DOI:
10.1039/C8RA06922K
(Paper)
RSC Adv., 2018,
8, 39463-39469
High-water-absorbing calcium alginate fibrous scaffold fabricated by microfluidic spinning for use in chronic wound dressings†
Received
18th August 2018
, Accepted 7th November 2018
First published on 26th November 2018
Abstract
More and more water-absorbing wound dressings have been studied since moist wound-healing treatment can effectively promote the healing of wounds. In this work, we introduce a novel method to produce improved wound dressings with high-water-absorbance. A high-water-absorbing calcium alginate (Ca-Alg) fibrous scaffold was fabricated simply by microfluidic spinning and centrifugal reprocessing. The structure and physical properties of the scaffold were characterized, and its water-absorbing, cytotoxicity properties and other applicability to wound dressings were comprehensively evaluated. Our results indicate that this material possesses high water-absorbing properties, is biocompatible, and has a 3D structure that mimics the extracellular matrix, while Ca-Alg fibers loaded with silver nanoparticles (AgNPs) exhibit broad-spectrum antibacterial activities; these properties meet the requirements for promoting the healing of chronic wounds and are widely applicable to wound dressings.
1. Introduction
A wound is an injury to normal tissue caused by external physical, chemical, biological, or other types of damage. Based on healing time, wounds are categorized as acute wounds (such as those resulting from surgical incisions and mechanical injuries) and chronic wounds (such as decubitus ulcers and diabetic foot ulcers). The increasing prevalence of chronic wounds, such as decubitus ulcers and ulceration, is closely associated with the elderly and the aging population. These types of chronic wound take long times to heal and are often accompanied by extensive wound exudates that can easily cause wound infection and ulceration if not promptly removed, thereby further complicating the wound-healing process. If an infection spreads, it can lead to life-threatening complications, such as sepsis, among others.1,2 A series of new functional wound dressings has been developed in recent years for accelerating the healing of these types of chronic wound.
In 1962, based on animal experiments, Winter introduced “moist wound-healing theory”, which suggested that a moist environment doubles the rate of wound healing compared to a dry environment, as a moist environment facilitates growth-factor release and cell proliferation that accelerates the migration of basal epidermal cells into the wound.3 A moist environment can effectively promote the healing of chronic wounds, such as decubitus ulcers and ulceration, provided that the wound is clean. On the basis of this theory, modern wound dressings with water absorption and moisture-retention features were fabricated in the forms of films, foams, hydrocolloids, hydrogels, and fibers. Among them, fiber wound dressings are well ventilated and possess greater surface areas and controllable structures, compared to other types of dressing.4–7 Hence, fiber wound dressings have become the focus of new wound-dressing research.
Alginate is a natural, non-toxic, hemostatic, biodegradable, and biocompatible linear polysaccharide extracted from brown algae. It has been widely used in wound dressings with well-recognized clinical therapeutic efficacy. It is an ideal wound filler that is softer, easier to fold, and easier to apply compared to other wound-dressing materials.8–11 In addition, alginate-based wound dressings are highly water absorbent compared to conventional dressings; they are capable of absorbing 15–17 times their own weight in physiological saline solution, equivalent to 5–7 times that of traditional gauze.12–16 Currently, most alginate-based wound dressings are fabricated from Ca-Alg. These wound dressings can absorb an extensive amount of wound exudate and expand upon contact with the wound. Ion-exchange reactions between calcium ions in the dressing and sodium ions in the wound exudate results in the formation of a hydrogel layer on the wound surface, providing a moist environment that promotes wound healing.17,18
Microfluidic techniques have been widely used in recent years for biological detection, drug screening, substance isolation, gene sequencing, and in vitro diagnosis, among others, due to advantages that include low loading volumes, rapid detection, cost effectiveness, and high portability.19–22 Many research groups (such as S. H. Lee, J. Qin, Q. Liang, et al.) have proposed and used microfluidic spinning to fabricate a series of fibers with varying morphologies, which broadens the applications of microfluidic chips.23–34 For instance, Jun et al. (2014) encapsulated cells in fibers by microfluidic spinning to fabricate a bottom-up biomedical porous material for tissue-engineering studies.24–29 In addition, Yu et al. (2014) fabricated an encodable biomimetic bamboo-like hybrid microfiber, while Xie et al. (2018) fabricated necklace-like microfibers using microfluidic spinning and developed other tissue-engineering applications for these fibers by integrating them with various types of cells and growth factors.30–34
Most pure natural polymers suitable for wound dressings can be prepared by microfluidic spinning in an aqueous environment devoid of organic solvents. On the other hand, the basic materials for electrospinning are polymers and solvents, commonly organic solvents, which are disadvantageous because some of them are toxic or highly cytotoxic. Consequently, there is a significant hurdle for the use of electrospun fibers in biomedical-engineering and wound-dressing applications. In contrast to electrospinning, microfluidic spinning, in which the materials are usually cell-friendly, is more suitable for wound-dressing applications. In addition, microfluidic spinning uses an eco-friendly aqueous system, which is more environmental friendly than electrospinning, and the electrospinning of pure natural polymers is generally difficult.23 To the best of our knowledge, to date, alginate, a common polysaccharide, has not been electrospun on its own, due to its high viscosity and intermolecular repulsions between its anionic groups.35 However, Ca-Alg fibers are easily fabricated by microfluidic spinning using pure aqueous sodium alginate solutions; organic solvents are not used throughout the entire microfluidic-spinning process, which is not possible using other spinning methods. For instance, electrospinning uses electrostatic forces and has strict voltage, humidity, and temperature requirements. On the other hand, microfluidic spinning is based on micro-scale fluid dynamics. The factors that influence microfluidic spinning are different to those of the bulk fluid due to surface-tension and energy-dissipation differences, as well as fluidic resistance.23 In addition, the preparation conditions for microfluidic spinning have no special voltage, humidity, or temperature requirements. The microfluidic-spinning process also involves a mild and wet environment, which contributes to the encapsulation of sensitive materials such as cells. Above all, the above-mentioned characteristics provide certainty when preparing safe, non-toxic and bioactive wound dressings.
The aforementioned features of moist wound-healing theory and microfluidic spinning inspired us to study new wound dressings that are applicable to the treatment of chronic wounds with extensive exudates, such as decubitus ulcers and ulcerations. In this study, microfluidic spinning was used to fabricate a calcium alginate (Ca-Alg) fibrous scaffold for wound-dressing use. There are following novelty points in our work. Firstly, there was rare to study the application of fibrous scaffold prepared via microfluidic spinning technology for wound healing; secondly, Ca-Alg fibrous scaffold not only possessed excellent biocompatibility and degradability, but also could fill the wound since its 3D structure, as the degradation speed of Ca-Alg fibrous scaffold was very fast, and its biocompatibility was outstanding, therefore, it can fused together with the new tissue without changing the wound dressings, which can accelerate the wound healing; thirdly, Ca-Alg fibrous scaffold had high porosity (94.4%) that could better to facilitate gas exchange between the wound and the outside environment, the high contact area of material and wound (specific surface area: 14.8 m2 g−1), which were beneficial to accelerate the healing of wound; in addition, the Ca-Alg fibrous scaffold possessed the higher water absorbing (35 g g−1) than the traditional calcium alginate fiber (7–15 g g−1).12–16 Its structural and morphological features were characterized using biological microscopy, scanning electron microscopy (SEM), inductively coupled plasma mass spectrometry (ICP-MS), mercury-intrusion porosimetry (MIP), and X-ray diffraction (XRD).
2. Experimental
2.1. Materials
Sodium alginate (Na-Alg) (viscosity = 1.05–1.15 Pa s) was purchased from Tianjin Fuchen Chemistry Reagent Factory (Tianjin, China). Calcium chloride (anhydrous) (CaCl2) (A.R.) was obtained from Xilong Chemical Co., Ltd. (Guangzhou, China). AgNP solution (0.1 mg mL−1, 20 nm) was purchased from Nanjing XFNANO Materials Tech Co., Ltd. (Nanjing, China). L929 mouse fibroblasts were obtained from the Shanghai Cancer Institute (Shanghai, China). Dulbecco's modified Eagle's medium (DMEM) and Cell Counting Kit-8 (CCK8) reagent were purchased from Paisley (UK). E. coli and S. aureus were obtained from Shanghai Luwei Technology Co., Ltd. (Shanghai, China). Nutrient agar plates were purchased from Shanghai Shenqi Biotechnology Co., Ltd. (Shanghai, China). Customized microfluidic chips used in this work were tailor made by the Dalian Institute of Chemical Physics, Chinese Academy of Sciences (Dalian, China).
2.2. Fabrication of the calcium alginate fibrous scaffold
A CaCl2 coagulation solution (2 wt%) and a Na-Alg spinning solution (2 wt%, 421 mPa s) were loaded into separate 2 L reagent bottles connected to a fluid-injection system (home-made) and a 10 mL syringe was fixed on the LSP02-1B microinjection pump (Longer Precision Pump Co., Ltd.), respectively. Fig. 1(a) shows that both solutions were respectively fed into channels A and B of the microfluidic chip, which was positioned in a Petri dish (diameter = 12 cm). Subsequently, 80 mL of the 2 wt% CaCl2 solution was added to the Petri dish. The solutions were continuously injected at 200 mL h−1 for CaCl2 and 0.5 mL h−1 for Na-Alg. As shown in Fig. 1(b), calcium alginate (Ca-Alg) fiber formed due to rapid Na+/Ca2+ exchange at the intersection within the chip.
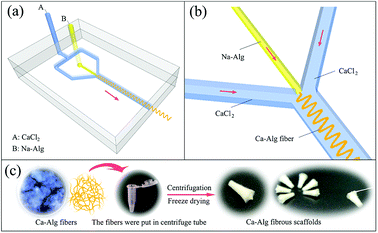 |
| Fig. 1 The schematic diagram was the process of fabrication Ca-Alg fibrous scaffolds using microfluidic spinning technology. (a) The structure diagram of microfluidic chip; (b) enlarged view of crossing section of A and B fluids; (c) the preparation process of Ca-Alg fibrous scaffolds. | |
The process used to fabricate the Ca-Alg fibrous scaffold is shown in Fig. 1(c). Briefly, Ca-Alg fibers were positioned in 1.5 mL centrifuge tubes and placed in a centrifuge (TGL-16B, Shanghai Anting Scientific Instrument Factory), and centrifuged at 10
000 rpm (10k rpm) for 10 min. After centrifugation, the resulting supernatant was discarded and the centrifuge tubes were frozen at −16 °C for 24 h, followed by vacuum lyophilization in a freeze dryer (Lab-1-80, Beijing Boyikang Laboratory Apparatus Co., Ltd.) for 24 h.
Ca-Alg fibers loaded with AgNPs were prepared as described for the Ca-Alg fibers. The 2 wt% Na-Alg and 0.1 mg mL−1 AgNP solutions were evenly mixed at a 2
:
1 mass ratio to prepare a spinning solution of Na-Alg loaded with AgNPs.
2.3. Characterization
2.3.1. Biologic microscope and SEM. One Ca-Alg fiber and its water-absorbed scaffold were separately placed on glass slides and excess water was removed with filter paper. Morphologies were examined by biological microscopy (OLYMPUS-CX31, Olympus Co., Japan).An appropriate amount of the fibers and the fibrous scaffold were fixed onto electron-microscope stages with conductive resins, after which they were coated with gold for 30 s. The morphologies of the gold-coated materials were examined by scanning electron microscopy (SEM, SU8010, Hitachi, Japan) at a 5 kV accelerator voltage.
2.3.2. MIP. The pore structure of the scaffold was characterized by mercury-intrusion porosimetry (MIP, PoreMaster-60, Quantachrome Instruments Co., Ltd., USA) in mercury-intrusion-scanning mode under the following conditions: the sample was dried at 40 °C for 48 h and examined at a mercury-solid contact angle of 140° and a mercury surface tension of 0.48 N m−1.
2.3.3. XRD. XRD was performed using an X-ray diffractometer (Bruker D8, Bruker Corporation, Germany) equipped with a copper target as the diffraction source, and at a voltage of 40 kV, a current of 300 mA, a scanning speed of 5° min−1, and a 10–90° scanning range.
2.3.4. ICP-MS. The silver content of the Ca-Alg fibers loaded with AgNPs were measured by inductively coupled plasma optical emission spectrometry (ICP-OES, Agilent Technologies, Inc., USA) under the following conditions: 1 kW of power, a 15 L min−1 plasma gas flow rate, a 1.5 L min−1 auxiliary gas flow rate, a 0.75 L min−1 nebulizer gas flow rate, and a 15 rpm pump speed.
2.4. Water absorption measurements
The Ca-Alg fibrous scaffold was weighed (recorded as m0) and added to 20 mL of distilled water in a 5 cm Petri dish. After immersion in water for 1 h at room temperature, the scaffold was removed using forceps and weighed (recorded as m1) after being blotted with a filter paper to remove excess water from the surface. The water absorption coefficient (Q) was calculated using the following equation:
The water absorption of the Ca-Alg scaffold was compared with that of a Ca-Alg 2D membrane, which was fabricated and examined in parallel. Briefly, a 2 cm rubber ring was first placed in a Petri dish that was filled with 2 wt% CaCl2 solution, after which 2 wt% sodium alginate solution was poured over the center of the rubber ring until the ring was entirely covered. Rapid ion exchange between the two solutions led to the formation of a Ca-Alg 2D membrane, which was then cooled to −16 °C for 24 h and further subjected to vacuum lyophilization for 24 h in a freeze dryer. The Ca-Alg 2D membrane was subjected to the same water-absorption testing as described for the above-mentioned Ca-Alg fibrous scaffold (n = 3).
2.5. Structural stability testing
Ca-Alg fibers were loaded in 1.5 mL centrifuge tubes and separately centrifuged at 4k, 6k, 8k, and 10k rpm for 10 min. Excess water in the upper part of the centrifuge tube was then removed by pipette. The Ca-Alg fibrous scaffolds were prepared following freeze drying. Each prepared sample was then placed in a 5 cm-diameter Petri dish, 20 mL of distilled water was added and the morphology was examined after incubation for 1 h at room temperature.
2.6. Cell-proliferation assay
Cell-proliferation rates in the presence of 1 mg mL−1 of the Ca-Alg fibrous scaffold was assessed in CCK8 assays using physiological saline solution as the blank control group. An L929 cell suspension in DMEM containing 10% fetal bovine serum was seeded into a 96-well plate at 8 × 103 cells per well and grown to the logarithmic phase at 37 °C and 5% CO2, after which experimental samples were added and incubation continued for a further 24 h. The medium was then discarded and replaced with fresh serum- and antibiotic-free DMEM containing 10% CCK8 reagent, after each well was rinsed twice with physiological saline solution. The plate was further incubated at 37 °C and 5% CO2 for 1 h. Each sample was tested five times. The growth status of the cells was determined using a ZOE™ inverted microscope (Bio-Rad Laboratories, Inc., USA), and the OD450 value for each well was measured using a SYNERGY™ Multi-Mode microplate reader (Biotek Instruments, Inc., USA) in order to calculate the relative growth rate (RGR%), as follows: RGR% = ODt/ODnc × 100%, where ODt and ODnc refer to the absorbance values of the sample and blank groups, respectively.
2.7. Antibacterial properties
Both Ca-Alg fibers and Ca-Alg fibers loaded with AgNP materials were subjected to antibacterial assays using the bacteriostatic ring method. Prior to each experiment, samples loaded on 8 cm discs were sterilized by ultraviolet radiation for 2 h. E. coli and S. aureus (0.3 mL) broth cultures were aliquoted and spread onto the surfaces of nutrient agar plates using a coated rod. The surfaces of the agar plates were allowed to dry for 15 min before sample discs of Ca-Alg fibers and Ca-Alg fibers loaded with AgNPs were placed onto the plates. The agar plates were then incubated in an MJX Intelligent Incubator (Ningbo Jiangnan Instrument Factory) at 37 °C for 16 h. The samples on the agar plates were then examined, and the diameters of the inhibition zones were measured.
2.8. Statistical analyses
The experimental data were analyzed using SPSS13.0 statistical software. A between-group comparison was performed by one-way analysis of variance, and α = 0.05 was selected as the threshold for statistical significance.
3. Results and discussion
3.1. Physico-morphological structures
The physico-morphological structures of Ca-Alg fibers and the Ca-Alg fibrous scaffold were examined by biological microscopy and SEM. The diameter of Ca-Alg fibers would increase as the velocity of the Na-Alg solution increase (Fig. S1 and S2†), on the contrary, the diameter would reduce as the velocity of the CaCl2 solution increase (Fig. S3 and S4†). The diameter of the dry fibers increased 1.4 times after thoroughly swollen (Fig. S5†). As shown in Fig. 2(a), the Ca-Alg fibers exhibit continuous and uniform fibrous morphologies with smooth surfaces following absorption of water. Fig. 2(b) reveals that the Ca-Alg fibrous scaffold has a good uniform porous structure with some fusion points at the fibers junctions, as indicated by arrows, which are ascribable to the intertwisting and merging of soft Ca-Alg fibers under high centrifugal force; as a result, the structure of the scaffold was formed through physical bonding.
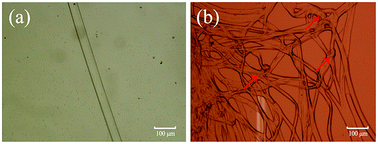 |
| Fig. 2 Biologic microscope images of Ca-Alg fibers and Ca-Alg fibrous scaffold in water absorption state. (a) Ca-Alg fiber ×10; (b) Ca-Alg fibrous scaffold ×10. | |
Fig. 3 displays SEM images of the Ca-Alg fibers and the Ca-Alg fibrous scaffold, which reveals that Ca-Alg fibers have porous structures with uniform diameters, as shown (Fig. 3(a and b)). Following centrifugation, the Ca-Alg fibers were densely intertwined and some fibers were broken (Fig. 3(c)); bonding points at fiber intersections are clearly observable in Fig. 4(d), as indicating by arrows. The above results indicate that at an effective centrifugal force, the bonding forces between the fibers in the scaffold are sufficiently large to ensure the structural stability of the scaffold.
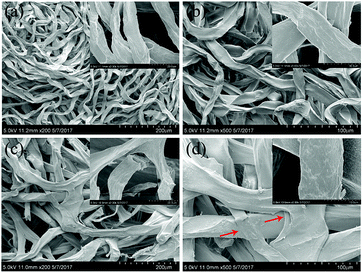 |
| Fig. 3 The SEM images of Ca-Alg fibers and the Ca-Alg fibrous scaffold. (a) Ca-Alg fibers ×200; (b) Ca-Alg fibers ×500; (c) Ca-Alg fibrous scaffold ×200; (d) Ca-Alg fibrous scaffold ×500. | |
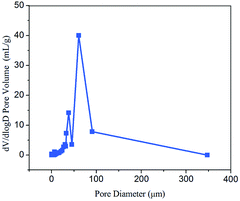 |
| Fig. 4 Pore size distribution of Ca-Alg fibrous scaffold. | |
MIP measurements reveal that the Ca-Alg fibrous scaffold has a porosity of 94.4% and an average pore diameter of 3.9 μm (Fig. 4). The densely interconnected porous framework renders the Ca-Alg fibrous scaffold well ventilated and facilitates the metabolic exchange of nutrients and water between the wound and the external environment. The scaffold can also absorb a large amount of wound exudate in order to create a moist environment, thereby favoring wound healing.36–38 As shown in Fig. S6,† the compression resistance of the Ca-Alg fibrous scaffold was about 5 MPa, indicating excellent mechanical properties as a kind of wound dressings.
3.2. Chemical characterization
In this study, we investigated the chemical properties of Ca-Alg fibers and the Ca-Alg fibers loaded with AgNPs by XRD and ICP-MS. The XRD spectra shown in Fig. 5 reveal a pronounced amorphous peak at 2θ = 21.4° for the Ca-Alg fibers (Fig. 5(a)); however, following the addition of AgNPs, a crystal diffraction peak corresponding to microcrystalline AgNPs (111) was observed at 2θ = 38.1°, in addition to the amorphous peak for Ca-Alg (Fig. 5(b)), which confirms the presence of AgNPs in the Ca-Alg fibers loaded with AgNPs. The ICP-OES spectrum revealed a Ag content of 4030.9 mg kg−1, which also supports the existence of elemental Ag in the Ca-Alg fibers loaded with the AgNP-containing scaffold.
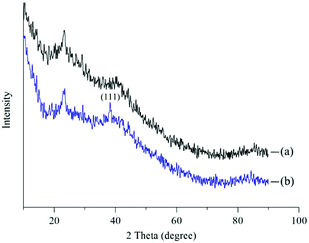 |
| Fig. 5 XRD spectra of (a) Ca-Alg fibers and (b) Ca-Alg fibers loaded with AgNPs. | |
3.3. Water absorbability testing
As shown in Fig. 6(a), the water absorbing capacity of the Ca-Alg fibrous scaffold was compared with that of the two-dimensional (2D) Ca-Alg membrane, which served as the control. Fig. 6(b) reveals that the scaffold retains its original morphology following water absorption. Calculations show that the water absorbing capacity of the Ca-Alg fibrous scaffold (3573%) was 3.9 times that of the Ca-Alg 2D membrane (908%). In addition to the excellent water absorbing ability of the Ca-Alg itself, the Ca-Alg fibrous scaffold contains densely distributed micron-sized pores that effectively improve water absorption by facilitating the retention of larger amounts of water.25,39
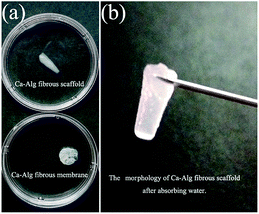 |
| Fig. 6 Water absorption test of Ca-Alg fibrous scaffold (a) the experimental image of water absorption test for Ca-Alg fibrous scaffold and 2D membrane; (b) the morphology of the Ca-Alg fibrous scaffold after water absorption test. | |
In this study, the Ca-Alg fibrous scaffold fabricated by microfluidic spinning was shown to absorb 35.7 times its own weight in water; this property can be used in wound dressings to absorb extensive wound exudate. In addition, this material can form a soft and adhesive hydrogel layer that seals the wound, ensuring a moist environment that is conducive to wound healing. Hence, the Ca-Alg fibrous scaffold is suitable for wound-healing applications.
3.4. Structural stability testing
Ca-Alg fibrous scaffolds were fabricated at different centrifugal velocities (4k, 6k, 8k, and 10k rpm), the results of which are shown in Fig. 7(a). After absorbing water for 1 h, the scaffolds swelled without changing their original shapes (Fig. 7(b)). When picked up by tweezers, the Ca-Alg fibrous scaffolds fabricated at low centrifugal velocities lost their original shapes, while that fabricated at a high centrifugal velocity (10k rpm) maintained its shape (Fig. 7(c)), which is ascribable to a denser intertwinement of Ca-Alg fibers induced by the high centrifugal force, which enhances the structural stability of the Ca-Alg fibrous scaffold. The Ca-Alg fibrous scaffold possesses excellent biodegradable in vitro. The compared experiment results of SEM images were shown in the Fig. S6,† the Ca-Alg fibrous scaffold (Fig. S7(a–d)†) exhibited poor permanence of retention characteristic compared with commercial products (Fig. S7(e–l)†). The degradation speed of our sample was the fastest in all test samples.
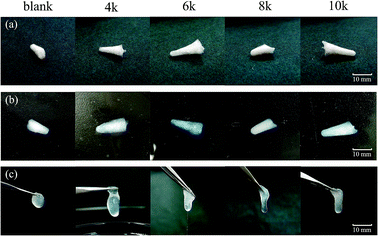 |
| Fig. 7 The structural stability test. (a) The Ca-Alg fibrous scaffolds were prepared at different centrifugal velocity (4k, 6k, 8k and 10k rpm); (b) the experimental images of the materials after absorbing water for 1 h; (c) the experimental images of the materials when picked up by a tweeze. | |
3.5. Cytotoxicity
Good biocompatibility is a prerequisite for materials used for treating wounds. Cytotoxicity assays are important in vitro biocompatibility assays for determining the biological responses of tissue following contact with a medical material. In this study, L929 mouse fibroblasts were studied in cell-proliferation assays to assess the cytotoxicity of the Ca-Alg fibrous scaffold. Fig. 8 reveals that, compared to the control group, adherent L929 cells displayed excellent proliferation and morphologies, with spherical as well as long spindle shapes in the presence of both Ca-Alg fibers and Ca-Alg fibers loaded with AgNPs. The cell-proliferation rates after 24 h of cultivation were 99.7 ± 3.6% (Ca-Alg fibers) and 100.6 ± 3.3% (Ca-Alg fibers loaded with AgNPs), with no significant differences compared to the control group (p > 0.05), indicating that both Ca-Alg fibers and Ca-Alg fibers loaded with AgNPs are non-toxic, safe, and reliable materials that do not significantly affect L929 cell proliferation. Our results provide an experimental basis for further studies into the applications of this material in wound-healing treatments.
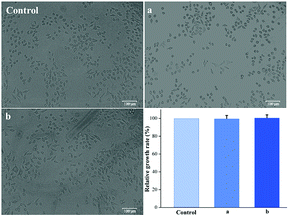 |
| Fig. 8 The images of cell morphologies and cell-proliferation data (control: control group; (a) Ca-Alg fibers; (b) Ca-Alg fibers loaded with AgNPs). | |
3.6. Antibacterial properties
One prerequisite for chronic wound dressings is that they show antibacterial properties that prevent infection of the wound.40 In this study, we assessed the antibacterial properties of Ca-Alg fibers loaded with AgNPs and the control group (Ca-Alg fibers) against E. coli and S. aureus using the bacteriostatic ring method, the results of which are shown in Fig. 9. Compared to the control group, the Ca-Alg fibers loaded with AgNPs exhibited significant zones of inhibition, with diameters of 4.9 mm and 5.2 mm, which indicates significant antibacterial activities against E. coli and S. aureus, respectively. On the other hand, the Ca-Alg fibers showed no bacteriostasis rings. The silver content increased with time point (Fig. S8†). That indicated that the antibacterial property is functional through the release of silver ions. These results indicate that the Ca-Alg fibers loaded with AgNPs and fabricated by microfluidic spinning release silver ions that are significantly antibacterial against both Gram-negative and Gram-positive bacteria. Hence, this material is useful as an antibacterial dressing for wound-healing applications.
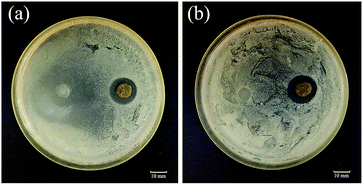 |
| Fig. 9 The results of antibacterial assays using the bacteriostatic ring method for Ca-Alg fibers (left) and Ca-Alg fibers loaded with AgNPs (right). (a) E. coli; (b) S. aureus. | |
4. Conclusions
The Ca-Alg fibrous scaffold fabricated by microfluidic spinning is 94.4% porous and has an interconnected porous framework with a specific surface area of 14.8 m2 g−1. These pores facilitate the retention of large amounts of water and render the scaffold capable of absorbing 35.7 times its own weight in water, which creates a moist environment for wound healing. The cytotoxicity assay indicated that the biocompatibilities of Ca-Alg fibers and Ca-Alg fibers loaded with AgNPs are excellent, while the antibacterial assays indicate that the Ca-Alg fibers loaded with AgNPs exhibit significant antibacterial activities against E. coli and S. aureus. In summary, the Ca-Alg fibrous scaffold fabricated by microfluidic spinning can be used as a new type of wound dressing for the care of chronic wounds.
Conflicts of interest
There are no conflicts of interest to declare.
Acknowledgements
This work was supported by the National Natural Science Foundation of China (No. 81472842, 81502560), the Foundation of Higher Education of Guangdong, China (No. 2016KTSCX146) and Natural Science Foundation of Guangdong Province, China (No. 2018A0303130245).
Notes and references
- M. P. Rowan, L. C. Cancio, E. A. Elster, D. M. Burmeister, L. F. Rose and S. Natesan, et al., Crit. Care, 2015, 19(1), 243–254 CrossRef PubMed.
- K. A. Rieger, J. Mater. Chem. B, 2013, 1(36), 4531–4541 RSC.
- G. D. Winter, Nature, 1962, 193(4812), 293–294 CrossRef CAS PubMed.
- C. Lagarde, P. J. M. Mulkens, R. Wimheuer and T. Bieber, J. Eur. Acad. Dermatol. Venereol., 1998, 11, S141 CrossRef.
- C. T. Chiu, J. S. Lee, C. S. Chu, Y. P. Chang and Y. J. Wang, Mater. Med., 2008, 19(6), 2503–2513 CrossRef CAS PubMed.
- Y. Suzuki, Y. Nishimura, M. Tanihara, K. Suzuki, A. K. Kitahara and Y. Yamawaki, et al., J. Artif. Organs, 1998, 1(1), 28–32 CrossRef CAS.
- F. A. Paskiabi, S. Bonakdar, M. A. Shokrgozar, M. Imani, Z. Jahanshiri and M. Shams-Ghahfarokhi, et al., Mater. Sci. Eng., C, 2017, 73, 130–136 CrossRef CAS PubMed.
- S. E. Barnett and S. J. Varley, Ann. R. Coll. Surg. Engl., 1987, 69, 153–155 CAS.
- D. Hoefer, J. K. Schnepf, T. R. Hammer, M. Fischer and C. Marquardt, J. Mater. Sci.: Mater. Med., 2015, 26(4), 1–9 CrossRef CAS PubMed.
- R. Singh and D. Singh, Mater. Med., 2012, 23(11), 2649–2658 CrossRef CAS PubMed.
- I. R. Sweeney, M. Miraftab and G. Collyer, Carbohydr. Polym., 2014, 102(1), 920–927 CrossRef CAS PubMed.
- Y. Qin, Polym. Int., 2008, 57(2), 171–180 CrossRef CAS.
- Y. Qin, Int. Wound J., 2010, 2(2), 172–176 CrossRef PubMed.
- Y. Qin, J. Appl. Polym. Sci., 2010, 100(3), 2516–2520 CrossRef.
- M. Rezvanian, M. C. Amin and S. F. Ng, Carbohydr. Polym., 2016, 137(2), 295–304 CrossRef CAS PubMed.
- S. Seetharaman, S. Natesan, R. S. Stowers, C. Mullens, D. G. Baer and L. J. Suggs, et al., Acta Biomater., 2011, 7(7), 2787–2797 CrossRef CAS PubMed.
- R. Raguvaran, B. K. Manuja, M. Chopra, R. Thakur, T. Anand and A. Kalia, et al., Int. J. Biol. Macromol., 2017, 96, 185–191 CrossRef CAS PubMed.
- M. C. Straccia, I. Romano, A. Oliva, G. Santagata and P. Laurienzo, Carbohydr. Polym., 2014, 108(1), 321–330 CrossRef CAS PubMed.
- E. Kang, S. J. Shin, K. H. Lee and S. H. Lee, Lab Chip, 2010, 10(14), 1856 RSC.
- E. Kang, G. S. Jeong, Y. Y. Choi, K. H. Lee, A. Khademhosseini and S. H. Lee, Nat. Mater., 2011, 10(11), 877 CrossRef CAS PubMed.
- J. Su, Y. Zheng and H. Wu, Lab Chip, 2009, 9(7), 996–1001 RSC.
- I. Yoo, S. Song, B. Yoon and J. M. Kim, Macromol. Rapid Commun., 2012, 33(15), 1256–1261 CrossRef CAS PubMed.
- J. Cheng, Y. Jun, J. Qin and S. H. Lee, Biomaterials, 2017, 114, 121–143 CrossRef CAS PubMed.
- Y. Jun, E. Kang, S. Chae and S. H. Lee, Lab Chip, 2014, 14(13), 2145–2160 RSC.
- C. H. Mun, J. Y. Hwang and S. H. Lee, Tissue Eng. Regener. Med., 2016, 13(2), 140–148 CrossRef CAS.
- B. G. Chung, K. H. Lee, A. Khademhosseini and S. H. Lee, Lab Chip, 2012, 12(1), 45–59 RSC.
- X. H. He, W. Wang, K. Deng, R. Xie, X. J. Ju and Z. Liu, et al., RSC Adv., 2014, 5(2), 928–936 RSC.
- E. Kang, Y. Y. Choi, S. K. Chae, J. H. Moon, J. Y. Chang and S. H. Lee, Adv. Mater., 2012, 24(31), 4271–4277 CrossRef CAS PubMed.
- D. Y. Park, C. H. Mun, E. Kang, D. Y. No, J. Ju and S. H. Lee, Biofabrication, 2014, 6(2), 024108–024115 CrossRef CAS PubMed.
- Y. Kitagawa, Y. Naganuma, Y. Yajima, M. Yamada and M. Seki, Biofabrication, 2014, 6(3), 035011–035020 CrossRef PubMed.
- Y. Tian, P. Zhu, X. Tang, C. Zhou, J. Wang and T. Kong, et al., Nat. Commun., 2017, 8(1), 1080 CrossRef PubMed.
- P. Xu, R. Xie, Y. Liu, G. Luo, M. Ding and Q. Liang, Adv. Mater., 2017, 29(34), 1701664 CrossRef PubMed.
- C. H. Yeh, P. W. Lin and Y. C. Lin, Microfluid. Nanofluid., 2010, 8(1), 115–121 CrossRef CAS.
- Y. Yu, W. Wei, Y. Wang, C. Xu, Y. Guo and J. Qin, Adv. Mater., 2016, 28(31), 6649–6655 CrossRef CAS PubMed.
- S. Wei and Y. L. Hsieh, Carbohydr. Polym., 2014, 102(1), 893–900 Search PubMed.
- O. Catanzano, V. D'Esposito, S. Acierno, M. R. Ambrosio, C. C. De and C. Avagliano, et al., Carbohydr. Polym., 2015, 131, 407–414 CrossRef CAS PubMed.
- D. Park, J. Park, H. Jang, J. Cheng, S. H. Kim and S. H. Lee, Biofabrication, 2017, 9(2) CrossRef CAS PubMed.
- S. Partap, I. Rehman, J. Jones and J. Darr, Adv. Mater., 2006, 18(4), 501–504 CrossRef CAS.
- S. Kirdponpattara, A. Khamkeaw, N. Sanchavanakit, P. Pavasant and M. Phisalaphong, Carbohydr. Polym., 2015, 132, 146–155 CrossRef CAS PubMed.
- Y. Hu and H. Yang, J. Microb. Biochem. Technol., 2015, 07(4), 228–233 Search PubMed.
Footnote |
† Electronic supplementary information (ESI) available. See DOI: 10.1039/c8ra06922k |
|
This journal is © The Royal Society of Chemistry 2018 |
Click here to see how this site uses Cookies. View our privacy policy here.