DOI:
10.1039/C8RA03448F
(Paper)
RSC Adv., 2018,
8, 21745-21753
1,3,4-Thiadiazol derivative functionalized-Fe3O4@SiO2 nanocomposites as a fluorescent probe for detection of Hg2+ in water samples
Received
22nd April 2018
, Accepted 24th May 2018
First published on 13th June 2018
Abstract
5-Amino-1,3,4-thiadiazole-2-thiol was used to synthesize a novel fluorescent functionalizing group on a Fe3O4@SiO2 magnetic nanocomposite surface for detection of heavy metal ions in water samples. The prepared probe was characterized by using X-ray diffraction, transmission electron microscopy, Fourier transform infrared spectroscopy, and a vibrating sample magnetometer. Among various tested ions, the new nanocomposite responded to Hg2+ ions with an intense fluorescence “turn-off”. The limit of detection of the probe shows that it is sensitive to the minimum Hg2+ concentration of 48.7 nM. Theoretical calculations were done for estimating binding energies of the three possible bonding modes and the visualized molecular orbitals were presented.
Introduction
The widespread toxicological properties of mercury have made it one of the most dangerous pollutants in the environment. Mercury released from natural sources or human activities ends up in the atmosphere and in water. According to the U.S. Environmental Protection Agency (EPA), the anthropogenic mercury emission in the US was measured to be 1.2 tons in 2011.1,2 Methyl mercury, which usually bioaccumulates in fish, can cause serious neurotoxic damage to the body. On the other hand, the possible form of mercury in drinking water is inorganic mercury. The main target of inorganic mercury in the body is the kidneys and especially the proximal tubule where mercury is accumulated by either luminal uptake of Cys–S–Hg–S–Cys or by basolateral uptake.3 The limit of mercury(II) concentration in drinking water has been determined by the EPA to be 2 ppb.4
In order to ascertain the exact amount of mercury ions in drinking water, there are various analytical methods including atomic absorption spectroscopy,5 inductively coupled plasma-mass spectrometry,6 high performance liquid chromatography,7 and electrochemical sensing.8 However, these techniques suffer from complex and unhandy equipment which limits their on-site applications. Recently, fluorescent probes have emerged as a novel, selective, clean, simple, and portable alternative for detection of various chemical compounds in different mediums.9–13 Designing metal fluorescent probes is a challenging field of research since it needs a vast knowledge of metal coordination chemistry as well as photophysical mechanisms. Although designing new organic fluorophores is still of great importance, development of nanostructured substrates is more appealing since they serve a porous structure for hosting large amounts of fluorophore molecules and are conveniently separated after metal adsorption.14
The immobilization of organic fluorophores has been reported on certain nanosized substrates such as graphene quantum dots,15 carbon dots,16 Ag@SiO2,17 SiO2,18 MCM-41,19 SBA-15,20 and Fe3O4@SiO2.21 The privilege of easy separation with external magnetic field has made Fe3O4@SiO2 a superior choice. Many reports have already presented application of different fluorescence probes based on Fe3O4@SiO2 nanocomposites in detection and removal of heavy metals.11,12,22–28 Both conventional and novel organic ligands with two important characteristics are potential for this purpose: (i) possessing suitable functional groups on one side to react and attach to SiO2 surface either directly or through spacers such as (3-aminopropyl)triethoxysilane (APTES) or N-(2-amino ethyl)-3-aminopropyl trimetoxysilane (AEAPTES) (ii) having a fluorophore group which tends to coordinate with heavy metal and its fluorometric response changes after coordination. 1,3,4-thiadiazole has been known as a widely applied chemical compound in agricultural, pharmaceutical, and materials chemistry.29 The broad spectrum of its biological activities in terms of antibacterial, anticancer, antidepressant, etc. has made it an attractive compound for biologists and chemists. The special structure of thiadiazole class of components enables keto/enol tautomerism which is the origin of fluidity of biological membranes,30,31 crystal polymorphism, solvatomorphism effects, and interactions in lipid membranes.32,33 Moreover, the dual fluorescence effect of 1,3,4-thiadiazole is an interesting phenomena which is very important in understanding protein interactions of this molecule in biological systems.34
Recently, our group reported the successful design of two novel Fe3O4@SiO2 fluorescence probes with precise detection ability of mercury ion in water.35,36 In continuation of our previous works, here, a novel fluorescent probe is designed and grafted on the Fe3O4@SiO2 surface. A thiadiazole derivative is synthesized and applied as the fluorophore functionalizing group on Fe3O4@SiO2 nanocomposites and is used for detection of Hg2+ in drinking water. 5-Amino-1,3,4-thiadiazole-2-thiol (ATT) ligand which is used as a starting material in this work, has already been reported as a potential silica modifier for sorption of different heavy metals including Hg2+.37 The –SH site on the aromatic ring in this molecule has provided an excellent coordination ability to soft heavy metals.
Experimental
Characterization
X-ray diffraction (XRD) patterns were recorded by a Philips-X'PertPro, X-ray diffractometer using Ni-filtered Cu Kα radiation at scan range of 10° < 2θ < 80°. Scanning electron microscopy images were obtained on LEO-1455VP. Room temperature photoluminescence properties were studied on a Perkin-Elmer (LS 55) Mvv fluorescence spectrophotometer. Fourier transform infrared (FT-IR) spectra were recorded on Magna-IR, spectrometer 550 Nicolet with 0.125 cm−1 resolution in KBr pellets in the range of 400–4000 cm−1. TEM images were taken on an EM208S Philips transmission electron microscope with an accelerating voltage of 100 kV. For TEM analysis, the nanoparticle powder was dispersed in ethanol by ultrasonic bath. Then a drop of the dispersion was placed on the TEM grid and was dried in the vacuum oven and then it was used for TEM imaging. Room temperature magnetic properties were investigated using a vibrating sample magnetometer (VSM), made by Meghnatis Daghigh Kavir Company (Iran) in an applied magnetic field sweeping between ±10
000 Oe.
Synthesis of thiadiazol-based ligand
5-(4-Vinylbenzylidene)amino-1,3,4-thiadiazole-2-thiol (VBYT) (3) was prepared using ATT by the classical Schiff base reaction. First, terephthaldialdehyde (TPAL) (1 mmol) was suspended in 10 mL of methanol and added to 5-amino-1,3,4-thiadiazole-2-thiol (1 mmol) and the solution was stirred under reflux at 100 °C for 1 h (VBYT, see Scheme 1). Then, AEAPTES (1 mmol) was added to this solution and the reaction mixture was stirred under reflux at 60 °C for another 1 h. The precipitate was filtered off and recrystallized in DMF. The red product (VBYT–AEAPTES, see Scheme 2) was then dried at room temperature. The progress of the reaction was checked by thin layer chromatography (TLC).
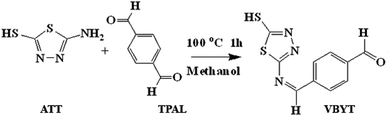 |
| Scheme 1 Synthetic procedure of VBYT. | |
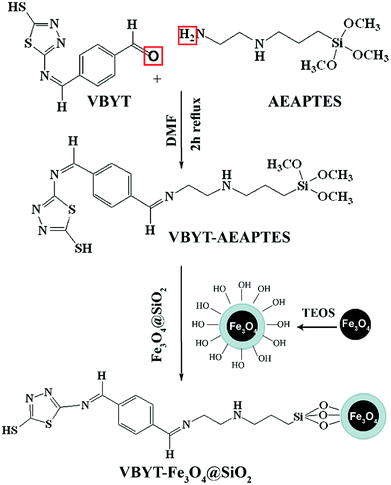 |
| Scheme 2 The procedure for synthesis of VBYT-Fe3O4@SiO2. | |
Synthesis of magnetic Fe3O4@SiO2 nanoparticles (NPs)
The Fe3O4-coated SiO2 nanoparticles were synthesized by a similar procedure developed by Dong et al.38 At first, for degassing the flask, the deionized water was exposed to nitrogen gas for 10 min. Then, Fe(NO3)3·9H2O (8.12 g, 0.020 mol) and FeCl2·4H2O (2.00 g, 0.010 mol) and 1 mL oleic acid were dissolved in 30 mL deionized water at 90 °C under nitrogen atmosphere and vigorous mechanical stirring (1000 rpm). Then, 20 mL of NH4OH (28 wt%) was quickly injected into the reaction mixture in one portion and it immediately changed to black. The mixture continued under this condition (90 °C) for 2.5 h and the colloid solution was cooled to room temperature. After cooling, it was suspended in chloroform.
For preparation of Fe3O4@SiO2 nanoparticles, a modified method reported by Zhu et al.23 was used. 25 mg of Fe3O4 nanoparticles was added into 40 mL of cyclohexane at room temperature by ultrasonic dispersion. Triton X-100 (9 g), octanol (8 mL) and H2O (1.7 mL) were then added with stirring to form water-in-oil microemulsion. The reaction mixture was vigorously stirred for 15 min. Then, 1 mL of TEOS was added slowly into the solution. The reaction vessel was stirred on a shaker for 1.5 h at room temperature and then, 1 mL aqueous ammonia (28 wt%) was added into the reaction. The stirring continued for 24 h at room temperature to form the Fe3O4@SiO2 core–shell nanoparticles. The Fe3O4@SiO2 nanoparticles were isolated via centrifugation at 4000 rpm and washed with ethanol for five times. The obtained dark grey product was allowed to dry at 80 °C.
Preparation of VBYT-Fe3O4@SiO2
First, 30 mg of dried Fe3O4@SiO2 and 30 mg (1 mmol) of VBYT–AEAPTES ligand were suspended in 20 mL of DMF. DMF was used as solvent here, because the solubility test for VBYT–AEAPTES ligand showed that it is completely soluble in it. The mixture was refluxed for 12 h at 60 °C. The products were collected by filtration and repeatedly washed with DMF and then ethanol several times.
Preparation of the fluorometric metal ion titration solution
The fluorometric measurements of metal ions were performed in phosphate-buffered saline (PBS) solutions (0.02 M, pH = 7.0). 0.03 g of the functionalized Fe3O4@SiO2 nanoparticles were dispersed in 3 mL of 1 × 10−6 M of different metal nitrate (Hg2+, Na+, Mg2+, Ca2+, Al3+, Cr3+, Zn2+, Ni2+, and Fe3+) aqueous PBS buffer solutions for testing the effect of metal type on fluorescence response of the as-prepared probe. The performance of the prepared nanocomposite was also tested in tap water of the chemistry laboratory located in University of Zabol, as a real sample.
Calculations
The molecular structure of VBYT–AEAPTES and its interaction with Hg2+ in three different bonding modes in the ground state were optimized on the basis of density function theory (DFT) at the Becke3–Lee–Yang–Parr (B3LYP) (with LanL2DZ basis) and by means of visual inspection using the GAUSSVIEW program (Version 5.0). All the calculations were performed using the GAUSSIAN 09 software package.39 The population analysis was performed by natural bond orbital (NBO) method using NBO program40 implemented under Gaussian 09 program package.41
Results and discussion
Characterization of as-prepared samples
The phase of the samples were characterized by their X-ray diffraction patterns. Fig. 1a and b show the XRD patterns of Fe3O4 and Fe3O4@SiO2 nanoparticles, respectively. Fig. 1a shows that the pattern corresponds to pure cubic Fe3O4 with JCPDS card no.: 19-629. The XRD pattern of Fe3O4@SiO2 consists of SiO2 and Fe3O4 phases (Fig. 1b). The broad band at ca. 2θ = 20–30° is attributed to the amorphous phase of SiO2. The peaks corresponding to Fe3O4 in Fe3O4@SiO2 (Fig. 1b) are weaker than those in pure Fe3O4 which shows that Fe3O4 is covered with SiO2 shell.
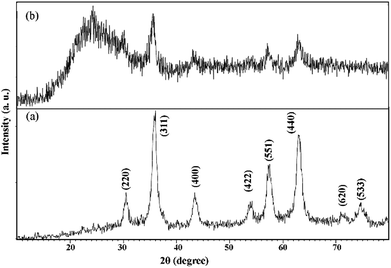 |
| Fig. 1 XRD patterns of (a) Fe3O4 nanoparticles and (b) Fe3O4@SiO2. | |
In order to study the surface chemistry of the samples, FT-IR spectroscopy was used. Fig. 2a–c show the FT-IR spectra of VBYT–AEAPTES ligand, Fe3O4@SiO2, and VBYT-Fe3O4@SiO2, respectively. In Fig. 2a, the weak peak at 712.86 cm−1 is referred to C–S stretching vibration. The peak at 1060.18 cm−1 is attributed to Si–O stretching vibration. The characteristic bands at 1610.43 and 1554.68 cm−1 are attributed to C
N and C
C stretching vibrations, respectively. The extremely weak band at 2598.26 cm−1 is for S–H stretching vibration.42 The appeared peak at 2925.59 cm−1 is assigned to sp3 C–H stretching of alkane chain. A single vibration at 3342.17 cm−1 is assigned to secondary amine in AEAPTES counterpart.
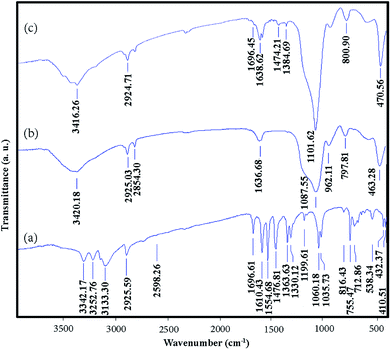 |
| Fig. 2 FT-IR spectra of (a) VBYT–AEAPTES ligand, (b) Fe3O4@SiO2, and (c) VBYT-Fe3O4@SiO2. | |
In Fig. 2b and c, the main appeared peaks correspond to Fe3O4 cores and SiO2 shells. The peaks around 590 cm−1 and 470 cm−1 in Fig. 2b and c are assigned to the Fe–O vibration of magnetite core. The displayed bands around 3400 cm−1 and 1636 cm−1 are due to the stretching and bending vibration of remained H2O and silanol O–H groups, respectively. In both spectra, the broad and strong peak around 1100 cm−1 is assigned to the overlapped stretching vibration of Si–O–Si bonds. Two characteristic peaks at 2854 cm−1 and 2925 cm−1 are referred to the symmetric and asymmetric stretching vibration of C–H of the alkyl groups. In Fig. 2c, weak peaks in the range of 1000–1700 cm−1 show the attachment of VBYT–AEAPTES ligand on the surface of Fe3O4@SiO2 nanocomposites.
For characterization of the prepared core–shell nanocomposites, TEM analysis was performed on images of VBYT-Fe3O4@SiO2 sample and the results were shown in Fig. 3a and b. It can be seen that the formed nanoparticles are very tiny and are partly agglomerated because of the magnetic properties of the nanocomposites. A higher magnification of one separate nanoparticle, shown in Fig. 3b-inset, clearly shows the core–shell structure of the nanocomposite. The diameter of the nanocomposite particle is approximately 32 nm with a core with the diameter of 11 nm. Therefore, the SiO2 coverage layer around Fe3O4 core is about 21 nm.
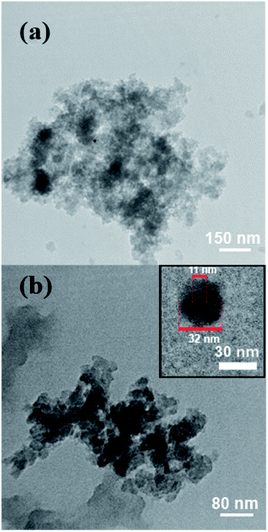 |
| Fig. 3 (a) and (b) TEM images of VBYT-Fe3O4@SiO2 from two different parts of the samples. Inset: one separate core–shell VBYT-Fe3O4@SiO2 nanoparticle. | |
For studying the magnetic properties of the Fe3O4 and VBYT-Fe3O4@SiO2 a vibrating sample magnetometer was used at 300 K (Fig. 4). The measured saturation magnetizations (Ms) are 50.33 emu g−1 and 2.89 emu g−1 for Fe3O4 and VBYT-Fe3O@SiO2, respectively that are shown in Fig. 4a. This decrease in magnetic properties is due to coverage of the Fe3O4 cores by SiO2 and VBYT layers. Both samples are superparamagnetic because they almost show no remanence and coercivity. Although the magnetization of VBYT-Fe3O@SiO2 is small, it is easily separated from the solution by placing a magnet, within 3 minutes (Fig. 4b). This results show that the prepared material is potential for water purification applications since it is efficiently absorbed by a simple magnet.
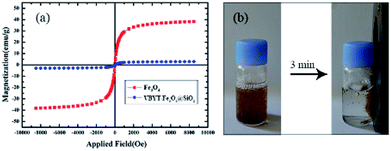 |
| Fig. 4 (a) The magnetic hysteresis loops of Fe3O4@SiO and VBYT-Fe3O4@SiO2 (b) the separation of VBYT-Fe3O4@SiO2 in aqueous solution after 3 minutes. | |
Testing the fluorescence properties of the nanocomposite in response to metal absorption
In order to test the response of the prepared sample against different metal ions, the fluorescence spectra of the nanocomposite were recorded in presence and absence of the some important metal ions including Ni2+, Hg2+, Zn2+, Mg2+, Al3+, Na+, Ca2+, Fe3+, and Cr3+. Fig. 5 shows the results of the fluorescence test after exciting the samples at λexc = 268 nm. The λmax emission (λmax em) of the free receptor (FR) i.e. VBYT-Fe3O4@SiO2 dispersed in buffer solution in absence of metal ions is at 409 nm. After addition of fixed amounts of Hg2+, Cr3+, Ni2+, Zn2+, Mg2+, Fe3+, Al3+, Ca2+, and Na+ the intensity of the maximum peak was either unchanged or slightly tuned in case of Al3+, Ni2+ and Ca2+. Moreover, almost no peak shift was observed at λmax em compared to the FR. For Zn2+, the peak at 340 nm was intensified that probably could be attributed to its different bonding mode compared with other metal ions which prohibits the photoinduced electron transfer (PET) mechanism and results in a “turn-on” switching.43,44 In case of Hg2+, a significant quenching (about 1100 units) was observed for λmax em peak at 409 nm. Therefore, by comparing the response of VBYT-Fe3O4@SiO2 nanocomposite to different ions, it was shown that the prepared material could be a good candidate for detection of Hg2+ ions. Similar results have been reported for application of silica gel modified with ATT by Tzvetkova et al. They indicated that among some heavy metals including Cu(II), Co(II), Ni(II), Cd(II), Pb(II) and Hg(II), the modified silica gel shows the highest sorption capacity against Hg(II).37 Certain factors such as suitable coordination geometry of ligand and ion, nitrogen affinity, and ion size and charge are effective in high selectivity of an ion.35,45 In continue, more tests were conducted in order to show the potential application of the nanocomposite on detection of Hg2+ ions in water samples.
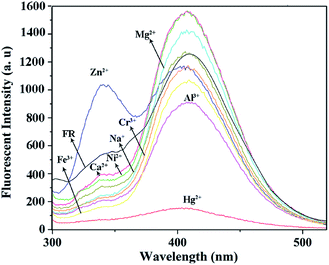 |
| Fig. 5 The fluorescence spectra of VBYT-Fe3O4@SiO2 in presence of various metal ions (λexc = 268 nm). | |
To elucidate the detection potential of VBYT-Fe3O4@SiO2 against Hg2+, a titration experiment was performed and the results were shown in Fig. 6. For this purpose 0.03 g of nanocomposite was dispersed in 10 mL of 0, 0.1, 0.3, 0.5, 1, 3, 5, 10, 20, and 30 × 10−6 M of Hg2+ in PBS buffer solution (pH = 7, water). As can be seen in Fig. 6a, with increasing Hg2+ concentration, two appeared peaks at 409 nm (denoted as blue emission) and 351 nm (denoted as UV emission) are gradually quenched. It is clearly seen that the blue emission is quenched faster than the UV emission. It proves that the part of the fluorophore molecule which is responsible for the UV emission, is first involved in PET mechanism from Hg2+ as the receptor. On the other hand, it seems that the receptor is directly bonded to the group(s) responsible for the blue emission and is transferring electron to this group(s) through PET mechanism44 which results in a faster “turn-off”. Fig. 6b shows the graph of fluorescence intensity versus Hg2+ concentration. It is seen that in the range of 0.1–0.5 μM of Hg2+, there is a good linear relationship in the graph with R2 = 0.9833. The regression equation is F = −1896.2C (μM Hg2+) + 1225.5 for the intensity change of the red shift with increasing Hg2+ concentration. The limit of detection (LOD) of the probe was calculated to be 48.7 nM for Hg2+ detection which satisfies the U.S. EPA (the U.S. Environmental Protection Agency) limits (∼2 ppb) of Hg2+ detection in drinking water and is a good record compared to some other reports.38,46–48
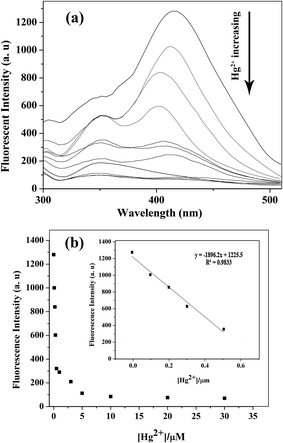 |
| Fig. 6 (a) Fluorescence emission spectra (λexc = 268 nm) of VBYT-Fe3O4@SiO2 (1 × 10−6 M) in the presence of increasing concentrations of Hg2+ (0–30 μM). (b) The change of the emission intensities at 409 nm with increasing Hg2+ concentration. | |
The selectivity of the synthesized probe was tested in presence of some interfering ions. Fig. 7 shows the fluorescence changes of VBYT-Fe3O4@SiO2 by treatment of 10−6 M of the interfering ions in 10 mL PBS buffer solution containing 0.03 g of the dispersed nanocomposite. Moreover, the interfering ions were tested in presence of the same concentration of Hg2+. It is shown that the tested metal ions (Na+, K+, Mg2+, Al3+, Ca2+, Cd2+, Ni2+, Co2+, Zn2+, Cu2+, Fe3+, Pb2+, Ag+) have small or no interference with Hg2+ ions and this ion can be easily detected in presence of either of the shown elements. In order to have an account of the behaviour of the designed probe in the real sample, the fluorescence spectra of FR as well as FR plus Hg2+ ions were tested in drinking tap water of University of Zabol as a real sample. The results shown in Fig. 7 (in blue columns) show that very similar behaviour is also observed in the real sample. These results suggest that VBYT-Fe3O4@SiO2 is a good candidate to be applied for detection of Hg2+ with high selectivity against other metal ions in a real aqueous samples.
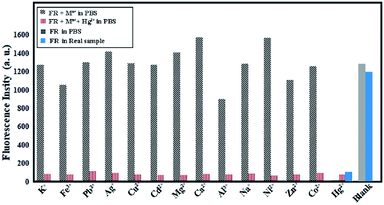 |
| Fig. 7 Fluorescence response of VBYT-Fe3O4@SiO2 to various interfering cations in aqueous solution. The concentrations of all the tested ions were 1 μM. The intensities were recorded at 409 nm, excitation at 268 nm, FR: free receptor. | |
To test the regeneration ability of the probe, VBYT-Fe3O4@SiO2 was tested by using excess amount of NaI which is able to remove Hg2+ from the chemosensor surface. Fig. 8 shows the results of the regeneration test which proves that the fluorescence properties of VBYT-Fe3O4@SiO2 is reversible and the probe still has its ability after removing the content of Hg2+ ions by NaI.
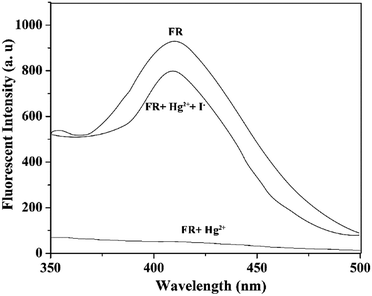 |
| Fig. 8 Fluorescence spectra of top: FR (VBYT-Fe3O4@SiO2), middle: FR plus Hg2+ (1 × 10−6 M), and, bottom: FR and Hg2+ plus I− (excess) in aqueous solution. | |
In the present study, interactions of the Hg+2 ions with positions on VBYT–AEAPTES ligand which encompass nitrogen and sulphur atoms were considered. Results indicate that the Hg+2 ions interact preferably with the ligand through atoms N28, N31, and S37 which are labelled in Scheme 3. The binary complexes which was formed through interactions of the Hg+2 ions with the mentioned atoms on the ligand are denoted as A, B, and C, respectively (see Scheme 3). The order of the binding energies of these complexes (in kcal mol−1) is: B (217.61) < A (220.20) < C (227.75). Also, gaps between energies of highest occupied molecular orbital (HOMO) and lowest unoccupied molecular orbital (LUMO) in ligand and complexes were calculated. The results point out that energy gap in the ligand is 2.857 eV and interactions of Hg+2 ions with the ligand lead to decreasing the energy gaps in the complexes. The order of energy gaps in the complexes (in eV) is: B (0.423) < A (0.432) < C (0.478) which is in agreement with the order of the binding energies.
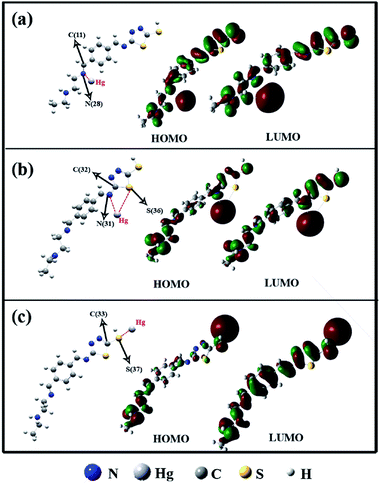 |
| Scheme 3 Molecular orbital structure of HOMO and LUMO of different bonding modes including (a) N28, (b) N31 and S36, and (c) S37. | |
The NBO analysis was performed on the mentioned complexes to obtain better insight regarding the order of binding energies. Donor–acceptor interaction energy (E2) values between all donors and acceptors were studied. Results indicate that the order of E2 values (in kcal mol−1) of interactions between lone pair (Lp) of Hg and antibonding (BD*) orbitals of X–Y (X = N28, N31, and S37; Y = C11, C32, and C33) in the complexes is: C (0.36) < A (0.51) < B (0.74). In fact, decrease of anti-bonding feature of X–Y bonds through interaction of Hg+2 ions with VBYT–AEAPTES ligand leads to increase of binding energies of the complexes.
Based on the abovementioned theoretical results, the fluorescence “turn-off” behaviour of VBYT-Fe3O4@SiO2 nanocomposites is depicted in Scheme 4. As can be seen in Scheme 4a, two different parts of the VBYT molecule including benzylidene and thiadiazole are responsible for UV and blue emissions, respectively. After addition of low concentrations of Hg2+, S37 site coordinated to the entered metal ions due to its higher binding energy. The coordinated Hg2+ ion may act as receptor and transfers electron to the fluorophore via PET mechanism causing the quenching of the blue emission (Scheme 4b). Another possible mechanism for quenching the UV band could be the enhanced intersystem crossing (ISC) to the triplet state due to “heavy ion effect”.49,50 By increasing the concentration of the Hg2+ ions in the solution and saturation of S37 sites in VBYT molecule, gradually N28–Hg2+ bond with high binding energy is formed and again, by PET mechanism, the UV emission turns off, as well. Quenching of blue emission due to the “heavy ion effect” is less likely since the sensor is already significantly spin–orbit coupled due to the already coordinated Hg2+ ion.44
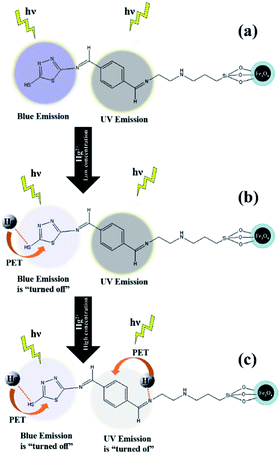 |
| Scheme 4 The proposed mechanism for “turn-off” behavior of UV and blue emissions of VBYT-Fe3O4@SiO2 after addition of Hg2+ ions: (a) free receptor (b) after addition of low concentration of Hg2+, (c) after addition of high concentration of Hg2+. | |
Conclusions
VBYT-Fe3O4@SiO2 nanocomposites were synthesized by using ATT as a starting material. The characterizations showed successful synthesis of Fe3O4@SiO2 and its functionalization with VBYT ligand. The prepared nanocomposite was easily separated from the solution by an external magnet. The fluorescence response of the nanocomposite against different ions showed that the blue fluorescence peak is easily quenched by adding Hg2+ ions. The sensitivity of the probe was calculated by titration tests and it was shown that the limit of detection is 48.7 nm and the fluorescence intensity diagram against Hg2+ concentration showed a good linear relationship in the range of 0.1–0.5 μM. Theoretical calculations showed that the binding energies of S37, N28, and N31 bonds with Hg2+ ion has an order of S37 > N28 > N31. The presence of various active sites for coordination to mercury atoms makes this detector potential adsorbent for removing heavy metal atoms with high capacity. The absorbing application of the designed nanomaterial is the subject of our further research work.
Conflicts of interest
There are no conflicts to declare.
Acknowledgements
This work was supported by University of Zabol.
Notes and references
- U.S.E.U.S.E.P. Agency, 2011 National Emissions Inventory, technical support document. Draft report, Version 2, 2015 Search PubMed.
- U.S. EPA, Data from the 2011 National Emissions Inventory Version 1, 2014 Search PubMed.
- J.-D. Park and W. Zheng, Human exposure and health effects of inorganic and elemental mercury, Journal of Preventive Medicine & Public Health, 2012, 45(6), 344 Search PubMed.
- U. EPA, Mercury Update: Impact on Fish Advisories, EPA Fact Sheet EPA-823-F-01-011, 2001 Search PubMed.
- M. Ruiz-de-Cenzano, A. Rochina-Marco, M. L. Cervera and M. de la Guardia, Speciation of methylmercury in market seafood by thermal degradation, amalgamation and atomic absorption spectroscopy, Ecotoxicol. Environ. Saf., 2014, 107, 90–96 CrossRef PubMed.
- H. Cheng, C. Wu, L. Shen, J. Liu and Z. Xu, Online anion exchange column preconcentration and high performance liquid chromatographic separation with inductively coupled plasma mass spectrometry detection for mercury speciation analysis, Anal. Chim. Acta, 2014, 828, 9–16 CrossRef PubMed.
- S. S. de Souza, A. D. Campiglia and F. Barbosa, A simple method for methylmercury, inorganic mercury and ethylmercury determination in plasma samples by high performance liquid chromatography–cold-vapor-inductively coupled plasma mass spectrometry, Anal. Chim. Acta, 2013, 761, 11–17 CrossRef PubMed.
- N. Wang, M. Lin, H. Dai and H. Ma, Functionalized gold nanoparticles/reduced graphene oxide nanocomposites for ultrasensitive electrochemical sensing of mercury ions based on thymine–mercury–thymine structure, Biosens. Bioelectron., 2016, 79, 320–326 CrossRef PubMed.
- Z. Liu, W. He and Z. Guo, Metal coordination in photoluminescent sensing, Chem. Soc. Rev., 2013, 42(4), 1568–1600 RSC.
- M. H. Keefe, K. D. Benkstein and J. T. Hupp, Luminescent sensor molecules based on coordinated metals: a review of recent developments, Coord. Chem. Rev., 2000, 205(1), 201–228 CrossRef.
- X. Peng, Y. Wang, X. Tang and W. Liu, Functionalized magnetic core–shell Fe3O4@SiO2 nanoparticles as selectivity-enhanced chemosensor for Hg(II), Dyes Pigm., 2011, 91(1), 26–32 CrossRef.
- M. Shao, F. Ning, J. Zhao, M. Wei, D. G. Evans and X. Duan, Preparation of Fe3O4@SiO2@Layered Double Hydroxide Core–Shell Microspheres for Magnetic Separation of Proteins, J. Am. Chem. Soc., 2012, 134(2), 1071–1077 CrossRef PubMed.
- S. Thatai, P. Khurana, J. Boken, S. Prasad and D. Kumar, Nanoparticles and core–shell nanocomposite based new generation water remediation materials and analytical techniques: a review, Microchem. J., 2014, 116, 62–76 CrossRef.
- Y. Zhou, L. Tang, G. Zeng, C. Zhang, Y. Zhang and X. Xie, Current progress in biosensors for heavy metal ions based on DNAzymes/DNA molecules functionalized nanostructures: a review, Sens. Actuators, B, 2016, 223, 280–294 CrossRef.
- F. Wang, Z. Gu, W. Lei, W. Wang, X. Xia and Q. Hao, Graphene quantum dots as a fluorescent sensing platform for highly efficient detection of copper(II) ions, Sens. Actuators, B, 2014, 190, 516–522 CrossRef.
- G. E. LeCroy, S. K. Sonkar, F. Yang, L. M. Veca, P. Wang, K. N. Tackett, J.-J. Yu, E. Vasile, H. Qian and Y. Liu, Toward structurally defined carbon dots as ultracompact fluorescent probes, ACS Nano, 2014, 8(5), 4522–4529 CrossRef PubMed.
- H.-S. Wang, C. Wang, Y.-K. He, F.-N. Xiao, W.-J. Bao, X.-H. Xia and G.-J. Zhou, Core–shell Ag@ SiO2 nanoparticles concentrated on a micro/nanofluidic device for surface plasmon resonance-enhanced fluorescent detection of highly reactive oxygen species, Anal. Chem., 2014, 86(6), 3013–3019 CrossRef PubMed.
- Y. Ding, W. Zhu, Y. Xu and X. Qian, A small molecular fluorescent sensor functionalized silica microsphere for detection
and removal of mercury, cadmium, and lead ions in aqueous solutions, Sens. Actuators, B, 2015, 220, 762–771 CrossRef.
- G. Jha, N. Anoop, A. Rahaman and M. Sarkar, Fluoride ion sensing in aqueous medium by employing nitrobenzoxadiazole-postgrafted mesoporous silica nanoparticles (MCM-41), Phys. Chem. Chem. Phys., 2015, 17(5), 3525–3533 RSC.
- Z. Dong, X. Tian, Y. Chen, J. Hou and J. Ma, Rhodamine group modified SBA-15 fluorescent sensor for highly selective detection of Hg2+ and its application as an INHIBIT logic device, RSC Adv., 2013, 3(7), 2227–2233 RSC.
- X. Guo, F. Mao, W. Wang, Y. Yang and Z. Bai, Sulfhydryl-modified Fe3O4@SiO2 core/shell nanocomposite: synthesis and toxicity assessment in vitro, ACS Appl. Mater. Interfaces, 2015, 7(27), 14983–14991 Search PubMed.
- U. Jeong, H. H. Shin and Y. Kim, Functionalized magnetic core–shell Fe@SiO2 nanoparticles as recoverable colorimetric sensor for Co2+ ion, Chem. Eng. J., 2015, 281, 428–433 CrossRef.
- B. Zhu, J. Zhao, H. Yu, L. Yan, Q. Wei and B. Du, Naphthalimide-functionalized Fe3O4@SiO2 core/shell nanoparticles for selective and sensitive adsorption and detection of Hg2+, Chem. Eng. J., 2013, 219, 411–418 CrossRef.
- L. Wang, J. Yan, W. Qin, W. Liu and R. Wang, A new rhodamine-based single molecule multianalyte (Cu2+, Hg2+) sensor and its application in the biological system, Dyes Pigm., 2012, 92(3), 1083–1090 CrossRef.
- C. Wang, S. Tao, W. Wei, C. Meng, F. Liu and M. Han, Multifunctional mesoporous material for detection, adsorption and removal of Hg2+ in aqueous solution, J. Mater. Chem., 2010, 20(22), 4635–4641 RSC.
- L. Sun, Y. Li, M. Sun, H. Wang, S. Xu, C. Zhang and Q. Yang, Porphyrin-functionalized Fe3O4@SiO2 core/shell magnetic colorimetric material for detection, adsorption and removal of Hg2+ in aqueous solution, New J. Chem., 2011, 35(11), 2697–2704 RSC.
- Y. Liu, R. Fu, Y. Sun, X. Zhou, S. A. Baig and X. Xu, Multifunctional nanocomposites Fe3O4@ SiO2–EDTA for Pb(II) and Cu(II) removal from aqueous solutions, Appl. Surf. Sci., 2016, 369, 267–276 CrossRef.
- X. Zhang, Y. Zhang, X. Zhang, S. Li and Y. Huang, Nitrogen rich core–shell magnetic mesoporous silica as an effective adsorbent for removal of silver nanoparticles from water, J. Hazard. Mater., 2017, 337, 1–9 CrossRef PubMed.
- Y. Hu, C.-Y. Li, X.-M. Wang, Y.-H. Yang and H.-L. Zhu, 1,3,4-Thiadiazole: synthesis, reactions, and applications in medicinal, agricultural, and materials chemistry, Chem. Rev., 2014, 114(10), 5572–5610 CrossRef PubMed.
- D. M. Kamiński, A. A. Hoser, M. Gagoś, A. Matwijczuk, M. Arczewska, A. Niewiadomy and K. Woźniak, Solvatomorphism of 2-(4-fluorophenylamino)-5-(2,4-dihydroxybenzeno)-1,3,4-thiadiazole chloride, Cryst. Growth Des., 2010, 10(8), 3480–3488 Search PubMed.
- A. Matwijczuk, D. Karcz, R. Walkowiak, J. Furso, B. Gładyszewska, S. Wybraniec, A. Niewiadomy, G. P. Karwasz and M. Gagoś, Effect of Solvent Polarizability on the Keto/Enol Equilibrium of Selected Bioactive Molecules from the 1,3,4-Thiadiazole Group with a 2,4-Hydroxyphenyl Function, J. Phys. Chem. A, 2017, 121(7), 1402–1411 CrossRef PubMed.
- A. A. Hoser, D. M. Kamiński, A. Matwijczuk, A. Niewiadomy, M. Gagoś and K. Woźniak, On polymorphism of 2-(4-fluorophenylamino)-5-(2,4-dihydroxybenzeno)-1,3,4-thiadiazole (FABT) DMSO solvates, CrystEngComm, 2013, 15(10), 1978–1988 RSC.
- D. M. Kamiński, A. Matwijczuk, D. Pociecha, E. Górecka, A. Niewiadomy, M. Dmowska and M. Gagoś, Effect of 2-(4-fluorophenylamino)-5-(2,4-dihydroxyphenyl)-1,3,4-thiadiazole on the molecular organisation and structural properties of the DPPC lipid multibilayers, Biochim. Biophys. Acta, Biomembr., 2012, 1818(11), 2850–2859 CrossRef PubMed.
- A. Matwijczuk, D. Kamiński, A. Górecki, A. Ludwiczuk, A. Niewiadomy, S. Maćkowski and M. Gagoś, Spectroscopic Studies of Dual Fluorescence in 2-((4-Fluorophenyl)amino)-5-(2,4-Dihydroxybenzeno)-1,3,4-Thiadiazole, J. Phys. Chem. A, 2015, 119(44), 10791–10805 CrossRef PubMed.
- N. Mir, A. Heidari, H. Beyzaei, S. Mirkazehi-Rigi and P. Karimi, Detection of Hg2+ in aqueous solution by pyrazole derivative-functionalized Fe3O4@SiO2 fluorescent probe, Chem. Eng. J., 2017, 327, 648–655 CrossRef.
- A. Heidari and N. Mir, 4-Acetamidobenzaldehyde-Functionalized Fe3O4@SiO2 Fluorescent Nanocomposite Probe for Detection of Hg2+ in Aqueous Solution, J. Fluoresc., 2017, 27(2), 659–667 CrossRef PubMed.
- P. Tzvetkova, P. Vassileva and R. Nickolov, Modified silica gel with 5-amino-1,3,4-thiadiazole-2-thiol for heavy metal ions removal, J. Porous Mater., 2010, 17(4), 459–463 CrossRef.
- Z. Dong, X. Tian, Y. Chen, Y. Guo and J. Ma, Dansyl derivative functionalized Fe3O4@SiO2 fluorescent probe for detection and removal of Hg2+ in aqueous solution, RSC Adv., 2013, 3(4), 1082–1088 RSC.
- M. Frisch, G. Trucks, H. B. Schlegel, G. Scuseria, M. Robb, J. Cheeseman, G. Scalmani, V. Barone, B. Mennucci, G. Petersson, Gaussian 09, revision a. 02, Gaussian, Inc., Wallingford, CT 200, 2009 Search PubMed.
- E. Glendening, A. Reed, J. Carpenter and F. Weinhold, NBO Version 3.1, TCI, University of Wisconsin, Madison 65, 1998 Search PubMed.
- A. E. Reed, L. A. Curtiss and F. Weinhold, Intermolecular interactions from a natural bond orbital, donor–acceptor viewpoint, Chem. Rev., 1988, 88(6), 899–926 CrossRef.
- C. L. Garcia and J. A. Lercher, Adsorption and surface chemistry of light thiols on Na-ZSM5 and H-ZSM5, J. Phys. Chem., 1991, 95(26), 10729–10736 CrossRef.
- L. N. Neupane, J.-Y. Park, J. H. Park and K.-H. Lee, Turn-on Fluorescent Chemosensor Based on an Amino Acid for Pb(II) and Hg(II) Ions in Aqueous Solutions and Role of Tryptophan for Sensing, Org. Lett., 2013, 15(2), 254–257 CrossRef PubMed.
- A. P. De Silva, H. N. Gunaratne, T. Gunnlaugsson, A. J. Huxley, C. P. McCoy, J. T. Rademacher and T. E. Rice, Signaling recognition events with fluorescent sensors and switches, Chem. Rev., 1997, 97(5), 1515–1566 CrossRef PubMed.
- X.-C. Fu, J. Wu, C.-G. Xie, Y. Zhong and J.-H. Liu, Rhodamine-based fluorescent probe immobilized on mesoporous silica microspheres with perpendicularly aligned mesopore channels for selective detection of trace mercury(II) in water, Anal. Methods, 2013, 5(10), 2615–2622 RSC.
- H. Wang, Y. Li, S. Xu, Y. Li, C. Zhou, X. Fei, L. Sun, C. Zhang, Y. Li, Q. Yang and X. Xu, Rhodamine-based highly sensitive colorimetric off-on fluorescent chemosensor for Hg2+ in aqueous solution and for live cell imaging, Org. Biomol. Chem., 2011, 9(8), 2850–2855 Search PubMed.
- Y. Xu, Y. Zhou, W. Ma, S. Wang and S. Li, Functionalized magnetic core–shell Fe3O4@SiO2 nanoparticles for sensitive detection and removal of Hg2+, J. Nanopart. Res., 2013, 15(6), 1–9 CrossRef.
- C.-l. Fang, J. Zhou, X.-j. Liu, Z.-h. Cao and D.-h. Shangguan, Mercury(II)-mediated formation of imide–Hg–imide complexes, Dalton Trans., 2011, 40(4), 899–903 RSC.
- D. S. McClure, Triplet–Singlet Transitions in Organic Molecules. Lifetime Measurements of the Triplet State, J. Chem. Phys., 1949, 17(10), 905–913 CrossRef.
- A. K. Singh, S. K. Singh, H. Mishra, R. Prakash and S. B. Rai, Structural, Thermal, and Fluorescence Properties of Eu(DBM)3Phenx Complex Doped in PMMA, J. Phys. Chem. B, 2010, 114(41), 13042–13051 CrossRef PubMed.
|
This journal is © The Royal Society of Chemistry 2018 |
Click here to see how this site uses Cookies. View our privacy policy here.