Bio-based acrylic acid from sugar via propylene glycol and allyl alcohol†
Received
14th July 2017
, Accepted 23rd November 2017
First published on 24th November 2017
Abstract
A new route for producing bio-based acrylic acid is proposed. It starts with the conversion of carbohydrates to propylene glycol, being main or by-product, and proceeds via a subsequent dehydration to allyl alcohol under gas-phase conditions over K-modified ZrO2 and a final oxidation over MoWVOx catalyst at atmospheric pressures. Although preliminary, this study shows the possibility to convert propylene glycol to allyl alcohol with ∼50 mol% selectivity (at ∼64 mol% conversion) and convert allyl alcohol to acrylic acid with ∼77 mol% selectivity (at ∼90 mol% conversion). The oxidation of unconverted propylene glycol or the by-products propanal and 1-propanol results in propionic acid under these conditions. A preliminary process flow scheme is proposed based on the distillation resistance of the reaction products.
Introduction
The present-day world is increasingly looking for biomass-based chemicals as renewable and possibly, environmentally friendlier alternative to petrochemicals.1–3 The US Department of Energy has identified 12 platform molecules that could be derived from biomass and lead to high-value chemicals.4 The upgrading of such platform molecules to chemicals using homogeneous and heterogeneous catalysts has been reported recently.5–7 The most promising feedstock is the carbohydrates that are contained in lignocellulose, in starch or as sugar syrup. Acidic hydrolysis of starch8,9 or cellulose10–12 gives glucose which is further converted to various platform molecules via a combination of reactions such as bond cleavage, hydrogenation and isomerization. A route of interest here is the hydrogenolysis of carbohydrates to a mixture of polyols, mainly glycerol, propylene glycol (PG) and ethylene glycol (EG),13–22 which are intermediate chemicals in their own rights but can also be further upgraded to other chemicals. One promising derivative of carbohydrate is acrylic acid (AcrA). It is used in large volumes (8 Mt a−1) for a wealth of applications that vary from acrylate coatings, to super absorbents, to adhesives and other materials.23,24
Bio-based acrylic acid can be produced from glycerol in two steps, namely the dehydration of glycerol to acrolein (ACR)25,26 and its subsequent oxidation to acrylic acid. The oxidation step is part of the conventional route that is based on propene oxidation that proceeds via acrolein and uses mixed Mo–oxides as catalyst. Recently, Zhang et al. reported the conversion of glycerol to acrylic acid via allyl alcohol (AA).27 In their work, glycerol is hydro-deoxy-dehydrated to allyl alcohol using formic acid as a sacrificial reagent, and then oxidized to acrylic acid using supported and unsupported MoWVOx catalysts. Although intriguing, this route seems less promising than the straight dehydration–oxidation route because of the need for sacrificial formic acid and additional O2 demand in the oxidation step.
Other routes to bio-based acrylic acid proceed via the conversion of carbohydrates to lactic acid or 3-hydroxy-propionic acid followed by their dehydration to acrylic acid.28–31 Lactic acid is also a potential intermediate in the conversion propylene glycol to acrylic acid32 as it can be produced by liquid-phase oxidation of propylene glycol.33,34 Alternative routes to upgrade propylene glycol to acrylic acid have not yet been disclosed. The dehydration of propylene glycol is generally carried out in the presence of acidic catalysts and leads to propanal (PAL), which can't be easily oxidized to acrylic acid.35–38 Allyl alcohol is only observed in minor amounts, if at all detected. We propose here a route that reverses the order of the reactions (Scheme 1), i.e., it proceeds via dehydration to form allyl alcohol and its oxidation to acrylic acid. We demonstrate 50% selectivity for the dehydration step using basic catalysts and 77% selectivity for the oxidation step using a MoWVOx oxidation catalyst. We also present a basic process scheme that accounts for the reactivity and separation characteristics of the reaction effluents.
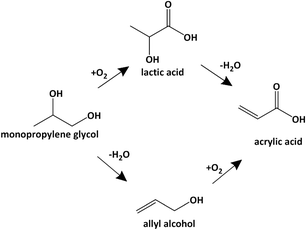 |
| Scheme 1 Plausible reaction scheme for the conversion of propylene glycol to acrylic acid. | |
The base-catalysed dehydration explored here was inspired by Sato et al. who reported the dehydration of 2,3-butanediol to 3-butene-2-ol using ZrO2 and CaO-modified ZrO2.39,40 The oxidation catalyst was taken from the work of Li and Zhang.27 The route proposed here contrasts to Li and Zhang's route by not using sacrificial formic acid.
It should be stressed that this preliminary study is focusing on proving the overall manufacturing chain and identifying key technology challenges to improve on. It, thereby, voluntarily spends moderate attention to catalyst synthesis, catalyst characterization and reaction mechanism, which will be topics of future studies.
Experimental section
Catalyst preparation
The basic dehydration catalysts were prepared by impregnation-drying of monoclinic ZrO2 (BET = 84 m2 g−1; from Gimex Technische keramiek b.v.) with KOH (from Sigma Aldrich). Typically, the required amount of KOH granules was dissolved in approximately 20 mL of water, a calculated amount of ZrO2 was added, stirred for 2 h at room temperature and then for 2–4 h at 100 °C to evaporate the water. The resulting wet paste was dried under vacuum overnight at 100 °C. The resulting catalyst is designated as nKZrO2 in which “n” indicates the weight percentage of KOH on ZrO2.
The oxidation catalyst was prepared by drying a solution of V, Mo and W salts as described in the literature.27 Typically, 2.6 g of ammonium monovanadate (from Merck), 14.7 g of Ammonium heptamolybdate (from Alfa Aesar) and 2.7 g of ammonium metatungstate (from Alfa Aesar) were dissolved in deionized water and then evaporated to dryness. This mixture was then calcined at 275 °C for 4 h and at 325 °C for 4 h under air and N2, respectively. The resulting catalyst shows Mo
:
W
:
V atomic ratios of about 7.3
:
1
:
2 and is designated as MoWVOx.
Catalyst characterization
All catalysts were characterized by N2 sorption at 77 K for BET surface area determination, using Micromeritics TriStar 3000 V6.03 analyzer. Prior to the analysis, the catalysts were degassed at 150 °C. X-ray diffraction patterns of the catalysts were obtained using Bruker D2 Phaser diffractometer with a CuKα radiation of 0.1544 nm.
Catalytic testing
The dehydration tests were performed in a fixed-bed, down flow quartz reactor (400 mm long, 4 mm id. and 6 mm od,) suspended in an electrical furnace. The catalyst (0.2 g with a particle size between 0.4–0.6 mm) was mixed with silica beads in similar amount and size, and held in the reactor between two quartz wool plugs. The liquid feed, an aqueous solution of 25 v% propylene glycol (from Sigma Aldrich), was pumped at a flow rate of 0.5 ml h−1 into the preheater maintained at 225 °C, along with Ar as carrier gas at 1200 ml h−1 and send onto the catalyst bed at a GHSV of 9051 l l−1 h−1 and the overall composition of Ar
:
PG
:
H2O ratio = 31
:
1
:
12 (mol/mol). GHSV is calculated using the formula,
The products were condensed using a cold trap (5–10 °C) placed at the bottom of the reactor. Uncondensed vapours and the gases were sent into an online gas chromatograph. The liquid products were analysed offline using high pressure liquid chromatograph. Both chromatographs were calibrated with all the reactants and possible products before quantification. Following formulas were used to calculate the conversion, selectivity and yield of the reactions.
The oxidation tests were performed with the same setup and the packing procedure of the catalyst (0.2 g) was followed exactly as mentioned earlier. The aqueous allyl alcohol (from Sigma Aldrich) solution was pumped at a flow rate of 0.5 ml h−1 into the preheater maintained at 150 °C along with the carrier gas (Ar) at 1080 ml h−1. Pure Oxygen (120 ml h−1) was added to the feed stream after the vaporizer and the final gas stream was fed at a GHSV of 8945 l l−1 h−1, and a molar composition of AA
:
H2O
:
Ar
:
O2 = 1
:
9
:
22
:
2.4 (mol/mol). Occasionally, allyl alcohol was replaced by propanal or 1-propanol (POL) from Sigma Aldrich and fed at a GHSV of 9426 l l−1 h−1 and composition of propanal (or 1-propanol): H2O
:
Ar
:
O2 = 1
:
36
:
68
:
7.7 (mol/mol). The reaction products were condensed using a cold trap (5–10 °C) placed at the bottom of the reactor. Uncondensed vapours and the gases were sent to an online gas chromatograph. The liquid products were analyzed offline using high pressure liquid chromatograph.
Results and discussion
Dehydration of propylene glycol
A typical performance of vapour-phase dehydration of propylene glycol over 10KZrO2 catalyst is illustrated in Fig. 1. The data reported corresponds to the initial activity measured at 400 °C and varying residence time using a feed of Ar
:
PG
:
water molar ratio of 31
:
1
:
12. Expectedly, the conversion increases with increasing residence time. The selectivity to allyl alcohol is high around 57 C% at low residence time and low conversion but plummets as the conversion exceeds 70%. Meanwhile, the selectivities for the by-products propanal and 1-propanol pass through a broad maximum at 8–12 C%. Other potential by-products such as hydroxyacetone (HA), lactaldehyde (LA), acetone (ACE) and acrolein could not be observed in any significant amounts here. However, the carbon balance did not add up to 100 C%, particularly at high conversion. The dotted lines represent extrapolated selectivities that add up to 100%. This results in an apparent selectivity for ‘unknown’ products around 25–35 C% at moderate residence time, up to 80 C% at high residence time and high conversion. Much of this unknown product is attributed to heavy components, as witnessed by large default in carbon balance and by brown deposit observed on the reactor wall, downstream of the catalyst bed. The deposit could not be dissolved completely in solvents such as methanol or 1-butanol. GC/MS analysis revealed the presence of four dipropylene glycol ether isomers as well as light methyl-cyclohexanones (Table S2, ESI†). Methyl-cyclopentenones are probably formed by aldol-condensation product hydroxyacetone with itself or with propanal, as illustrated in scheme (Scheme S1, ESI†). They can also undergo subsequent aldol condensation reactions towards heavy products. The same holds for aldol condensation of propanal and etherification of PG (Scheme S2, ESI†). Hence, these reactions likely contributed to the formation of heavier products that condense on the reactor wall and polymerize under reaction conditions. Variations in process conditions were also investigated but resulted all in lower selectivity towards allyl alcohol. This was the case for operation at lower temperature and with more concentrated feed (Fig. S1 and S2, ESI†). More efforts should be devoted to identify the unknown products and the reactions that are responsible to their formation.
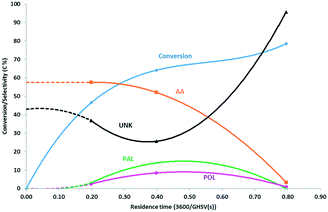 |
| Fig. 1 Effect of residence time on the dehydration of propylene glycol over 10KZrO2. Reaction conditions: 25 v% aq. PG, temperature = 400 °C, Ar : PG : H2O ratio = 31 : 1 : 12, AA: Allyl alcohol, PAL: Propanal, POL: 1-propanol, UNK: unknowns. | |
A preliminary kinetic analysis suggests that the propylene glycol conversion is 1st order in propylene glycol (Fig. S3†), shows no statistically significant order in water (Fig. S5†) and shows apparent activation energy around 100 kJ mol−1 (Fig. S4†). The runs were usually completed within a single day of 4–6 h duration and showed no clear indication of catalyst deactivation. If anything, the catalyst seemed even to activate during such short runs (Fig. S3–S6, ESI†). Eventual long-term deactivation should be investigated in future research.
Effects of catalyst formulations were also investigated. Lower K-loading on ZrO2 resulted in increased activity and in a selectivity shift towards more propanal and 1-propanol (Table S1, ESI†). Interestingly, acetone and hydroxyacetone were observed in significant amounts now. Lower K-loading also resulted in lower selectivity for unknown, which contained various aldol condensation products at high conversion and some dioxolanes–ketalization products of propylene glycol with carbonyls such as propanal at lower conversion (Table S3, ESI†).
The production of allyl alcohol is not limited to KZrO2 catalysts. A calcium hydroxyapatite (Ca/P = 1.56 atom/atom) showed significant selectivity towards allyl alcohol(Table S1, ESI†). Hydroxyapatites with higher Ca/P ratio are known to have higher basicity and are thereby expected to be good candidates as well.
Although too incomplete to be conclusive, the selectivity data reported here shed some light on the reaction chemistry. K-doped catalysts seem to dehydrate propylene glycol to allyl alcohol as primary product, which undergoes secondary isomerization towards propanal and further hydrogenation to 1-propanol or aldol condensation to heavy products. Catalysts with no/low K-doping are more active and favour propylene glycol conversion to 1-propanol and hydroxyacetone as main products with comparable selectivity. This suggests that propylene glycol is dehydrated to propanal, which undergoes H-transfer from unconverted propylene glycol to form hydroxyacetone and 1-propanol. Such H-transfer reaction has been indeed reported previously for ZrO2 catalysts.41,42
Suggested reaction scheme is displayed in Scheme 2. We propose that K-doping seems to poison Lewis acid sites43 that catalyse dehydration of propylene glycol to propanal as well as the H-transfer reaction. However, a more detailed study is required to ascertain these preliminary conclusions on the reaction scheme. Therefore, with such results, we decided not to optimize further but rather check the subsequent step, i.e., the oxidation of allyl alcohol to acrylic acid.
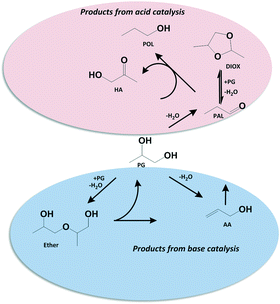 |
| Scheme 2 Observed reaction scheme during the dehydration of propylene glycol over ZrO2 catalysts. | |
Oxidation of Allyl alcohol
Allyl alcohol has been reported to be oxidized to acrylic acid using MoVW-based oxide catalysts.27,44 We could confirm this claim using an unsupported MoVW mixed oxide catalyst as displayed in Fig. 2. Acrylic acid was obtained at a maximum selectivity of ∼77 mol% with an allyl alcohol conversion of ∼90 mol% at 310 °C. Higher temperatures resulted in a drop in acrylic acid yield due to the formation of CO2, acetic acid (AceA) and unknown products which may be resulting from the polymerization of acrylic acid and acrolein. Indeed, brownish material was observed on the inner walls of the reactor downstream of the catalyst.
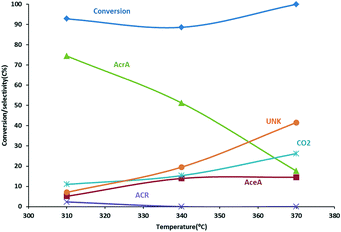 |
| Fig. 2 Effect of temperature on the oxidation of Allyl alcohol over MoVWOx catalyst. Reaction conditions: 30 v% aq. AA, AA : H2O : Ar : O2 = 1 : 7.5 : 19 : 2.1 (mol/mol), GHSV: 6372 h−1, AceA: acetic acid, AcrA: acrylic acid, ACR: acrolein, UNK: unknowns. | |
The catalyst used for this reaction is closely related to that of the catalyst used for acrolein oxidation in the conventional propene-based process.45–47 The addition of W is claimed to provide structural stability.48 The addition of water to the feed has been claimed to facilitate the desorption of acrylic acid from the catalyst surface and to depress coke formation, increasing thereby the selectivity to acrylic acid,49,50 although beneficial effect has been reported to be minimal at higher temperatures.51
Since the chemistry of the oxidation of allyl alcohol was discussed in more depth in the literature,27 this study chose to focus on new and complementary aspects of the process, namely the reactivity of dehydration by-products. Allyl alcohol was not the sole product of propylene glycol dehydration; propanal and 1-propanol were also produced in significant amounts. We, therefore, explored the possibility to convert these by-products to acrylic acid as well. However, preliminary oxidation experiments did not show much promising results with this catalyst (Fig. S7 and S8, ESI†). The oxidation resulted mainly in CO2, unknown products and smaller amounts of acetic and propionic acids. This may, however, be partly due to the large excess of oxygen used in these runs, namely 7.7 molar equivalents instead of 2. Oxidation of C3 oxygenates like propanal and 1-propanol was evaluated with diluted feed because these by-products are expected to be present in low concentration in the real feed. This explains the high O2/oxygenate feed ratio. We also studied the oxidation of propylene glycol directly. However, acrylic acid was observed below 3 mol% in selectivity. As observed in the other oxidation cases, the main products consisted of propionic and acetic acids at low temperature (340 °C) and of CO2 and unknowns at higher temperatures (400 °C) (Fig. S9, ESI†). Further optimization of conditions and catalyst formulations is needed to enable co-processing of these feeds.
Distillation potential and preliminary process concept
Having proven the chemistry of the process, we then checked the distillation resistance of the two product streams to see whether their work up by means of distillation threatens to be costly. The distillation resistance, Ω52 is based on the relative mass fraction of each component and on the temperature gap between its atmospheric boiling point and that of the next heavier component. Analysis of industrial processes allowed to define a target for distillation resistance of <10–15 [1/°C] to limit the distillation costs to <$100 per ton product at 100 kton per annum scale. The full analysis is discussed in ESI† and only summarized below.
Analysis of the full dehydration effluent revealed a prohibitive resistance of ∼130[1/°C], which is due to the presence of three close boilers, namely allyl alcohol (97 °C), 1-propanol (98 °C) and water (100 °C) (Table S4, ESI†). These are even suspected to form azeotropic mixtures, which further complicates their separation. So, we need to consider recovering these components as a mixture and feed them unseparated to the oxidation step. Upon assuming that we can also recycle unconverted propylene glycol with eventual heavy products without separation, then the distillation resistance falls to 9.4[1/°C] (Table 1, top), i.e. within the industrial practice. Much of the remaining resistance is determined by the need to evaporate 6 ton of water per ton of allyl alcohol (based on 50 v% propylene glycol in water), which can be further reduced by either reducing the water dilution of the feed or by reaching higher yield per pass (assumed here at 30 mol%). These are clear targets for future research. Another research target would be ensuring that the heavy product can be recycled with unconverted propylene glycol.
Table 1 Distillation resistance of the dehydration effluent (top) and the oxidation effluent (bottom) without separation of close-boilers
Name |
Boiling point (°C) |
Concentration fi/fproda (ton/ton product) |
Ω
prod. (1/°C) |
Concentrations are normalized to allyl alcohol or acrylic acid concentrations for the dehydration and oxidation effluent, respectively. PAL: propanal, POL: 1-propanol, PG: propylene glycol, HE: heavier end products, ACR: acrolein, AA: allyl alcohol, AcrA: acrylic acid, PrAc: propionic acid. |
Dehydration effluent |
PAL |
48 |
0.52 |
1.0 |
Water/AA/POL |
100 |
7.36 |
8.4 |
PG + HE |
188 |
1.82 |
|
Total |
|
9.70 |
9.4 |
Oxidation effluent |
CO2 + gas |
−78 |
NA |
|
Acetaldehyde |
20.2 |
0.01 |
0.0 |
PAL |
48 |
0.01 |
0.2 |
ACR |
53 |
0.01 |
0.0 |
Water/AA/AceA |
100 |
3.26 |
7.9 |
AcrA/PrAc |
141 |
1.01 |
2.1 |
HE(PG) |
188 |
0.09 |
|
Total |
|
4.39 |
10.3 |
The product of oxidation step also contains pairs of close-boilers, namely allyl alcohol–water (97–100 °C) and acrylic acid–propionic acid (PrAc) (both ∼141 °C). The mixture of acrylic and propionic acids is generally separated by crystallization and it can be kept as mixture in the present analysis.53 Full separation of the other products results in a distillation resistance amounts to ∼22[1/°C] (Table S5, ESI†). Not separating allyl alcohol and acetic acid from the large amount of water (3 ton/ton acrylic acid) further decreases the resistance to 10[1/°C] (Table 1, bottom). Future research should then focus on decreasing the water/allyl alcohol ratio in the feed (here 8.7 molar and 2.7 by weight) while maintaining high yield towards acrylic acid.
Based on the information available, a preliminary process flow scheme can be proposed (Fig. 3). Accordingly, aqueous propylene glycol is evaporated and fed to the dehydration reactor. The effluent undergoes a crude separation to light products (e.g. propanal) and unconverted propylene glycol and other heavy product for recycling. The resulting aqueous allyl alcohol stream is then mixed with air and send to the oxidation reactor to produce a mixture of acrylic acid and propionic acid and a crude water stream that contains allyl alcohol and acetic acid. These two mixtures are then worked up by means of non-distillative separations to provide valuable by-products. The economic viability of such process will depend on future success in materializing the following improvements:
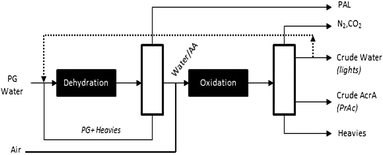 |
| Fig. 3 Preliminary process flow scheme for upgrading propylene glycol to acrylic acid industrial operating window. | |
• Minimizing the water dilution that is required for both dehydration and oxidation steps.
• Minimizing the by-production of 1-propanol and propanal during propylene glycol dehydration to minimize the formation of acetic and propionic acids.
• Demonstrating the possibility to recycle unconverted propylene glycol and other heavy products (particularly the dioxolane intermediates) for further conversion to allyl alcohol.
Interestingly, several catalytic performance reported here also meets common industrial practice, as proposed in the literature54 and discussed, detailed in ESI.† Accordingly, attention is to be paid to the catalyst selectivity, catalyst activity (more accurately volumetric reactor productivity), catalyst stability and product concentration. As shown in Table 2 (details in ESI†), no significant challenge is expected for meeting the industrial targets of catalyst activity and overall stability. However, the selectivity and product concentration of the propylene glycol dehydration step are falling short at this stage. It should be realized here that the selectivity target is not chosen arbitrarily but has been calculated based on feed and product prices, as proposed elsewhere.54 Future catalyst and process optimization needs to focus on improving the selectivity at lower dilution and or higher conversion. Further improvements of activity or stability does not seem necessary at this stage.
Table 2 Performance vs. proven industrial window (details in ESI)
|
Unit |
Target |
PG dehydration |
AA oxidation |
Key economic premises include acrylic acid and propylene glycol priced at $2000 per ton and $2200 per ton, respectively, a conversion cost of 2 × $200 per ton (for 2-step process) and no value for the by-product (no brackets) or a value of $1000 per ton for half the by- products (between brackets).
The stability target reports the life time prior to disposal, assuming the catalyst can be regenerated by coke burn off but needs to have produced >1 kg of product per g of catalyst prior to disposal. PG: propylene glycol, AA: allyl alcohol. |
Selectivity |
% theory |
>85(>75)a |
50 |
80 |
Activity |
g lreact−1 h−1 |
>100 |
500 |
500 |
Stability |
Monthb |
<24 |
5.5 |
2.5 |
Product concentration |
wt% |
>3 |
1 |
7 |
Conclusion
In conclusion, we show here the possibility to convert propylene glycol to acrylic acid via allyl alcohol using a simple two-step process. Allyl alcohol and acrylic acid selectivities of ∼50 mol% and 77 mol%, respectively are reported without extensive process and catalyst optimization. We also propose a preliminary process flow scheme and formulate several improvements that could help minimizing the production cost. This process concept might even be integrated into a conventional process for acrylic acid manufacture based on propene oxidation. Indeed, the propylene glycol dehydration effluent might be used as a feed supplement to the acrolein oxidation step of the conventional process.
Conflicts of interest
There are no conflicts of interest to declare.
Acknowledgements
The authors thank University of Twente for the experimental work and Shell Global Solution International b.v. for funding the project.
References
-
J.-P. Lange, Lignocellulose Conversion: An Introduction to Chemistry, Process and Economics, in Catalysis for Renewables, Wiley-VCH Verlag GmbH & Co. KGaA, 2007, pp. 21–51 Search PubMed.
- J.-P. Lange, Lignocellulose conversion: an introduction to chemistry, process and economics, Biofuels, Bioprod. Biorefin., 2007, 1(1), 39–48 CrossRef CAS.
-
J.-P. Lange, Resource Efficiency of Chemical Manufacturing Chains: Present and Future, in Sustainable Development in the Process Industries, John Wiley & Sons, Inc., 2010, pp. 23–37 Search PubMed.
-
T. Werpy and G. Peterson, Top value added chemicals from biomass, 2004 Search PubMed.
- A. Corma, S. Iborra and A. Velty, Chemical Routes for the Transformation of Biomass into Chemicals, Chem. Rev., 2007, 107(6), 2411–2502 CrossRef CAS PubMed.
- R. A. Sheldon, Green and sustainable manufacture of chemicals from biomass: state of the art, Green Chem., 2014, 16(3), 950–963 RSC.
- P. J. Deuss, K. Barta and J. G. de Vries, Homogeneous catalysis for the conversion of biomass and biomass-derived platform chemicals, Catal. Sci. Technol., 2014, 4(5), 1174–1196 CAS.
- V. B. Kumar, I. N. Pulidindi and A. Gedanken, Selective conversion of starch to glucose using carbon based solid acid catalyst, Renewable Energy, 2015, 78, 141–145 CrossRef CAS.
- A. Yepez, A. Garcia, M. S. Climent, A. A. Romero and R. Luque, Catalytic conversion of starch into valuable furan derivatives using supported metal nanoparticles on mesoporous aluminosilicate materials, Catal. Sci. Technol., 2014, 4(2), 428–434 CAS.
- Z. Wu, S. Ge, C. Ren, M. Zhang, A. Yip and C. Xu, Selective conversion of cellulose into bulk chemicals over Bronsted acid-promoted ruthenium catalyst: one-pot vs. sequential process, Green Chem., 2012, 14(12), 3336–3343 RSC.
- E. Girard, D. Delcroix and A. Cabiac, Catalytic conversion of cellulose to C2-C3 glycols by dual association of a homogeneous metallic salt and a perovskite-supported platinum catalyst, Catal. Sci. Technol., 2016, 6(14), 5534–5542 CAS.
- C. Chatterjee, F. Pong and A. Sen, Chemical conversion pathways for carbohydrates, Green Chem., 2015, 17(1), 40–71 RSC.
- A. M. Ruppert, K. Weinberg and R. Palkovits, Hydrogenolysis Goes Bio: From Carbohydrates and Sugar Alcohols to Platform Chemicals, Angew. Chem., Int. Ed., 2012, 51(11), 2564–2601 CrossRef CAS PubMed.
- X. Jin, J. Shen, W. Yan, M. Zhao, P. S. Thapa, B. Subramaniam and R. V. Chaudhari, Sorbitol Hydrogenolysis over Hybrid Cu/CaO-Al2O3 Catalysts: Tunable Activity and Selectivity with Solid Base Incorporation, ACS Catal., 2015, 5(11), 6545–6558 CrossRef CAS.
- Y. Jia and H. Liu, Mechanistic insight into the selective hydrogenolysis of sorbitol to propylene glycol and ethylene glycol on supported Ru catalysts, Catal. Sci. Technol., 2016, 6(19), 7042–7052 CAS.
- G. V. Ling, A. J. Driessen, A. C. Piet and J. C. Vlugter, Continuous Production of Glycerol by Catalytic High Pressure Hydrogenolysis of Sucrose, Ind. Eng. Chem. Prod. Res. Dev., 1970, 9(2), 210–212 Search PubMed.
- V. G. Matveeva, E. M. Sulman, O. V. Manaenkov, A. E. Filatova, O. V. Kislitza, A. I. Sidorov, V. Y. Doluda, M. G. Sulman and E. V. Rebrov, Hydrolytic hydrogenation of cellulose in subcritical water with the use of the Ru-containing polymeric catalysts, Catal. Today, 2017, 280(Part 1), 45–50 CrossRef CAS.
- H. A. Duarte, M. E. Sad and C. R. Apesteguía, Aqueous phase reforming of sorbitol on Pt/Al2O3: Effect of metal loading and reaction conditions on H2 productivity, Int. J. Hydrogen Energy, 2016, 41(39), 17290–17296 CrossRef CAS.
- R. Vijaya Shanthi, T. M. Sankaranarayanan, R. Mahalakshmy and S. Sivasanker, Fly ash based Ni catalyst for conversion of sorbitol into glycols, J. Environ. Chem. Eng., 2015, 3(3), 1752–1757 CrossRef CAS.
- J. Zhang, S. Wu, Y. Liu and B. Li, Hydrogenation of glucose over reduced Ni/Cu/Al hydrotalcite precursors, Catal. Commun., 2013, 35, 23–26 CrossRef CAS.
- D. K. Mishra, J.-M. Lee, J.-S. Chang and J.-S. Hwang, Liquid phase hydrogenation of d-glucose to d-sorbitol over the catalyst (Ru/NiO–TiO2) of ruthenium on a NiO-modified TiO2 support, Catal. Today, 2012, 185(1), 104–108 CrossRef CAS.
- H. Li, W. Wang and J. Fa Deng, Glucose Hydrogenation to Sorbitol over a Skeletal Ni-P Amorphous Alloy Catalyst (RANEY® Ni-P), J. Catal., 2000, 191(1), 257–260 CrossRef CAS.
- R. Beerthuis, G. Rothenberg and N. R. Shiju, Catalytic routes towards acrylic acid, adipic acid and ?-caprolactam starting from biorenewables, Green Chem., 2015, 17(3), 1341–1361 RSC.
- J.-W. Wang, C.-Y. Chen and Y.-M. Kuo, Effect of experimental parameters on the formation of chitosan–poly(acrylic acid) nanofibrous scaffolds and evaluation of their potential application as DNA carrier, J. Appl. Polym. Sci., 2010, 115(3), 1769–1780 CrossRef CAS.
- B. Katryniok, S. Paul, M. Capron and F. Dumeignil, Towards the Sustainable Production of Acrolein by Glycerol Dehydration, ChemSusChem, 2009, 2(8), 719–730 CrossRef CAS PubMed.
- B. Katryniok, S. Paul, V. Belliere-Baca, P. Rey and F. Dumeignil, Glycerol dehydration to acrolein in the context of new uses of glycerol, Green Chem., 2010, 12(12), 2079–2098 RSC.
- X. Li and Y. Zhang, Highly Efficient Process for the Conversion of Glycerol to Acrylic Acid via Gas Phase Catalytic Oxidation of an Allyl Alcohol Intermediate, ACS Catal., 2016, 6(1), 143–150 CrossRef CAS.
-
M. Dusselier and B. F. Sels, Selective Catalysis for Cellulose Conversion to Lactic Acid and Other α-Hydroxy Acids, in Selective Catalysis for Renewable Feedstocks and Chemicals, ed. K. M. Nicholas, Springer International Publishing, Cham, 2014, pp. 85–125 Search PubMed.
- P. Mäki-Arvela, I. L. Simakova, T. Salmi and D. Y. Murzin, Production of Lactic Acid/Lactates from Biomass and Their Catalytic Transformations to Commodities, Chem. Rev., 2014, 114(3), 1909–1971 CrossRef PubMed.
- M. Dusselier, P. Van Wouwe, A. Dewaele, E. Makshina and B. F. Sels, Lactic acid as a platform chemical in the biobased economy: the role of chemocatalysis, Energy Environ. Sci., 2013, 6(5), 1415–1442 CAS.
- Y. Fan, C. Zhou and X. Zhu, Selective Catalysis of Lactic Acid to Produce Commodity Chemicals, Catal. Rev.: Sci. Eng., 2009, 51(3), 293–324 CAS.
-
J. P. Lange, Production of acrylic acid, WO14108415, 2014.
- L. Prati and M. Rossi, Gold on Carbon as a New Catalyst for Selective Liquid Phase Oxidation of Diols, J. Catal., 1998, 176(2), 552–560 CrossRef CAS.
- Y. Ryabenkova, P. J. Miedziak, N. F. Dummer, S. H. Taylor, N. Dimitratos, D. J. Willock, D. Bethell, D. W. Knight and G. J. Hutchings, The Selective Oxidation of 1,2-Propanediol by Supported Gold-Based Nanoparticulate Catalysts, Top. Catal., 2012, 55(19), 1283–1288 CrossRef CAS.
- K. Mori, Y. Yamada and S. Sato, Catalytic dehydration of 1,2-propanediol into propanal, Appl. Catal., A, 2009, 366(2), 304–308 CrossRef CAS.
- D. Zhang, S. A. I. Barri and D. Chadwick, Dehydration of 1,2-propanediol to propionaldehyde over zeolite catalysts, Appl. Catal., A, 2011, 400(1–2), 148–155 CrossRef CAS.
- S. Sato, R. Takahashi, T. Sodesawa, H. Fukuda, T. Sekine and E. Tsukuda, Synthesis of α-hydroxyketones from 1,2-diols over Cu-based catalyst, Catal. Commun., 2005, 6(9), 607–610 CrossRef CAS.
- G. Peng, X. Wang, X. Chen, Y. Jiang and X. Mu, Zirconia-supported niobia catalyzed formation of propanol from 1,2-propanediol via dehydration and consecutive
hydrogen transfer, J. Ind. Eng. Chem., 2014, 20(5), 2641–2645 CrossRef CAS.
- H. Duan, D. Sun, Y. Yamada and S. Sato, Dehydration of 2,3-butanediol into 3-buten-2-ol catalyzed by ZrO2, Catal. Commun., 2014, 48(0), 1–4 CrossRef CAS.
- H. Duan, Y. Yamada and S. Sato, Selective dehydration of 2,3-butanediol to 3-buten-2-ol over ZrO2 modified with CaO, Appl. Catal., A, 2014, 487(0), 226–233 CrossRef CAS.
- L.-Z. Tao, S.-H. Chai, H.-P. Wang, B. Yan, Y. Liang and B.-Q. Xu, Comparison of gas-phase dehydration of propane polyols over solid acid–base catalysts, Catal. Today, 2014, 234(0), 237–244 CrossRef CAS.
- M. Chia and J. A. Dumesic, Liquid-phase catalytic transfer hydrogenation and cyclization of levulinic acid and its esters to [gamma]-valerolactone over metal oxide catalysts, Chem. Commun., 2011, 47(44), 12233–12235 RSC.
- M. Zabeti, K. B. Sai Sankar Gupta, G. Raman, L. Lefferts, S. Schallmoser, J. A. Lercher and K. Seshan, Aliphatic Hydrocarbons from Lignocellulose by Pyrolysis over Cesium-Modified Amorphous Silica Alumina Catalysts, ChemCatChem, 2015, 7(20), 3386–3396 CrossRef CAS.
- T. V. Andrushkevich, Heterogeneous Catalytic Oxidation of Acrolein to Acrylic Acid: Mechanism and Catalysts, Catal. Rev.: Sci. Eng., 1993, 35(2), 213–259 CAS.
- K. Schuh, W. Kleist, M. Høj, V. Trouillet, P. Beato, A. D. Jensen, G. R. Patzke and J.-D. Grunwaldt, Selective oxidation of propylene to acrolein by hydrothermally synthesized bismuth molybdates, Appl. Catal., A, 2014, 482, 145–156 CrossRef CAS.
- S. Endres, P. Kampe, J. Kunert, A. Drochner and H. Vogel, The influence of tungsten on structure and activity of Mo–V–W-mixed oxide catalysts for acrolein oxidation, Appl. Catal., A, 2007, 325(2), 237–243 CrossRef CAS.
- L. Giebeler, P. Kampe, A. Wirth, A. H. Adams, J. Kunert, H. Fuess and H. Vogel, Structural changes of vanadium–molybdenum–tungsten mixed oxide catalysts during the selective oxidation of acrolein to acrylic acid, J. Mol. Catal. A: Chem., 2006, 259(1–2), 309–318 CrossRef CAS.
- S. R. G. Carrazán, C. Martín, V. Rives and R. Vidal, Selective oxidation of isobutene to methacrolein on multiphasic molybdate-based catalysts, Appl. Catal., A, 1996, 135(1), 95–123 CrossRef.
- M. Ai, Oxidation of propane over V2O5–P2O5-based catalysts at relatively low temperatures, Catal. Today, 1998, 42(3), 297–301 CrossRef CAS.
- M. M. Lin, Selective oxidation of propane to acrylic acid with molecular oxygen, Appl. Catal., A, 2001, 207(1–2), 1–16 CrossRef CAS.
- T. Jekewitz, N. Blickhan, S. Endres, A. Drochner and H. Vogel, The influence of water on the selective oxidation of acrolein to acrylic acid on Mo/V/W-mixed oxides, Catal. Commun., 2012, 20, 25–28 CrossRef CAS.
- J.-P. Lange, Don't Forget Product Recovery in Catalysis Research—Check the Distillation Resistance, ChemSusChem, 2017, 10(1), 245–252 CrossRef CAS PubMed.
-
M. J. Schultz, T. P. Binder, N. Sudharsan and A. Hilaly, Separation of propionic acid from acrylic acid, WO2015031182A1, 2015.
- J.-P. Lange, Catalysis for biorefineries - performance criteria for industrial operation, Catal. Sci. Technol., 2016, 6(13), 4759–4767 CAS.
Footnote |
† Electronic supplementary information (ESI) available: XRD, BET analysis, GCMS, distillation resistance. See DOI: 10.1039/c7cy01416c |
|
This journal is © The Royal Society of Chemistry 2018 |
Click here to see how this site uses Cookies. View our privacy policy here.