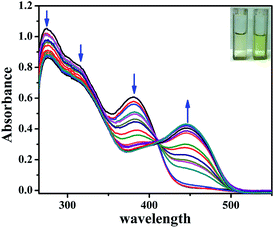 |
| Fig. 2 UV-vis titration spectra of L′H (5 μM) with Zn2+ ions (0–15 μM) in DMSO solution at 25 °C with naked eye visual color change of L′H only and Zn(II) complex (1) [in inset]. | |
To verify the potential of the probe L′H in the intracellular atmosphere, another inspection of the probe was carried out to check the interferences of other competitive ions. Metal ion selectivity assays were performed without changing other experimental conditions. On the addition of an excess of 10 equivalents of relevant metal ions (i.e., Cr3+, Mn2+, Fe3+, Co2+, Ni2+, Cu2+, Cd2+, Hg2+, Na+, K+, Ca2+, Mg2+, Al3+, and Pb2+), no significant change was observed in the UV-vis spectral pattern.
Fluorescence studies of L′H
The organic probe L′H exhibited a very feeble emission intensity at 520 nm (λex: 440 nm). Upon incremental addition of Zn2+ ions (0–15 μM), the fluorescence intensity steadily and significantly increased by about ∼11-fold at 520 nm (Fig. 3). This spectral characteristic for the addition of Zn2+ ions was supported by the shift from colourless to fluorescent green. To check the saturation time of the resulting emission, the time-dependent profile for the fluorescent detection of Zn2+ ions (Fig. S8†) was also obtained under the same conditions, and it was determined to be 15 min. From the fluorimetric titration curve, a plot of the fluorescence intensity vs. concentration of Zn2+ ions is depicted in Fig. S9.†
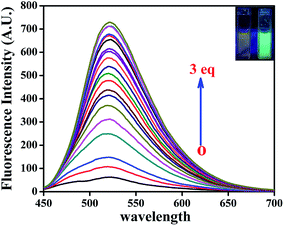 |
| Fig. 3 Fluorescence titration of L′H (5 μM) with the incremental addition of Zn2+ ions (0–15 μM) DMSO solution with naked eye fluorescence color change L′H only and zinc(II) complex (1) [in inset]. | |
The emission intensity increased due to addition of Zn2+ ions (0–15 μM) to the solution of L′H (5 μM) with an enhancement of the fluorescence quantum yield20 by ca. three times (Φ = 0.157) in DMSO, which was calculated by integrating the area under the fluorescence curves using the following equation:
where
A is the area under the fluorescence spectral curve, OD is the optical density of the compound at the excitation wavelength 440 nm, and
η is the refractive index of the solvent used. The standard used for the measurement of fluorescence quantum yield was fluorescein (
Φ = 0.79 in a 0.1 M NaOH solution).
Selectivity
The selectivity study of the organic moiety L′H towards various cations was carried out using transition metal ions (Cr3+, Mn2+, Fe3+, Co2+, Ni2+, Cu2+, Zn2+, Cd2+, and Hg2+), alkali and alkaline earth metal ions (Na+, K+, Ca2+, and Mg2+), and some other metal ions (Al3+ and Pb2+) (Fig. S10†), having a concentration 50 times that of the ligand. The organic moiety (L′H) had a remarkable specificity and selectivity towards Zn2+ ions over the other metal ions examined herein. Interestingly, the introduction of other metal ions caused the emission intensity to be either weakened or unchanged. In the presence of a 10 equivalent excess of various tested ions together with L′H and Zn2+ ions, no adverse effect on the intensity was observed (Fig. S11†).
Job's plot from fluorescence experiments
A series of solutions containing L′H and Zn2+ ions were prepared such that the total concentration of the sample solution remained constant (20 μM) in all the sets. The mole fractions of Zn2+ ions were varied from 0.1 to 0.8. The emission intensity (for L′H) at 520 nm was plotted against the mole fraction of [Zn2+] ions. From a Job's plot analysis (Fig. S12†), it was revealed that the maximum emission was at 2
:
1 ratio. These data indicate that the complex species in solution should form a 2
:
1 complex with Zn2+ ions, in accordance with the single crystal observations.
Effect of pH
A pH study was performed for the probe L′H in the absence and presence of Zn2+ ions over the pH range of 4.0–11.0 (Fig. S13†).
Detection limit calculation
The detection limit was calculated from the calibration curve based on the fluorescence enhancement at 520 nm (Fig. 4) focusing on the lower concentration region of Zn2+ ions. From the slope of the curve (S) and the standard deviation of seven replicate measurements of the zero level (σzero), the detection limit was estimated using the equation 3σ/S.21 The data obtained from this graph indicated that this probe can effectively detect Zn2+ ion at very low concentrations up to 27.80 nM.
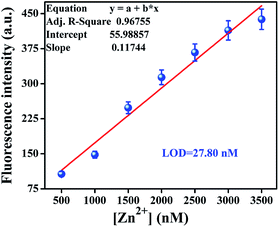 |
| Fig. 4 Calibration curve in the nanomolar range to calculate the LOD of Zn2+ ions by L′H in DMSO solution. | |
Spectroscopic studies of dinuclear zinc(II) complex (1)
Absorption study. The UV-vis absorption spectrum of 1, obtained in the DMSO solution, exhibited intense absorption-bands at the energy levels at 440 nm due to metal-to-ligand charge-transfer (MLCT) transitions. To find the binding affinity of 1 towards HPO42−, the spectral changes by the addition of HPO42− ions to the DMSO solution of 1 were investigated. The incremental addition of HPO42− ions to 1 led to a decrease in the intensity of the band at 432 nm with an appearance of new bands at around 315, 365, and 495 nm (Fig. 5).
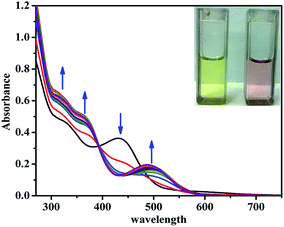 |
| Fig. 5 UV-vis titration spectra of 1 (25 μM) with HPO42− ions (0–75 μM) in DMSO solution at 25 °C. Naked eye visual color change of 1 only and 1 in presence of HPO42− ions [inset]. | |
Emission study. The displacement of Zn2+ ions from the complex (1) by HPO42− ions was studied from the emission spectral change of the titration curve, as shown in Fig. 6. For the emission study, DMSO solution was continuously titrated with an increasing concentration of HPO42− ions. The spectra show the generation of a red-shifted emission band with a maximum at ∼595 nm. The continuous increase in the HPO42− ions concentration (Fig. S14†) led to a concomitant enhancement of the emission band with a maximum at ∼595 nm. The resultant solution was kept undisturbed for 15 min, and then, the emission spectrum was obtained considering the time-dependent profile for the fluorescent detection of HPO42− ions, as shown in Fig. S15.† The emission intensity increased due to addition of HPO42− ions (0–75 μM) to the solution of 1 (25 μM), with the enhancement of fluorescence quantum yield by ca. two times (Φ = 0.3421) in DMSO, which was calculated by integrating the area under the fluorescence curves.
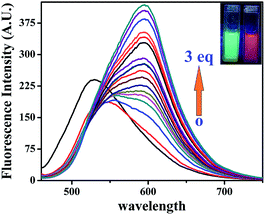 |
| Fig. 6 Fluorescence titration of 1 (25 μM) with the incremental addition of HPO42− ions (0, 2.5, 5, 10, 15, 20, 25, 30, 35, 40, 45, 50, 55, 60, 65, 70, and 75 μM) in DMSO solution (λex = 440 nm). Naked eye fluorescence color change 1 only and 1 in presence of HPO42− ions [inset]. | |
To examine the efficacy of the resulting dinuclear zinc(II) complex (1), herein, the rupture of the dinuclear zinc(II) complex (1) to restore L′H and the subsequent change in the spectral properties of the dinuclear zinc(II) complex (1) in DMSO were studied towards different anions (SCN−, PO43−, MO42−, CN−, F−, Cl−, Br−, I−, HAsO42−, AcO−, HPO42−, H2AsO4−, SO42−, H2PO4−, HSO4−, NO3−, CO32−, HCO3−, ClO4−, and P2O74− (1 equiv., as sodium/potassium salts)). The binding studies exhibited a fast, sensitive, and distinct response towards HPO42− ions in comparison to the other anions (Fig. S16†). In the fluorescence spectrum, upon addition of HPO42− ions, the emission band at 520 nm was quenched, and a new red-shifted band at 595 nm appeared. This result indicated the weakening of the existing dinuclear zinc(II) complex (1) bond due to the interaction of HPO42− ions with Zn2+ ions to form Zn(HPO4) species, insoluble in this medium. Almost no interference was observed for the detection of HPO42− anions even in the presence of other anions, having 50 times higher concentration (Fig. S16†).
The detection limit was calculated from the calibration curve based on the fluorescence enhancement at 595 nm (Fig. S17†) focusing on the lower concentration region of HPO42− anions. From the slope of the curve (S) and the standard deviation of seven replicate measurements of the zero level (σzero), the detection limit was estimated using the equation 3σ/S.21 The data obtained from this graph indicates that this probe can effectively detect HPO42− anions at very low concentrations up to 3.12 × 10−7 M.
The emission spectra of L′H, L′H + Zn2+ ion (1), and 1 + HPO42− ions were also obtained with the increasing solvent polarity, as shown in Fig. S18.† From this study, it was revealed that the polarity of the solvent had almost no effect on the emission wavelength (λem) but had an effect on the emission intensity, which was highest in DMSO in both cases.
The turn on green fluorescence (λem = 520 nm) was due to the formation of a new dinuclear zinc(II) complex (1), which was based on chelation-enhanced fluorescence (CHEF) process, and the fluorescence arose due to the formation of a dinuclear zinc(II) complex of a newly in situ formed macrocyclic ligand (L) during the reaction of L′H with Zn2+ ions.
The displacement mechanistic pathway in the complex (1) by hydrogen phosphate anion was established by UV-vis and fluorescence spectra (Fig. S19†), ESI mass spectra (Fig. S20†), and 1H NMR spectra (Fig. S21†). We obtained a new absorption peak at ca. 495 nm due to the addition of HPO42− anions to the zinc(II) complex (1); moreover, a similar type of peak was obtained at ca. 495 nm in the case of L′H in the presence of a base, a signature of the anionic species L′ (viz. Fig. S13†) formed with a high fluorescence quantum yield (Φ = 0.300). The findings from these studies indicate that the appearance of the red fluorescence (λem = 595 nm) of 1 upon the gradual addition of HPO42− anions was due to the formation of a deprotonated form of L′H. On the basis of these spectroscopic observations, a plausible sensing pathway is shown in Scheme 2.
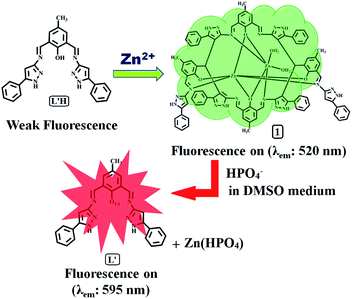 |
| Scheme 2 Probable mechanism for Zn2+ sensing by L′H and HPO42− ions sensing by the Zn(II) complex (1). | |
TCSPC experiment
To strengthen the proposed mechanism, the fluorescence average life time measurement of 1 was performed, and it was found to be 0.56 ns at λem = 595 nm. The average lifetime of the formed species, due to the addition of HPO42− ions to the solution of 1, increased to 2.25 ns at λem = 595 nm (viz. 1
:
HPO42−; 1
:
1 in Fig. 7). According to the equations:22 τ−1 = kr + knr and kr = Φf/τ, the radiative rate constant kr and total nonradiative rate constant knr of dinuclear zinc(II) complex (1) in the absence and presence of HPO42− ions are listed in Table S3.† The data suggests that the kr/knr ratio was reasonably enhanced due to the reasonable decrease of knr in support of the increased in fluorescence in the presence of HPO42− ions at λem = 595 nm.
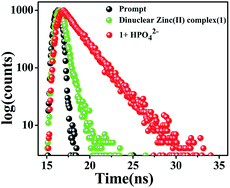 |
| Fig. 7 Time-resolved fluorescence decay of dinuclear zinc(II) complex (1) and 1 in the presence of HPO42− ions (at λem = 595 nm) in DMSO using a nano-LED at 470 nm as the light source. | |
Conclusions
A new non-fluorescent Schiff base-type organic probe (L′H) was synthesized and spectroscopically characterised. L′H selectively sensed Zn2+ ions based on a chelation-enhanced fluorescence (CHEF) process at a λem of 520 nm (green fluorescence) through the formation of a new dinuclear zinc(II) complex (1) with almost no interference of competitive ions. A red-colored solid dinuclear zinc(II) complex of a new in situ formed macrocyclic-type ligand (L), formulated as Zn2L, (1) was isolated from the reaction mixture of L′H with Zn2+ ions and was characterized by spectroscopic tools along with detailed structural analyses using single crystal X-ray crystallography. Interestingly, complex (1) also selectively sensed HPO42− anions with a red fluorescence (λem: 595 nm) in the same solvent, DMSO, on excitation at the same wavelength (λex: 440 nm).
Acknowledgements
The financial assistance received from Department of Science and Technology, Govt. of West Bengal (DST, GoWB, vide project no. 698 (Sanc.)/ST/P/S & T/15-G/2015) is gratefully acknowledged. S. Lohar is thankful to UGC, New Delhi, India for the fellowship.
Notes and references
-
(a) D. Li, L. Liu and W. H. Li, ACS Chem. Biol., 2015, 10, 1054 CrossRef CAS PubMed;
(b) Y. Ding, Y. Tang, W. Zhu and Y. Xie, Chem. Soc. Rev., 2015, 44, 1101 RSC;
(c) Z. Guo, G. H. Kim, I. Shin and J. Yoon, Biomaterial, 2012, 33, 7818 CrossRef CAS PubMed;
(d) K. Tayade, S. K. Sahoo, B. Bondhopadhyay, V. K. Bhardwaj, N. Singh, A. Basu, R. Bendre and A. Kuwar, Biosens. Bioelectron., 2014, 61, 429 CrossRef CAS PubMed;
(e) H. Tapiero and K. D. Tew, Biomed. Pharmacother., 2003, 61, 399 CrossRef.
-
(a) J. Yoon, S. K. Kim, N. J. Singh and K. S. Kim, Chem. Soc. Rev., 2006, 35, 355 RSC;
(b) R. Martínez-Manez and F. Sancanon, Chem. Rev., 2003, 103, 4419 CrossRef PubMed;
(c) J. F. Callan, A. P. de Silva and D. C. Magri, Tetrahedron, 2005, 61, 8551 CrossRef CAS.
-
(a) L. Fabbrizzi, M. Licchelli, G. Rabaioli and A. Taglietti, Coord. Chem. Rev., 2000, 205, 85 CrossRef CAS;
(b) P. A. Gale, Acc. Chem. Res., 2006, 39, 465 CrossRef CAS PubMed.
-
(a) A. I. Bush, W. H. Pettingell, G. Multhaup, M. Paradis, J. P. Vonsattel, J. F. Gusella, K. Beyreuther, C. L. Masters and R. E. Tanzi, Science, 1994, 265, 1464 CAS;
(b) C. J. Frederickson, J. Y. Koh and A. I. Bush, Nat. Rev. Neurosci., 2005, 6, 449 CrossRef CAS PubMed;
(c) D. D. Mott, M. Benveniste and R. J. Dingledine, J. Neurosci., 2008, 28, 1659 CrossRef CAS PubMed;
(d) J. M. Berg and Y. Shi, Science, 1996, 271, 1081 CAS.
- A. Krężel and W. Maret, J. Biol. Inorg Chem., 2006, 11, 1049 CrossRef PubMed.
-
(a) A. I. Bush, Trends Neurosci., 2003, 26, 207 CrossRef CAS PubMed;
(b) D. Noy, I. Solomonov, O. Sinkevich, T. Arad, K. Kjaer and I. Sagi, J. Am. Chem. Soc., 2008, 130, 1376 CrossRef CAS PubMed;
(c) C. V. Banks and R. E. Bisque, Anal. Chem., 1957, 29, 522 CrossRef CAS;
(d) A. R. Fakhari, M. Shamsipur and K. H. Ghanbari, Anal. Chim. Acta, 2002, 460, 177 CrossRef CAS;
(e) Q. Li, X. H. Zhao, Q. Z. Lv and G. G. Liu, Sep. Purif. Technol., 2007, 55, 76 CrossRef CAS.
-
(a) K. K. Upadhyay, A. Kumar, J. Zhao and R. K. Mishra, Talanta, 2010, 81, 714 CrossRef CAS PubMed;
(b) J. F. Zhang, S. Kim, J. H. Han, S. J. Lee and J. S. Kim, Org. Lett., 2011, 13, 5294 CrossRef CAS PubMed;
(c) Y. Xu, J. Meng, L. X. Meng, Y. Dong, Y. X. Cheng and C. J. Zhu, Chem.–Eur. J., 2010, 16, 12898 CrossRef CAS PubMed;
(d) Q. H. You, P. S. Chan, W. H. Chan, N. K. Mak and R. N. S. Wong, RSC Adv., 2012, 2, 11078 RSC;
(e) H. Y. Lin, P. Y. Cheng, C. F. Wan and A. T. Wu, Analyst, 2012, 137, 4415 RSC.
-
(a) S. Comby, S. A. Tuck, L. K. Truman, O. Kotova and T. Gunnlaugsson, Inorg. Chem., 2012, 51, 10158 CrossRef CAS PubMed;
(b) J. Jia, Q. C. Xu, R. C. Li, X. Tang, Y. F. He, M. Y. Zhang, Y. Zhang and G. W. Xing, Org. Biomol. Chem., 2012, 10, 6279 RSC;
(c) X. Meng, S. Wang, Y. Li, M. Zhu and Q. Guo, Chem. Commun., 2012, 48, 4196 RSC;
(d) T. Mukherjee, J. C. Pessoa, A. Kumar and A. R. Sarkar, Dalton Trans., 2012, 41, 5260 RSC;
(e) S. H. Mashraqui, R. Betkar, S. Ghorpade, S. Tripathi and S. Britto, Sens. Actuators, B, 2012, 174, 299 CrossRef CAS;
(f) U. C. Saha, B. Chattopadhyay, K. Dhara, S. K. Mandal, S. Sarkar, A. R. Khuda-Bukhsh, M. Mukherjee, M. Helliwell and P. Chattopadhyay, Inorg. Chem., 2011, 50, 1213 CrossRef CAS PubMed.
-
(a) G. Mandal, M. Darragh, Y. A. Wang and C. D. Heyes, Chem. Commun., 2013, 49, 624 RSC;
(b) G. Sivaraman, T. Anand and D. Chellappa, Analyst, 2012, 137, 5881 RSC;
(c) L. J. Liang, S. J. Zhen, X. J. Zhao and C. Z. Huang, Analyst, 2012, 137, 5291 RSC;
(d) P. G. Sutariya, N. R. Modi, A. Pandya, B. K. Joshi, K. V. Joshi and S. K. Menon, Analyst, 2012, 137, 5491 RSC;
(e) Y. W. Choi, G. J. Park, Y. J. Na, H. Y. Jo, S. A. Lee, G. R. You and C. Kim, Sens. Actuators, B, 2014, 194, 343 CrossRef CAS;
(f) E. J. Song, H. Kim, I. H. Hwang, K. B. Kim, A. R. Kim, I. Noh and C. Kim, Sens. Actuators, B, 2014, 195, 36 CrossRef CAS;
(g) G. J. Park, H. Kim, J. J. Lee, Y. S. Kim, S. Y. Lee, S. Lee, I. Noh and C. Kim, Sens. Actuators, B, 2015, 215, 568 CrossRef CAS;
(h) J. J. Lee, S. A. Lee, H. Kim, L. T. Nguyen, I. Noh and C. Kim, RSC Adv., 2015, 5, 41905 RSC;
(i) A. K. Bhanja, C. Patra, S. Mondal, D. Ojha, D. Chattopadhyay and C. Sinha, RSC Adv., 2015, 5, 48997 RSC;
(j) S. Dey, A. Roy, G. P. Maiti, S. K. Mandal, P. Banerjee and P. Roy, New J. Chem., 2016, 40, 1365 RSC;
(k) H. Y. Lin, T. Y. Chen, C. K. Liu and A. T. Wu, Luminescence, 2016, 31, 236 CrossRef CAS PubMed.
- For HPO42− sensing, see:
(a) M. S. Han and D. H. Kim, Angew. Chem., Int. Ed., 2002, 41, 3963 CrossRef; for H2PO4− sensing, see: ref. 10b and c
(b) S. Sen, M. Mukherjee, K. Chakrabarty, I. Hauli, S. K. Mukhopadhyay and P. Chattopadhyay, Org. Biomol. Chem., 2013, 11, 1537 RSC;
(c) D. Zhang, J. R. Cochrane, A. Martinez and G. Gao, RSC Adv., 2014, 4, 29735 RSC; for PPi sensing, see: ref. 10d
(d) S. K. Kim, D. H. Lee, J. I. Hong and J. Yoon, Acc. Chem. Res., 2009, 42, 23 CrossRef CAS PubMed; for ATP sensing, see: ref. 10e
(e) A. Ojida, H. Nonaka, Y. Miyahara, S. I. Tamaru, K. Sada and I. Hamachi, Angew. Chem., Int. Ed., 2006, 118, 5644 CrossRef; for CTP3 sensing, see: ref. 10f
(f) K. Nikura and E. V. Anslyn, J. Am. Chem. Soc., 1998, 120, 8533 CrossRef; for IP3 sensing, see: ref. 10g
(g) D. J. Oh and K. H. Ahn, Org. Lett., 2008, 10, 3539 CrossRef CAS PubMed; for phosphoprotein sensing, see: ref. 10h and i
(h) S. Aoki, M. Zulkefeli, M. Shiro, M. Kohsako, K. Takeda and E. Kimura, J. Am. Chem. Soc., 2005, 127, 9129 CrossRef CAS PubMed;
(i) T. Anai, E. Nakata, Y. Koshi, A. Ojida and I. Hamachi, J. Am. Chem. Soc., 2007, 129, 6232 CrossRef CAS PubMed; PPi polymer sensing, see: ref. 10j
(j) D. Aldakov and P. Anzenbacher Jr, J. Am. Chem. Soc., 2004, 126, 4752 CrossRef CAS PubMed; for recent articles on PPi and ATP sensing, see: ref. 10k and l
(k) X. Huang, Z. Guo, W. Zhu, Y. Xie and H. Tian, Chem. Commun., 2008, 5143 RSC;
(l) A. Ojida, I. Takashima, T. Kohira, H. Nonaka and I. Hamachi, J. Am. Chem. Soc., 2008, 130, 12095 CrossRef CAS PubMed.
-
(a) C. P. Mathews and K. E. van Holde, Biochemistry, Benjamin/Cummings Publishing Company, Inc., Redwood City, CA, 1990 Search PubMed;
(b) P. Nyren, Anal. Biochem., 1987, 167, 235 CrossRef CAS PubMed;
(c) T. Tabary and L. Ju, J. Immunol. Methods, 1992, 156, 55 CrossRef CAS PubMed.
-
(a) X. l. Ni, X. Zeng, C. Redshaw and T. Yamato, J. Org. Chem., 2011, 76, 5696 CrossRef CAS PubMed;
(b) L. M. Mesquita, V. André, C. V. Esteves, T. Palmeira, M. N. Berberan-Santos, P. Mateus and R. Delgado, Inorg. Chem., 2016, 55, 2212 CrossRef CAS PubMed;
(c) I. Ravikumar and P. Ghosh, Inorg. Chem., 2011, 50, 4229 CrossRef CAS PubMed;
(d) S. Khatua, S. H. Choi, J. Lee, K. Kim, Y. Do and D. G. Churchill, Inorg. Chem., 2009, 48, 2993 CrossRef CAS PubMed.
-
(a) Z. Guo, S. Park, J. Yoon and I. Shin, Chem. Soc. Rev., 2014, 43, 16 RSC;
(b) S. Pal, S. Lohar, M. Mukherjee, P. Chattopadhyay and K. Dhara, Chem. Commun., 2016, 52, 13706 RSC.
-
(a) X. Lou, D. Ou, Q. Li and Z. Li, Chem. Commun., 2012, 48, 8462 RSC;
(b) B. Sen, M. Mukherjee, S. Pal, K. Dhara, S. K. Mandal, A. R. Khuda-Bukhsh and P. Chattopadhyay, RSC Adv., 2014, 4, 14919 RSC;
(c) D. Sarkar, A. K. Pramanik and T. K. Mondal, RSC Adv., 2014, 4, 25341 RSC;
(d) X. Lou, H. Mu, R. Gong, E. Fu, J. Qin and Z. Li, Analyst, 2011, 136, 684 RSC;
(e) B. Sen, M. Mukherjee, S. Banerjee, S. Pal and P. Chattopadhyay, Dalton Trans., 2015, 44, 8708 RSC;
(f) V. Bhalla, V. Vij, M. Kumar, P. R. Sharma and T. Kaur, Org. Lett., 2012, 14, 1012 CrossRef CAS PubMed.
-
(a) L. He, C. Liu and J. H. Xin, Sens. Actuators, B, 2015, 213, 181 CrossRef CAS;
(b) E. Hao, T. Meng, M. Zhang, W. Pang, Y. Zhou and L. Jiao, J. Phys. Chem. A, 2011, 115, 8234 CrossRef CAS PubMed.
- W. Kabsch, Acta Crystallogr., Sect. D: Biol. Crystallogr., 2010, 66, 125 CrossRef CAS PubMed.
- M. C. Burla, R. Caliandro, B. Carrozzini, G. L. Cascarano, C. Cuocci, C. Giacovazzo, M. Mallamo, A. Mazzone and G. J. Polidori, Appl. Crystallogr., 2015, 48, 306 CrossRef CAS.
- G. M. Sheldrick, Acta Crystallogr., Sect. A: Found. Crystallogr., 2008, 64, 112 CrossRef CAS PubMed.
- D. M. Watkin, L. Pearce and C. K. Prout, Cameron – A Molecular Graphics Package, Chemical Crystallography Laboratory, University of Oxford, 1993 Search PubMed.
- W. H. Melhuish, J. Phys. Chem., 1961, 65, 229 CrossRef CAS.
-
(a) S. Pal, M. Mukherjee, B. Sen, S. Lohar and P. Chattopadhyay, RSC Adv., 2014, 4, 21608 RSC;
(b) S. Lohar, S. Pal, B. Sen, M. Mukherjee, S. Banerjee and P. Chattopadhyay, Anal. Chem., 2014, 86, 11357 CrossRef CAS PubMed.
- N. J. Turro, Modern Molecular Photochemistry, Benjamin/Cummings Publishing Co., Inc., Menlo Park, CA, 1978 Search PubMed.
Footnote |
† Electronic supplementary information (ESI) available: Materials and physical methods, tables, figures, characterization data, and some spectra. CCDC 1528839. CCDC no. for dinuclear zinc(II) complex (1). For ESI and crystallographic data in CIF or other electronic format see DOI: 10.1039/c7ra02175e |
|
This journal is © The Royal Society of Chemistry 2017 |
Click here to see how this site uses Cookies. View our privacy policy here.