DOI:
10.1039/C7RA09687A
(Paper)
RSC Adv., 2017,
7, 53653-53657
Computational design of enhanced photocatalytic activity of two-dimensional cadmium iodide†
Received
31st August 2017
, Accepted 1st November 2017
First published on 22nd November 2017
Abstract
The recent synthesis of two-dimensional cadmium iodide (CdI2) opens up the questions of its properties and potential applications in optoelectronic and photovoltaic devices. Using a first-principles design approach, the electronic structure of 2D CdI2 is determined. The calculated results of the band gaps and band edges demonstrated that CdI2 is a suitable photocatalyst for water splitting. Monolayer CdI2 should exhibit a relatively low photocatalytic activity due to its large band gap (about 3.0 eV). Some favourable doping can introduce extra bands to its band gap with the redox potentials of water straddled in its band gap, which can lead to improved photocatalytic performance. Multilayer CdI2 with a narrower band gap may absorb a finite amount of visible light, making it a more suitable photocatalyst. Furthermore, multilayer CdI2 exhibits significant modulation of its band gap and band alignment by applying normal strain and a vertical electric field. A reduced band gap with the CBM and VBM approaching the H+/H2 and the H2O/O2 potentials, respectively, can result in an enhanced photocatalytic activity by applying normal strain and a vertical electric field.
Introduction
The conversion of solar light into hydrogen by photocatalytic water splitting is an attractive technology for producing an alternative energy resource to fossil fuels without environmental pollution.1–4 To achieve high efficiency, the photocatalyst should satisfy several requirements.5,6 The photocatalyst should have suitable band edges to straddle the redox potentials of water. Specifically, its conduction band minimum (CBM) should be higher than the hydrogen reduction potential (H+/H2) and the valence band maximum (VBM) should be lower than the water oxidation potential (H2O/O2). The standard H+/H2 and H2O/O2 potentials with respect to the vacuum level are −4.44 eV and −5.67 eV, respectively.7 In addition to this, the photocatalyst should possess a band gap between 1.5 and 3.0 eV in order to utilize UV light and visible light.8,9
Recently, various two-dimensional (2D) materials have been predicted to have remarkable photocatalytic activities.10–12 First-principles calculations have predicted that single-layer group-III monochalcogenides are suitable for photocatalytic water splitting.13 Some previous works demonstrated that single-layer MoS2, WS2, PtS2 and PtSe2 are potential photocatalysts due to their suitable band edges with respect to the redox potentials of water.14–16 2D SnS and GeSe materials are demonstrated to be promising alternative materials for optoelectronic applications.17–20 Furthermore, heterobilayer GeSe/SnS could have promising applications in photovoltaic devices by modifying its electronic structure.21 These 2D materials maximize the surface area available for water splitting and minimize the distance of generated electron and hole migration, which enhances their catalytic performance.22
Beyond 2D chalcogenide materials, metal halide materials with layered crystal structures (e.g., PbI2 and CdI2) have also attracted significant attention due to their importance as precursors for the synthesis of halide perovskites.23–27 Our previous works have demonstrated that layered PbI2 possesses great potential in photovoltaic devices.28,29 2D CdI2 nanoplates have also been synthesized by using a vapor transport and deposition approach.25,30,31 However, the physical properties of 2D CdI2 are far from clear. In particular, we focus on layered CdI2 as a possible photocatalyst and propose some effective approaches to improve its photocatalytic performance.
Computational methods
All calculations are implemented in the VASP code32,33 within the projector-augmented plane-wave method.34 The generalized gradient approximation (GGA) of the Perdew, Burke and Ernzerhof (PBE) functional35 is adopted for the electron exchange and correlation. The plane-wave cutoff energy is set as 450 eV and the HSE06 hybrid functional36–38 is used to calculate the band structure of monolayer CdI2. A vacuum larger than 15 Å is used to simulate the isolated sheet. The pair-wise force field in the DFT-D2 method of Grimme39 is employed in the calculations of multilayer CdI2 to correctly describe the vdW interactions between layers. For the relaxation of CdI2 of various thicknesses, a (15 × 15 × 1) Monkhorst–Pack grid40 is used, whereas for the relaxation of a doped CdI2 monolayer, the first Brillouin zone is sampled with a (5 × 5 × 1) Monkhorst–Pack grid. All structures are fully relaxed with a force tolerance of 0.02 eV Å−1.
Results and discussion
Compared with layered transition metal dichalcogenides, CdI2 has a layered structure with a hexagonal unit cell and it crystallizes in the space group P63mc. This indicates that the 1T phase of CdI2 is the most stable, rather than the 2H phase. As shown in Fig. 1a, each sheet of Cd atoms is sandwiched between two sheets of I atoms through strong ionic bonding,41,42 and the atomic layers combined together via weak van der Waals (vdW) interactions. The optimized lattice constant of monolayer CdI2 is 4.33 Å. Its band structure calculated on the PBE level indicates an indirect band gap of 2.53 eV in Fig. 1b. According to the calculated density of states (Fig. 1c), the VBM is mainly contributed to by Cd_p and I_p orbitals and the CBM is composed of I_p and Cd_s orbitals. In order to correct the PBE band gaps, a hybrid Heyd–Scuseria–Ernzerhof functional is adopted to describe the electron exchange and correlation (Fig. S1†). The general features of the band structure are well preserved except for a larger band gap than that of the PBE functional. Furthermore, the spin–orbit coupling (SOC) effect is taken into consideration in Fig. S1.† An obvious spin–orbit splitting is observed at the VBM of the band structure. As a result, the initial quadruple degenerate VBM becomes doubly degenerate. Meanwhile, the SOC exerts little influence on the CBM of the CdI2 bands. This is attributed to the strong coupling between the Cd_d and I_p orbitals at the VBM and the absence of the Cd_d orbital at the CBM in the band structure of CdI2. Although an indirect band gap is not favourable for water splitting, layered CdI2 has suitable band edges to straddle the redox potentials of water. The photocatalytic activity can be enhanced when combining CdI2 with other photocatalysts, such as C3N4, to construct a direct Z-scheme type photocatalysis system for water splitting.43,44 What is more, the imaginary part of the dielectric function and light absorption (Fig. S2†) indicate good absorption in solar light. Our results are in good agreement with previous works except for an energy shift that is due to the underestimation of the GGA-PBE functional. CdI2 thin films are reported by experimental works to have a direct optical energy gap of ∼3.5 eV which seems too large for photocatalysis.30,31 It is indicated by our results and previous experimental works that CdI2 exhibits good absorption in ultraviolet light and has great potential in photocatalysis. Efficient ways to modulate its band edges are desired to improve the optoelectronic properties and the photocatalytic activity.
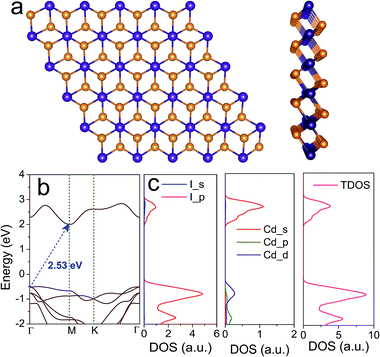 |
| Fig. 1 (a) The lattice structure of single-layer CdI2 from top and side views. The purple and brown balls denote the Cd and I atoms, respectively. (b) The band structure of single-layer CdI2 and (c) the projected density of states (PDOS) and total density of states (TDOS). | |
It seems that the band gap of monolayer CdI2 is too large to efficiently utilize the visible light in photocatalysis. It is well known that the electronic properties including the band gaps of most layered transition metal dichalcogenides are strongly dependent on the thickness of the materials.45,46 Thus we checked the thickness-dependent electronic structure of CdI2 as shown in Fig. 2a and b. It is indicated that both the CBM and the VBM approach the Fermi level, resulting in a decrease of the band gap of CdI2 with thickness. The vacuum level is taken as a reference in Fig. 2b. It is indicated in Fig. 2b that the energies of both the CBM and the VBM increase with the number of layers. Furthermore, the band gap decreases gradually with the number of layers. The evolution of the band edges and band gap with the thickness is not very significant because of the rather weak interlayer coupling. What is more, the redox potentials of water are straddled between the band edges of CdI2 with various thicknesses. The reduced band gap can make CdI2 a good photocatalyst with it absorbing solar light over a wider wavelength range. Consequently, the photocatalytic performance can be improved to some extent by increasing the thickness of CdI2 to a suitable value.
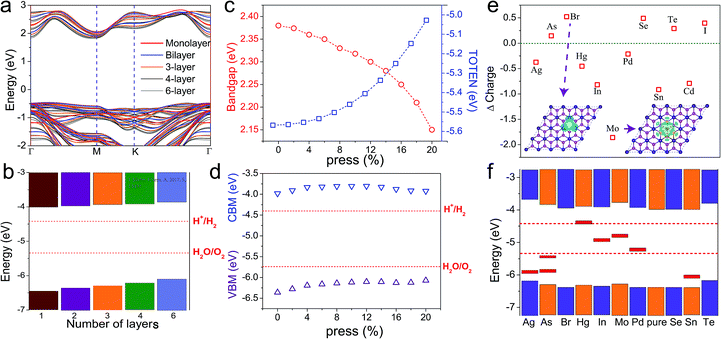 |
| Fig. 2 (a) Band structures and (b) band alignments of CdI2 of different thickness. Evolution of (c) band gap (red circles), total energy (blue squares) and (d) band edges of the CdI2 bilayer as functions of the applied normal strain. (e) Transfer charge results from Bader charge analysis in the doped CdI2 monolayer. The insets are the charge density difference in the Mo-doped and the Br-doped CdI2 monolayer. (f) Band alignments of the doped CdI2 monolayer. The red stripes in the band gap are the dopant bands introduced by substitution. The vacuum level is set as zero and is taken as a reference in (b), (d), and (f). The redox potentials of water are plotted as red dashed lines. | |
According to Fig. 2a and b, the band edges and band gap of CdI2 exhibit weak dependence on the thickness with respect to most 2D transition metal dichalcogenides. An efficient approach to improve its photocatalytic performance is desired. It has been demonstrated that 2D materials exhibit significant modulation of their electronic structures by applying a normal strain. The evolution of the band structures of bilayer Cd2 with an applied normal strain is shown in Fig. S3.† The normal strain is calculated as ε = (d0 − d)/d0. Our results indicate that the band gap of bilayer CdI2 decreases with the applied normal strain (Fig. 2c). Clear insight can be obtained from the variation of the band edges of bilayer CdI2 with the applied normal strain (Fig. 2d). The VBM increases gradually with the applied strain while the CBM does not show a monotonous trend because of the CBM’s shift from the M point to the Γ point. Furthermore, the redox potentials of water are always straddled between the VBM and the CBM of the pressed CdI2 bilayer. The CdI2 bilayer with a reduced band gap from the application of normal strain is a good photocatalyst with improved performance due to the widened wavelength range for light absorption.
It should be mentioned that the interlayer binding in the CdI2 bilayer is relatively weaker than that in most of the transition metal dichalcogenide bilayers such as those of MoS2. Fig. 2c also shows the evolution of the total energy of the CdI2 bilayer. The charge density difference (Fig. S4†) shows slight charge transfer between layers in the CdI2 bilayer upon binding together. The weak binding gives rise to the relatively weak response of the electronic structure and strain energy to the applied strain. Similar evidence comes from the integrated charge density of the CdI2 bilayer under different normal strain (Fig. S5†). It is found that applying a normal strain will bring about extra charge exchanging between layers. It can be concluded that applying a normal strain is an efficient way to improve the photocatalytic performance.
Besides applying normal strain, substituted doping has also been demonstrated to be an effective method to modulate the band alignment of a semiconductor. In Fig. 2e and f, several non-magnetic atoms are employed to make substituting dopants in a (4 × 4 × 1) CdI2 monolayer supercell. Dopant bands are induced either in the band gap or near the band edges (Fig. S6†), which should exert a significant influence on the optoelectronic and photovoltaic properties of monolayer CdI2. In Fig. 2e, Bader charge analysis demonstrates that charge transfer occurs between dopants and it defected the CdI2 monolayer.
The band alignments of these doped CdI2 monolayers are plotted in Fig. 2f. It is found that In, Mo and Pd will introduce dopant bands in the redox potential of water, making the corresponding doped CdI2 not suitable for photocatalysis, while other dopants employed here will not introduce extra bands in the redox potential of water. Ag, As and Sn dopants will induce extra bands between the VBM and the H2O/O2 potential, and Hg can induce extra bands between the H+/H2 potential and the CBM, making the corresponding doped CdI2 suitable for photocatalysis in water splitting.
In many optoelectronic and photovoltaic devices, the materials are always subjected to a vertical electric field (E⊥). Multilayer 2D materials usually exhibit significant modulation of their electronic structures by applying an E⊥. As shown in Fig. 3a, the band structures of the CdI2 bilayer under different E⊥ are calculated. Our results indicate that its band gap is significantly reduced by the applied E⊥. Further insight can be obtained from the variation of the band edges with the E⊥ in Fig. 3b. It is clear that the CBM exhibits a linear descending trend with the E⊥ while the VBM is hardly influenced by the E⊥. As a photocatalyst, when subjected to a vertical electric field, the CBM of CdI2 will approach the H+/H2 level. This will improve the absorption of solar light, further leading to enhanced photocatalytic activity. However, a strong E⊥ will reduce the CBM of CdI2 to the position below the H+/H2 level, making CdI2 unsuitable as a photocatalyst.
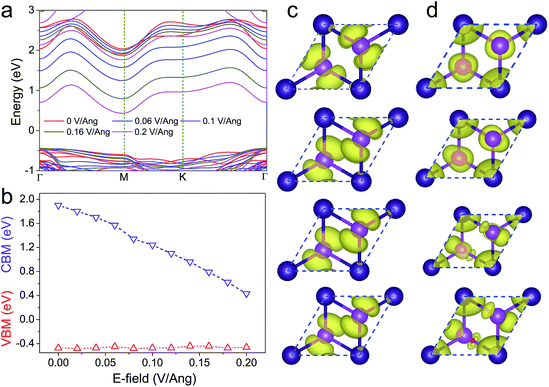 |
| Fig. 3 Evolution of the (a) band structure and (b) band edges of the CdI2 bilayer as functions of the applied vertical electric field. (c) and (d) plot the band decomposed charge density of the VBM and CBM at four high-symmetry k-points (Γ, M, K, A) of the CdI2 bilayer, respectively. The A point is the k-point between the K and the Γ points. | |
To make the distinct responses of the VBM and the CBM to the applied E⊥ clear, the band decomposed charge density of the CBM and the VBM at the four high-symmetry k-points (Γ, M, K, A) is shown in Fig. 3c and d. A is the k-point between K and Γ, which is given in Fig. 3a. As can be seen, the lowest-energy carriers (holes) are almost completely localized around the I atoms, forming π bonds. The absence of a polarized distribution between the I and Cd atoms results in the weak response of the VBM to the applied E⊥, while at the CBM, the lowest-energy carriers (electrons) are neither localized on the I atoms nor the Cd atoms. Charge exchange exists between I and Cd atoms. As a result, the applied E⊥ exerts a significant influence on the CBM.
Conclusions
In summary, the electronic structure of 2D CdI2 is explored by density functional theory calculations. The calculated results of the band gaps and band edges demonstrated that CdI2 is a suitable photocatalyst for water splitting. Monolayer CdI2 should exhibit a low photocatalytic activity due to its large band gap (about 3.0 eV). Multilayer CdI2 with a narrower band gap may absorb a finite amount of visible light, making it a more suitable photocatalyst. What is more, its photocatalytic activity can be enhanced by applying normal strain and a vertical electric field with two distinct mechanisms. Both the CBM and VBM of bilayer CdI2 can be tuned by applying a normal strain, while a vertical E⊥ is only able to modulate the CBM of bilayer CdI2. Some favourable substituting dopants can also make the band alignment of CdI2 more suitable for photocatalysis. These results provide valuable guidance for enhancing the photocatalytic activity of 2D CdI2.
Conflicts of interest
There are no conflicts to declare.
Acknowledgements
This work was financially supported by the National Natural Science Foundation of China (Grant no. 11674310, 61622406, 61571415, 51502283, and 51602065), the National Key Research and Development Program of China (Grant No. 2016YFB0700700), “Hundred Talents Program” of Chinese Academy of Sciences (CAS), and the CAS/SAFEA International Partnership Program for Creative Research Teams.
References
- T. Simon, N. Bouchonville, M. J. Berr, A. Vaneski, A. Adrovic, D. Volbers, R. Wyrwich, M. Döblinger, A. S. Susha and A. L. Rogach, et al., Nat. Mater., 2014, 13, 1013 CrossRef CAS PubMed
. - X. Chen, L. Liu, Y. Y. Peter and S. S. Mao, Science, 2011, 331, 746 CrossRef CAS PubMed
. - R. Asahi, T. Morikawa, H. Irie and T. Ohwaki, Chem. Rev., 2014, 114, 9824 CrossRef CAS PubMed
. - J. Ran, J. Zhang, J. Yu, M. Jaroniec and S. Z. Qiao, Chem. Soc. Rev., 2014, 43, 7787 RSC
. - Y. Gai, J. Li, S.-S. Li, J.-B. Xia and S.-H. Wei, Phys. Rev. Lett., 2009, 102, 036402 CrossRef PubMed
. - J. Kang, S. Tongay, J. Zhou, J. Li and J. Wu, Appl. Phys. Lett., 2013, 102, 012111 CrossRef
. - V. Chakrapani, J. C. Angus, A. B. Anderson, S. D. Wolter, B. R. Stoner and G. U. Sumanasekera, Science, 2007, 318, 1424 CrossRef CAS PubMed
. - A. K. Singh, K. Mathew, H. L. Zhuang and R. G. Hennig, J. Phys. Chem. Lett., 2015, 6, 1087 CrossRef CAS PubMed
. - S. Chen and L.-W. Wang, Chem. Mater., 2012, 24, 3659 CrossRef CAS
. - W. J. Ong, Front. Mater., 2017, 4, 11 Search PubMed
. - D. Zeng, L. Xiao, W. J. Ong, P. Wu, H. Zheng, Y. Chen and D. L. Peng, J. Mater. Chem. A, 2017, 5, 16171–16178 CAS
. - X. Lv, W. Wei, Q. Sun, F. Li, B. Huang and Y. Dai, Appl. Catal., B, 2017, 217, 275 CrossRef CAS
. - H. L. Zhuang and R. G. Hennig, Chem. Mater., 2013, 25, 3232 CrossRef CAS
. - H. L. Zhuang and R. G. Hennig, J. Phys. Chem. C, 2013, 117, 20440 CAS
. - N. Singh, G. Jabbour and U. Schwingenschlögl, Eur. Phys. J. B, 2012, 85, 392 CrossRef
. - G. Guo and W. Liang, J. Phys. C: Solid State Phys., 1986, 19, 995 CrossRef CAS
. - P. D. Antunez, J. J. Buckley and R. L. Brutchey, Nanoscale, 2011, 3, 2399 RSC
. - A. K. Singh and R. G. Hennig, Appl. Phys. Lett., 2014, 105, 042103 CrossRef
. - Z. Ma, B. Wang, L. Ou, Y. Zhang, X. Zhang and Z. Zhou, Nanotechnology, 2016, 27, 415203 CrossRef PubMed
. - L. Huang, F. Wu and J. Li, J. Chem. Phys., 2016, 144, 114708 CrossRef PubMed
. - C. Xia, J. Du, W. Xiong, Y. Jia, Z. Wei and J. Li, J. Mater. Chem. A, 2017, 5, 13400 CAS
. - A. Kudo and Y. Miseki, Chem. Soc. Rev., 2009, 38, 253 RSC
. - Y. Hu, Y. Guo, Y. Wang, Z. Chen, X. Sun, J. Feng, T. M. Lu, E. Wertz and J. Shi, J. Mater. Res., 2017, 325, 1 Search PubMed
. - J. Zhang, T. Song, Z. Zhang, K. Ding, F. Huang and B. Sun, J. Mater. Chem. C, 2015, 3, 4402 RSC
. - R. Ai, X. Guan, J. Li, K. Yao, P. Chen, Z. Zhang, X. Duan and X. Duan, ACS Nano, 2017, 11, 3413 CrossRef CAS PubMed
. - I. Karbovnyk, I. Bolesta, I. Rovetskyi, V. Lesivtsiv, Y. Shmygelsky, S. Velgosh and A. Popov, Low Temp. Phys., 2016, 42, 594 CrossRef CAS
. - J. Robertson, J. Phys. C: Solid State Phys., 1979, 12, 4753 CrossRef CAS
. - M. Zhong, S. Zhang, L. Huang, J. You, Z. Wei, X. Liu and J. Li, Nanoscale, 2017, 9, 3736 RSC
. - M. Zhong, L. Huang, H.-X. Deng, X. Wang, B. Li, Z. Wei and J. Li, J. Mater. Chem. C, 2016, 4, 6492 RSC
. - P. Tyagi, A. G. Vedeshwar and N. C. Mehra, Phys. B, 2001, 304, 166 CrossRef CAS
. - I. A. Kariper, J. Mater. Res. Technol., 2016, 5, 77 CrossRef CAS
. - G. Kresse and J. Furthmüller, Phys. Rev. B, 1996, 54, 11169 CrossRef CAS
. - G. Kresse and J. Furthmüller, Comput. Mater. Sci., 1996, 6, 15 CrossRef CAS
. - P. E. Blöchl, Phys. Rev. B, 1994, 50, 17953 CrossRef
. - J. P. Perdew, K. Burke and M. Ernzerhof, Phys. Rev. Lett., 1996, 77, 3865 CrossRef CAS PubMed
. - J. Heyd, G. E. Scuseria and M. Ernzerhof, J. Chem. Phys., 2003, 118, 8207 CrossRef CAS
. - J. Heyd, J. E. Peralta, G. E. Scuseria and R. L. Martin, J. Chem. Phys., 2005, 123, 174101 CrossRef PubMed
. - J. Heyd, G. E. Scuseria and M. Ernzerhof, J. Chem. Phys., 2006, 124, 9906 CrossRef
. - S. Grimme, J. Comput. Chem., 2006, 27, 1787 CrossRef CAS PubMed
. - H. J. Monkhorst and J. D. Pack, Phys. Rev. B, 1976, 13, 5188 CrossRef
. - İ. A. Kariper, J. Mater. Res. Technol., 2016, 5, 77 CrossRef
. - Y. Wang, Y.-Y. Sun, S. Zhang, T.-M. Lu and J. Shi, Appl. Phys. Lett., 2016, 108, 013105 CrossRef
. - J. Wang, Z. Guan, J. Huang, Q. Li and J. Yang, J. Mater. Chem. A, 2014, 2, 7960 CAS
. - Q. Li, N. Zhang, Y. Yang, G. Wang and D. H. Ng, Langmuir, 2014, 30, 8965 CrossRef CAS PubMed
. - W. Jin, P.-C. Yeh, N. Zaki, D. Zhang, J. T. Sadowski, A. Al-Mahboob, A. M. van Der Zande, D. A. Chenet, J. I. Dadap and I. P. Herman, et al., Phys. Rev. Lett., 2013, 111, 106801 CrossRef PubMed
. - R. Coehoorn, C. Haas, J. Dijkstra, C. Flipse, R. De Groot and A. Wold, Phys. Rev. B, 1987, 35, 6195 CrossRef CAS
.
Footnote |
† Electronic supplementary information (ESI) available. See DOI: 10.1039/c7ra09687a |
|
This journal is © The Royal Society of Chemistry 2017 |
Click here to see how this site uses Cookies. View our privacy policy here.