DOI:
10.1039/C7RA09619D
(Paper)
RSC Adv., 2017,
7, 47520-47526
The effect of additional methyl on the magnetic relaxation and toroidal moment of Dy6 complex†
Received
30th August 2017
, Accepted 3rd October 2017
First published on 9th October 2017
Abstract
Two new μ4-O bridged hexanuclear lanthanide complexes, [Dy6L′4(μ4-O)(NO3)4]·4CH3OH (1) and [Sm6L′4(μ4-O)(CH3COO)4]·2CH3OH (2), have been assembled by using methyl modified Schiff-base ligand H3L′ (2,6-bis((2-hydroxypropylimino)methyl)-4-methylphenol). The complex 1 shares a similar μ4-O bridged Dy6 core as the parent Dy6-2, while coordination geometries and magnetic interactions are slightly changed upon the modulation of the ligand, which results in distinct single-molecule magnetic (SMM) and single-molecule toroic (SMT) properties.
Introduction
The design and construction of single-molecule magnets (SMMs) has attracted increasing attention since the discovery of SMM behaviour,1 because they have potential applications in processing and storing magnetic information at a molecular level.2–7 Thus a large number of SMMs, including 3d complexes, 3d/4f complexes and 4f complexes, have been reported. Thereinto, 4f-based complexes are particularly appealing due to the strong magnetic anisotropy of 4f ions, and a flurry of groundbreaking results have been produced.2,5,8–12 It is noteworthy that some cyclic Dy3,13–15 Dy4,16–18 and cyclic/local cyclic Dy6 complexes19–21 have exhibited SMM behaviours and toroidal moments that arise from a vortex arrangement of local magnetic moments of the individual Dy(III) centers. These systems are defined as single-molecule toroics (SMTs), which are promising candidates for future applications in quantum computing and information storage.5,22 Toroidal moment is generally influenced by the molecular symmetry, local magnetic moment as well as magnetic interactions. However, the study on magneto-structural relationships of SMTs is rare.16,21 Thus, it is of primary importance to design and construct a comprehensive (quasi)isostructural series of complexes to improve our knowledge of magneto-structural relationships.
In reality, most lanthanide SMMs have been synthesized by serendipitous approaches. Modulating the structure of complexes by design and choice of ligands is still a challenge. In general, one type of ligand affords a particular type of complexes possessing a certain nuclearity and topology.23–26 In this regard, we have been interested to investigate the magneto-structural relationships of SMTs with one typic ligand. Recently, we have successfully assembled a Dy6 SMTs with enhanced toroidal moment that was formed by linkage of two Dy3 units (the complex is defined as Dy6-2).27 To go a step further, we modified slightly the Schiff-base ligand by adding a methyl, and assembled two μ4-O bridged hexanuclear lanthanide clusters, [Dy6L′4(μ4-O)(NO3)4]·4CH3OH (1) and [Sm6L′4(μ4-O)(CH3COO)4]·2CH3OH (2). Though Dy6-2 and complex 1 have similar structures, the coordination geometries and magnetic interactions are changed upon the slight modulation of the ligand. The coordination environment of Dy(III) ions will influence the local tensor of anisotropy and crystal-field splitting. Thus they exhibit dissimilar magnetic behaviour. Complex 1 shows SMM behaviour with obvious quantum tunnelling of the magnetization (QTM) and SMT property with large conventional magnetization.
Experimental section
General
All starting materials were of A.R. Grade and were used as commercially obtained without further purification. 2,6-Diformyl-4-methylphenol (DFMP) were prepared according to a previously published method.28 The Schiff-base ligand H3L′ (2,6-bis((2-hydroxypropylimino)methyl)-4-methylphenol) (shown in Scheme 1) was prepared by the in situ condensation of DFMP and (DL)-1-amino-2-propanol in a 1
:
2 ratio in methanol.
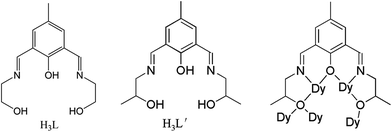 |
| Scheme 1 Ligands H3L and H3L′ and the coordination formation of H3L′. | |
Elemental analyses for C, H, and N were carried out on a Perkin-Elmer 2400 analyzer. Fourier transform IR spectra (4000–300 cm−1) were measured using KBr pellets by a Fourier transform infrared spectrometer Nicolet 6700. All magnetization data were recorded on a Quantum Design MPMS-XL7 SQUID magnetometer equipped with a 7 T magnet. The variable-temperature magnetization was measured with an external magnetic field of 1000 Oe in the temperature range of 1.9–300 K. The experimental magnetic susceptibility data are corrected for the diamagnetism estimated from Pascal's tables and sample holder calibration.
X-ray crystallography
Suitable single crystals of 1 and 2 were selected for single-crystal X-ray diffraction analysis. Crystallographic data were collected at 185(2) K on a Bruker ApexII CCD diffractometer with graphite monochromated Mo-Kα radiation (λ = 0.71073 Å). The structure was solved by direct methods and refined on F2 with full-matrix least-squares techniques using SHELXS-97 and SHELXL-97 programs.29,30 The locations of Dy(III) ion were easily determined, and O, N, and C atoms were subsequently determined from the difference Fourier maps. Anisotropic thermal parameters were assigned to all non-hydrogen atoms. The H atoms were introduced in calculated positions and refined with a fixed geometry with respect to their carrier atoms. CCDC 1566095 and 1566096 contain the supplementary crystallographic data for this paper.†
Synthesis of [Dy6L′4(μ4-O)(NO3)4]·4CH3OH (1)
A solution of Dy(NO3)3·6H2O (137.8 mg, 0.30 mmol) in 5 ml methanol was added in a solution of DFMP (0.15 mmol) and (DL)-1-amino-2-propanol (0.3 mmol) in 5 ml methanol in presence of triethylamine. The mixture was continual stirred at room temperature for 20 min. The resulting reaction mixture was sealed in an autoclave and maintained at 90 °C for 3 days, and then cooled slowly to room temperature to yield yellow single crystals suitable for X-ray diffraction. Yield: 33 mg, (27%, based on the metal). Elemental analysis (%) calcd for Dy6C64H92N12O29: C, 31.14, H, 3.76, N, 6.81; found C, 30.96, H, 3.67, N, 6.50. IR (KBr, cm−1): 2856 (w), 1646 (vs), 1549 (m), 1497 (m), 1452 (s), 1396 (m), 1368 (w), 1323 (s), 1281 (m), 1231 (m), 1082 (m), 1042 (s), 902 (w), 873 (w), 813 (m), 775 (m), 739 (w).
Synthesis of [Sm6L′4(μ4-O)(CH3COO)4]·2CH3OH (2)
A procedure similar to that for 1 was followed except that Dy(NO3)3·6H2O was replaced by Sm(CH3COO)3·H2O (0.3 mmol, 105.2 mg). Yield: 27 mg (23%, based on the metal salt). Elemental analysis (%) calcd for Sm6C70H94N8O23: C, 36.27; H, 4.09; N, 4.83. Found: C, 36.16; H, 3.97; N, 4.53. IR (KBr, cm−1): 2910 (w), 1644 (s), 1548 (vs), 1444 (s), 1395 (m), 1323 (m), 1231 (w), 1231 (w), 1138 (m), 1033 (s), 928 (w), 854 (w), 816 (w), 678 (w).
Results and discussion
To explore the effect of additional methyl groups on the lanthanide compound, the symmetrical Schiff-base H3L′ (Scheme 1) was formed by the in situ condensation of DFMP and (DL)-1-amino-2-propanol (1
:
2) in methanol. Similar to the procedure for Dy6-2, the reactions of in situ formed H3L′ with Dy(NO3)3·6H2O/Sm(CH3COO)3·H2O in the presence of triethylamine under solvothermal condition produce block-shaped crystals of [Dy6L′4(μ4-O)(NO3)4]·4CH3OH (1) and [Sm6L′4(μ4-O)(CH3COO)4]·2CH3OH (2). The structures of 1 and 2 were established by single crystal X-ray diffraction. The crystal data and structure refinement are summarised in Table 1.
Table 1 Crystal data and structure refinement for complexes 1 and 2
Compound |
1 |
2 |
Empirical formula |
Dy6C64H92N12O29 |
Sm6C70H94N8O23 |
Fw (g mol−1) |
2468.50 |
2317.63 |
Crystal system |
Triclinic |
Monoclinic |
Space group |
P![[1 with combining macron]](https://www.rsc.org/images/entities/char_0031_0304.gif) |
C2/c |
a (Å) |
12.4459(5) |
18.6094(11) |
b (Å) |
13.8185(5) |
17.218(1) |
c (Å) |
25.2852(10) |
25.3390(14) |
α (°) |
82.073(1) |
90 |
β (°) |
81.870(1) |
92.634(1) |
γ (°) |
75.400(1) |
90 |
V (Å3) |
4142.1(3) |
8110.5(8) |
Z, ρcalcd (Mg m−3) |
2, 1.979 |
4, 1.898 |
F(000), Rint |
2376, 0.0306 |
4504, 0.0744 |
R1, wR2 [I > 2σ(I)] |
0.0455, 0.1085 |
0.0495, 0.1146 |
R1, wR2 (all data) |
0.0750, 0.1271 |
0.0887, 0.1394 |
GOF |
1.006 |
1.008 |
As shown in Fig. 1, complex 1 crystallizes in the triclinic space group P
and consists of six Dy(III) ions, four (L′)3− ligands, one μ4-O anion and four NO3− anions. The core of 1, similar to the Dy6-2, consists of two [Dy3(μ3-O)2(μ2-O)2] triangular units linked by one μ4-O2− ion in an edge-to-edge arrangement. The triangular Dy3 units in 1 are more equilateral than that in Dy6-2 with Dy⋯Dy distances in range 3.4933(8)–3.4017(6) Å. The dihedral angle between the two Dy3 planes in 1 is 30.101(12)° that is slightly larger than that in Dy6-2 with 29.6568°.
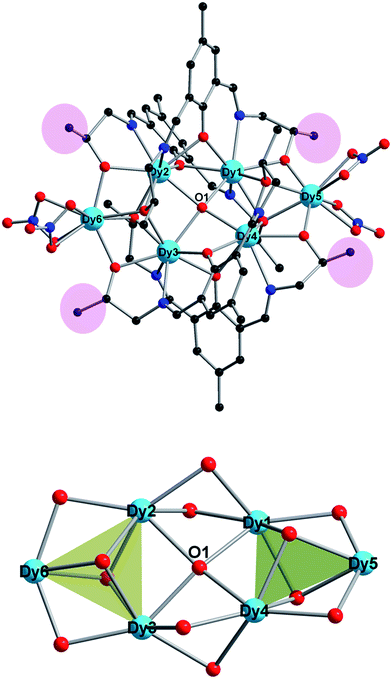 |
| Fig. 1 (Top) Structure of 1 with hydrogen atoms omitted for clarity. (Bottom) μ4-O bridged hexanuclear core. | |
It is notable that all donors in ligand H3L′ are coordinated, and each of the four (L′)3− binds five Dy(III) ions utilizing their tridentate pocket (O2N) along with bridging alkoxido oxygen (Scheme 1). This is different from some Schiff-base ligands that only coordinated partially.31 Two arms of alkoxido oxygens in ligands bridge distinctly: while one arm bridges two Dy(III) ions of one triangular edge, the other arm links three Dy(III) ions upon the other triangular unit. Additionally, the phenoxido oxygen bridges two Dy(III) ions from two triangular units. Therefore, alkoxido oxygens from four ligands fasten Dy3 triangles, one μ4-O anion and four phenoxido oxygens of ligands further strengthen the “Dy3 + Dy3” construction from center and periphery, respectively. This is the same with the reported Dy6-2. Such a robust edge-to-edge arrangement will enhance the toroidal magnetic moment of the molecule.
The coordination spheres of Dy5 and Dy6 ions are completed respectively by two nitrate ions, and all Dy(III) ions are eight-coordinate (Fig. 2). This is different from Dy6-2, where five Dy(III) centers are eight-coordinate and one Dy(III) ion is nine-coordinate. The exact geometries of all Dy(III) centers were determined by using the SHAPE 2.1
32,33 and the results are listed in Table S1.† For complex 1, Dy1/Dy3/Dy5/Dy6 centers are situated in distorted square antiprismatic geometries, and Dy2/Dy4 centers are situated in distorted triangular dodecahedron. For Dy6-2, Dy1/Dy3/Dy5 centers are situated in distorted square antiprismatic geometries, Dy2/Dy4 centers are situated in distorted triangular dodecahedron, and Dy6 ion is in mono-capped square antiprism. The changes probably have a great impact on the magnetic anisotropy and further the magnetic properties.34–37
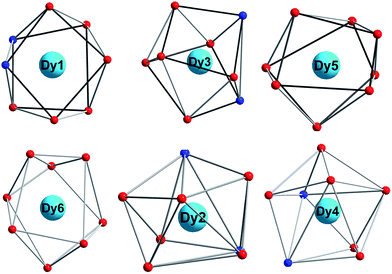 |
| Fig. 2 Coordination polyhedra (distorted square antiprism for Dy1, Dy3, Dy5 and Dy6; distorted triangular dodecahedron for Dy2 and Dy4) for six Dy3+ ions in 1. | |
In addition, the Dy–O bond lengths fall in the range of 2.235(6)–2.478(7) Å with the average bond of 2.37 Å, the Dy–N bond lengths fall in the range of 2.457(8)–2.490(8) Å with the average bond of 2.47 Å. Those average bonds in complex 1 are similar to that of Dy6-2 (average bonds are 2.48 and 2.38 Å for Dy–O and Dy–N, respectively). This indicates the metal–ligand interactions of complex 1 and Dy6-2 are similar.
In [Dy6L′4(μ4-O)(NO3)4] core, the four ligands are twisted along the C–N bonds, and each ligand straddles two triangles to coordinate five Dy(III) ions, resulting in formation of quadruple-stranded helicates (Fig. 3).38–40 Significantly, two stereoisomers of right- (Δ) and left-hand (Λ) configurations are in crystal, forming a racemic mixture. The packing arrangement of 1 reveals that Δ- and Λ-configuration helicates are alternate along the crystallographic a axis (Fig. 4).
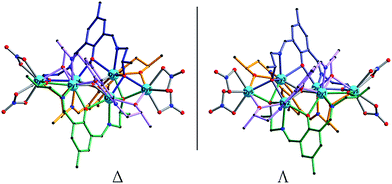 |
| Fig. 3 Two stereoisomers of right- (Δ) and left-hand (Λ) configurations in 1. | |
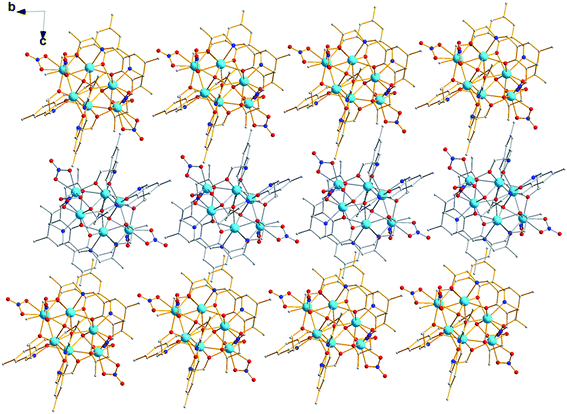 |
| Fig. 4 Crystal packing of 1 along the crystallographic a axis showing the stereoisomers. | |
Complex 2 crystallizes in the monoclinic space group C2/c, the asymmetric unit contains four Sm(III) ions, two (L′)3− ligands and two CH3COO− ions. The structure of 2 is essentially isomorphous to 1 (Fig. S1†), consists of a μ4-O2− ion linked two triangular units in an edge-to-edge arrangement, where Sm⋯Sm distances are in range of 3.4439(10)–3.6234(96) Å, the dihedral angle between the two Sm3 planes in 2 is 28.176° that is smaller than that in 1. In the asymmetric unit, Sm2 and Sm3 ions are eight-coordinate with 6O2N, and both display distorted triangular dodecahedral geometries based on SHAPE 2.1 (Fig. S2 and Table S2†). The coordination spheres of Sm1 and Sm4 ions are filled respectively by two CH3COO− ions, forming eight-coordinate Sm1 and Sm4 ions with 8O. The exact geometries of Sm1 and Sm4 ions are both distorted square antiprisms based on SHAPE 2.1. In addition, the Sm–O bond lengths fall in the range of 2.331(6)–2.506(8) Å, the Sm–N bond lengths fall in the range of 2.507(9)–2.552(9) Å.
In [Sm6L′4(μ4-O)(CH3COO)4] core, each of four ligands coordinates five Sm(III) ions and straddles two triangular units, producing formation of quadruple-stranded helicates (Fig. S3†). As shown in Fig. S4,† the two stereoisomers of Δ and Λ configurations form a racemic mixture, which can be seen in molecular structures and crystal packing.
Magnetic studies
The direct current (dc) susceptibility studies of complexes 1 and 2 were investigated under a 1000 Oe field in the temperature range 2–300 K (Fig. 5). At room temperature, the χMT value of complex 1 is 86.37 cm3 K mol−1. The value is in agreement with the expected theoretical value (85.02 cm3 K mol−1) for six free Dy(III) ions (6H15/2, S = 5/2, L = 5, g = 4/3, C = 14.17 cm3 K mol−1). On cooling, χMT decreases throughout the whole temperature range: firstly, χMT decreases monotonously down to 50 K, then decreases abruptly to 21.03 cm3 K mol−1 at 2.0 K. The thermal variation of the χMT indicates the progressive depopulation of the excited Stark sublevels of Dy(III) ions and/or weak molecular magnetic interactions.41,42 Actually, this profile of χMT is similar but higher to that of Dy6-2, and the minimum is larger than that of Dy6-2 (6.59 cm3 K mol−1 at 2.0 K), which indicates the weaker magnetic interaction in complex 1. The large minimum (21.03 cm3 K mol−1) also indicates the magnetic ground state of complex 1 is different from the net toroidal moment.16,20,43
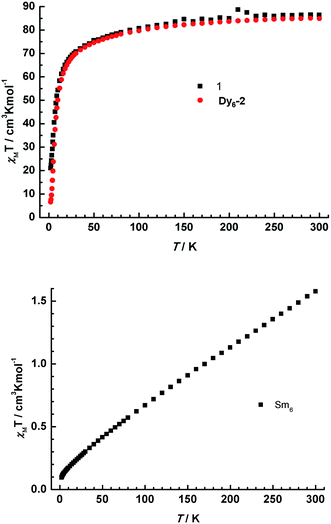 |
| Fig. 5 (Top) Plots of χMT vs. T for 1 (black square) and Dy6-2 (red circle) in a dc field of 1000 Oe (2–300 K); (bottom) plots of χMT vs. T for 2 in a dc field of 1000 Oe. | |
For complex 2, the χMT value (Fig. 5) observed at room temperature is 1.58 cm3 K mol−1, which is significantly larger than the theoretical one. This is attributed to the first or even the higher excited states (6H7/2, 6H9/2,…, 6H15/2) of the Sm(III) ion that can be populated obviously at room temperature.44 On cooling, the χMT value decreases continuously to a minimum of 0.10 cm3 K mol−1 at 2.0 K.
The field dependence of magnetization (M) was measured between 1.9 and 5 K. As shown in Fig. 6, it is obviously that M under variable field at 1.9 K shows a “S” shape, which is consistent with SMTs.22 The peak (d(M/Ms)/dH) vs. field suggests inflection around 12 kOe. The inflection is less evident than that in Dy6-2. M reaches a maximum value 33.83 μB at about 45 kOe, which is far lower than the expected saturation value of 60 μB for six noninteracting Dy(III) ions. This can be mostly attributed to crystal-field effects and the possible weak antiferromagnetic interactions that make the low-lying excited states accessible. Meanwhile, the non-superposition of the M vs. H/T data implies the presence of significant magnetic anisotropy. The static magnetic behaviours, including the dc susceptibility and the field dependence of M, reveal that the ground state of 1 could be toroidal magnetic moment and the conventional magnetic moment should be larger than that of Dy6-2.22
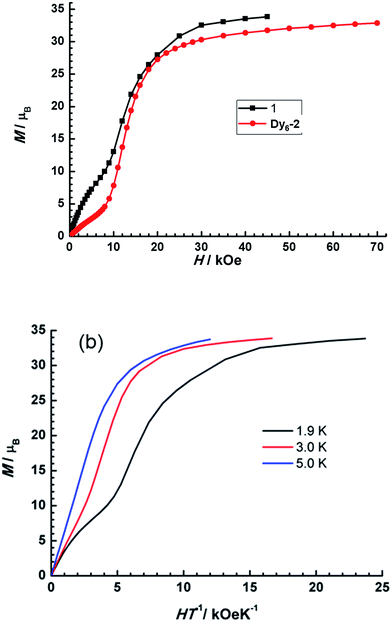 |
| Fig. 6 (Top) Field dependence of magnetization at 1.9 K for 1 and Dy6-2. (Bottom) M versus H/T plots for 1 at 1.9 K, 3 K and 5 K. | |
Furthermore, the anisotropy axes of complex 1 were calculated using the Magellan program45–47 based on an electrostatic model (Fig. 7 and Table S3†). The results indicate that complex 1 shows toroidal moment in both Dy3 units. This agrees with the magnetic properties. The ab initio calculations48–50 are planned to provide quantitative evaluation of the anisotropy axes and Dy–Dy coupling interactions.
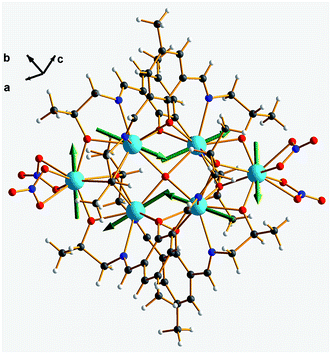 |
| Fig. 7 Ground-state magnetic anisotropy of complex 1. The green arrows represent the orientations of the anisotropic axes for each Dy(III) ion, as calculated by the electrostatic model. | |
To compare the magnetic relaxation been Dy6-2 and 1, the dynamics of the magnetization of 1 were investigated using alternating current (ac) susceptibility measurements (Fig. 8). Under zero dc field, the variable-temperature ac susceptibility of 1 shows the temperature dependence maximum, which signals the freezing of the spins by the anisotropy barrier and is typical for SMM behaviour. Both 1 and Dy6-2 show broad peak in the out-of-phase susceptibility, but the former appears at 7 K that is lower than that of Dy6-2 (at about 9 K). This indicates lower effective energy barrier for complex 1. Obviously, unlike the Dy6-2, the rise in the out-of-phase and in-phase susceptibility on cooling at low temperatures indicates the presence of QTM for 1, which may be due to the weak magnetic interaction that is too weak to suppress the QTM.51–53 This agrees well with dc magnetic susceptibility that indicates weaker magnetic interaction in complex 1.
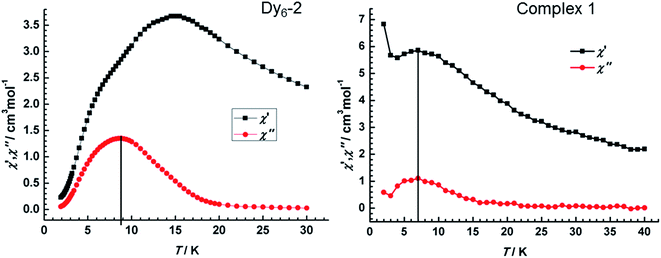 |
| Fig. 8 Temperature dependence of the out-of phase and in-phase ac susceptibility for Dy6-2 (left) and 1 (right) at 1000 Hz under zero dc field. | |
The reported isomorphous Dy6-2 displayed impressive dynamic magnetic properties with effective suppression of QTM and remarkable toroidal moment. However, obvious QTM is present and toroidal moment is less distinct in 1. Comparing the structure data of complex 1 and Dy6-2, they have the identical metal–ligand interaction, but distinctive coordination geometries mainly on Dy6 ion. For Dy6-2, Dy6 ion is nine-coordinate with a mono-capped square antiprism. While Dy6 center in complex 1 is eight-coordinate situated in distorted square antiprismatic geometries, which is due to the steric hindrance from additional methyl groups of ligands (Fig. 1). The changes in coordination geometries have a great impact on the orientation of the easy axes. Consequently, the toroidal moment retains in complex 1 but with a large conventional magnetization, SMM behaviour also is observed but with a non-ignorable QTM. It should be note that the weaker magnetic interaction in complex 1 could not suppress the QTM.
Conclusions
In conclusion, methyl modified Schiff-base ligand has been successfully used to synthesize two new hexanuclear lanthanide complexes, [Dy6L′4(μ4-O)(NO3)4]·4CH3OH (1) and [Sm6L′4(μ4-O)(CH3COO)4]·2CH3OH (2), under solvothermal condition. Single crystal X-ray diffraction studies revealed that complex 1 has a μ4-O bridged Dy6 core, μ4-O bridged two [Dy3(μ3-O)2(μ2-O)2] triangular units in an edge-to-edge arrangement, that is the same as the parent Dy6-2. While the coordination geometries especially on Dy6 site and magnetic interactions change, which result in distinct magnetic properties. Complex 1 shows SMM behaviour with a non-ignorable QTM and SMT behaviour with a large conventional magnetization. The work provides an efficient strategy to probe the magneto-structural relationships of SMTs, further to design the special SMTs and SMMs.
Conflicts of interest
There are no conflicts to declare.
Acknowledgements
This research work was supported by the National Natural Science Foundation of China (no. 21401034), the Natural Science Foundation of Heilongjiang Province (QC2015008), the Innovative Talents Training Plan for Young Undergraduates in Heilongjiang Province (UNPYSCT-2016074), and the Open Funds of the State Key Laboratory of Rare Earth Resource Utilization (RERU2017018).
References
- R. Sessoli, D. Gatteschi, A. Caneschi and M. A. Novak, Nature, 1993, 365, 141–143 CrossRef CAS.
- D. N. Woodruff, R. E. Winpenny and R. A. Layfield, Chem. Rev., 2013, 113, 5110–5148 CrossRef CAS PubMed.
- S. Sanvito, Chem. Soc. Rev., 2011, 40, 3336–3355 RSC.
- P. Zhang, L. Zhang and J. Tang, Dalton Trans., 2015, 44, 3923–3929 RSC.
- S. Liu, T. J. Cui, Q. Xu, D. Bao, L. Du, X. Wan, W. X. Tang, C. Ouyang, X. Y. Zhou, H. Yuan, H. F. Ma, W. X. Jiang, J. Han, W. Zhang and Q. Cheng, Light: Sci. Appl., 2016, 5, e16076 CrossRef CAS.
- M. Gu, X. Li and Y. Cao, Light: Sci. Appl., 2014, 3, e177 CrossRef CAS.
- N. Papasimakis, S. Thongrattanasiri, N. I. Zheludev and F. J. Garcia de Abajo, Light: Sci. Appl., 2013, 2, e78 CrossRef.
- P. Zhang, Y.-N. Guo and J. Tang, Coord. Chem. Rev., 2013, 257, 1728–1763 CrossRef CAS.
- Y.-S. Meng, S.-D. Jiang, B.-W. Wang and S. Gao, Acc. Chem. Res., 2016, 49, 2381–2389 CrossRef CAS PubMed.
- H. Wang, B.-W. Wang, Y. Bian, S. Gao and J. Jiang, Coord. Chem. Rev., 2016, 306, 195–216 CrossRef CAS.
- Y.-J. Liang, F. Liu, Y.-F. Chen, X.-J. Wang, K.-N. Sun and Z. Pan, Light: Sci. Appl., 2016, 5, e16124 CrossRef CAS.
- C. C. Lin, W.-T. Chen, C.-I. Chu, K.-W. Huang, C.-W. Yeh, B.-M. Cheng and R.-S. Liu, Light: Sci. Appl., 2016, 5, e16066 CrossRef CAS.
- L. Ungur, W. V. d. Heuvela and L. F. Chibotaru, New J. Chem., 2009, 33, 1224–1230 RSC.
- S. Xue, X.-H. Chen, L. Zhao, Y.-N. Guo and J. Tang, Inorg. Chem., 2012, 51, 13264–13270 CrossRef CAS PubMed.
- J. Tang, I. Hewitt, N. T. Madhu, G. Chastanet, W. Wernsdorfer, C. E. Anson, C. Benelli, R. Sessoli and A. K. Powell, Angew. Chem., Int. Ed., 2006, 45, 1729–1733 CrossRef CAS PubMed.
- J. F. Wu, S. Y. Lin, S. Shen, X. L. Li, L. Zhao, L. Zhang and J. K. Tang, Dalton Trans., 2017, 46, 1577–1584 RSC.
- C. Das, S. Vaidya, T. Gupta, J. M. Frost, M. Righi, E. K. Brechin, M. Affronte, G. Rajaraman and M. Shanmugam, Chem.–Eur. J., 2015, 21, 15639–15650 CrossRef CAS PubMed.
- S. Biswas, S. Das, T. Gupta, S. K. Singh, M. Pissas, G. Rajaraman and V. Chandrasekhar, Chem.–Eur. J., 2016, 22, 18532–18550 CrossRef CAS PubMed.
- S. K. Langley, B. Moubaraki, C. M. Forsyth, I. A. Gass and K. S. Murray, Dalton Trans., 2010, 39, 1705–1708 RSC.
- L. Ungur, S. K. Langley, T. N. Hooper, B. Moubaraki, E. K. Brechin, K. S. Murray and L. F. Chibotaru, J. Am. Chem. Soc., 2012, 134, 18554–18557 CrossRef CAS PubMed.
- X.-L. Li, J. Wu, J. Tang, B. Le Guennic, W. Shi and P. Cheng, Chem. Commun., 2016, 52, 9570–9573 RSC.
- L. Ungur, S.-Y. Lin, J. Tang and L. F. Chibotaru, Chem. Soc. Rev., 2014, 43, 6894–6905 RSC.
- L. Zhang, P. Zhang, L. Zhao, S.-Y. Lin, S. Xue, J. Tang and Z. Liu, Eur. J. Inorg. Chem., 2013, 2013, 1351–1357 CrossRef CAS.
- R. J. Blagg, L. Ungur, F. Tuna, J. Speak, P. Comar, D. Collison, W. Wernsdorfer, E. J. L. McInnes, L. F. Chibotaru and R. E. P. Winpenny, Nat. Chem., 2013, 5, 673–678 CrossRef CAS PubMed.
- J. Wu, L. Zhao, M. Guo and J. Tang, Chem. Commun., 2015, 51, 17317–17320 RSC.
- S. Biswas, S. Das, J. Acharya, V. Kumar, J. van Leusen, P. Kogerler, J. M. Herrera, E. Colacio and V. Chandrasekhar, Chem.–Eur. J., 2017, 23, 5154–5170 CrossRef CAS PubMed.
- S.-Y. Lin, W. Wernsdorfer, L. Ungur, A. K. Powell, Y.-N. Guo, J. Tang, L. Zhao, L. F. Chibotaru and H.-J. Zhang, Angew. Chem., Int. Ed., 2012, 51, 12767–12771 CrossRef CAS PubMed.
- R. R. Gagne, C. L. Spiro, T. J. Smith, C. A. Hamann, W. R. Thies and A. K. Shiemke, J. Am. Chem. Soc., 1981, 103, 4073–4081 CrossRef CAS.
- G. M. Sheldrick, SHELXS-97, Program for Crystal Structure Solution, University of Göttingen, Germany, 1997 Search PubMed.
- G. M. Sheldrick, SHELXL-97, Program for Crystal Structure Refinement, University of Göttingen, Germany, 1997 Search PubMed.
- S.-Y. Lin, C. Wang and Z. Xu, RSC Adv., 2016, 6, 103035–103041 RSC.
- E. C. Mazarakioti, J. Regier, L. Cunha-Silva, W. Wernsdorfer, M. Pilkington, J. K. Tang and T. C. Stamatatos, Inorg. Chem., 2017, 56, 3568–3578 CrossRef CAS PubMed.
- M. Llunell, D. Casanova, J. Girera, P. Alemany and S. Alvarez, SHAPE, version 2.1 Search PubMed.
- M. E. Boulon, G. Cucinotta, J. Luzon, C. Degl'innocenti, M. Perfetti, K. Bernot, G. Calvez, A. Caneschi and R. Sessoli, Angew. Chem., Int. Ed., 2013, 52, 350–354 CrossRef CAS PubMed.
- S.-Y. Lin, J. F. Wu, C. Wang, L. Zhao and J. K. Tang, Eur. J. Inorg. Chem., 2015, 2015, 5488–5494 CrossRef CAS.
- Y. Bi, Y.-N. Guo, L. Zhao, Y. Guo, S.-Y. Lin, S.-D. Jiang, J. Tang, B.-W. Wang and S. Gao, Chem.–Eur. J., 2011, 17, 12476–12481 CrossRef CAS PubMed.
- G.-J. Chen, Y.-N. Guo, J.-L. Tian, J. Tang, W. Gu, X. Liu, S.-P. Yan, P. Cheng and D.-Z. Liao, Chem.–Eur. J., 2012, 18, 2484–2487 CrossRef CAS PubMed.
- S.-Y. Lin, G.-F. Xu, L. Zhao, Y.-N. Guo, Y. Guo and J. Tang, Dalton Trans., 2011, 40, 8213–8217 RSC.
- A. Stephenson and M. D. Ward, Chem. Commun., 2012, 48, 3605–3607 RSC.
- L. Zhang, P. Zhang, L. Zhao, J. Wu, M. Guo and J. Tang, Dalton Trans., 2016, 45, 10556–10562 RSC.
- D. Aguila, L. A. Barrios, V. Velasco, L. Arnedo, N. Aliaga-Alcalde, M. Menelaou, S. J. Teat, O. Roubeau, F. Luis and G. Aromi, Chem.–Eur. J., 2013, 19, 5881–5891 CrossRef CAS PubMed.
- S.-Y. Lin, X.-L. Li, H. Ke and Z. Xu, CrystEngComm, 2015, 17, 9167–9174 RSC.
- P.-H. Guo, J.-L. Liu, Z.-M. Zhang, L. Ungur, L. F. Chibotaru, J.-D. Leng, F.-S. Guo and M.-L. Tong, Inorg. Chem., 2012, 51, 1233–1235 CrossRef CAS PubMed.
- Molecular Magnetism, ed. O. Kahn, VCH Publishers, New York, 1993 Search PubMed.
- N. F. Chilton, D. Collison, E. J. L. McInnes, R. E. P. Winpenny and A. Soncini, Nat. Commun., 2013, 4, 2551 Search PubMed.
- S.-D. Jiang and S.-X. Qin, Inorg. Chem. Front., 2015, 2, 613–619 RSC.
- Y. Peng, V. Mereacre, A. Baniodeh, Y. Lan, M. Schlageter, G. E. Kostakis and A. K. Powell, Inorg. Chem., 2016, 55, 68–74 CrossRef CAS PubMed.
- L. Ungur and L. F. Chibotaru, Inorg. Chem., 2016, 55, 10043–10056 CrossRef CAS PubMed.
- L. F. Chibotaru and L. Ungur, in Programs SINGLE_ANISO and POLY_ANISO, University of Leuven, 2006 Search PubMed.
- L. Ungur and L. F. Chibotaru, Phys. Chem. Chem. Phys., 2011, 13, 20086–20090 RSC.
- Y.-N. Guo, X.-H. Chen, S. Xue and J. Tang, Inorg. Chem., 2011, 50, 9705–9713 CrossRef CAS PubMed.
- Y.-N. Guo, G.-F. Xu, W. Wernsdorfer, L. Ungur, Y. Guo, J. Tang, H.-J. Zhang, L. F. Chibotaru and A. K. Powell, J. Am. Chem. Soc., 2011, 133, 11948–11951 CrossRef CAS PubMed.
- J. D. Rinehart, M. Fang, W. J. Evans and J. R. Long, Nat. Chem., 2011, 3, 538–542 CrossRef CAS PubMed.
Footnote |
† Electronic supplementary information (ESI) available. CCDC 1566095 and 1566096. For ESI and crystallographic data in CIF or other electronic format see DOI: 10.1039/c7ra09619d |
|
This journal is © The Royal Society of Chemistry 2017 |
Click here to see how this site uses Cookies. View our privacy policy here.