DOI:
10.1039/C8BM01258J
(Paper)
Biomater. Sci., 2019,
7, 187-195
Red blood cell-derived nanovesicles for safe and efficient macrophage-targeted drug delivery in vivo†
Received
8th October 2018
, Accepted 25th October 2018
First published on 25th October 2018
Abstract
Macrophage-targeted drug delivery has great therapeutic potential for the treatment of cancers and inflammatory diseases. There is also an unmet need for efficient and nontoxic means of in vivo macrophage depletion to determine the role of macrophages under normal and disease settings. Herein, we explored the potential of red blood cell (RBC)-derived nanovesicles (RDNVs) as drug nanocarriers to specifically deplete macrophages. We show that RDNVs are effective hydrophilic drug carriers and can effectively deliver drugs into macrophages both in vitro and in vivo. Nanovesicles derived from both wild-type mouse RBCs (WT-RDNVs) and CD47 KO mouse RBCs (KO-RDNVs) can encapsulate clodronate with good stability in PBS for long-term storage. However, KO-RDNVs were more efficiently engulfed by macrophages in vitro and more rapidly cleared in vivo than WT-RDNVs, indicating that CD47 also serves as a “don't eat me” molecule for RDNVs as it does for RBCs. Accordingly, clodronate-encapsulated KO-RDNVs (KO-RDNV/CLD) were significantly more toxic to mouse macrophages in vitro than drug-loaded WT-RDNVs (WT-RDNV/CLD). Furthermore, WT-RDNV/CLD showed prolonged accumulation in tissues (e.g., liver and lung) and macrophage depletion versus KO-RDNV/CLD. Importantly, RBC-derived nanovesicles are more biocompatible and less toxic in vivo than clodronate-encapsulated liposomes—the current gold-standard macrophage-depleting reagent. This study offers a useful strategy for macrophage-targeted drug delivery.
Introduction
Immune cells have important functions in autoimmune diseases, inflammatory diseases, and cancer.1 Of these, the pathogenicity of macrophages derives from their ability to modulate the function of neighboring cells—a characteristic that can be exploited when considering their potential as therapeutic targets.2 Previous studies have shown that macrophages are effective therapeutic targets for the treatment of immune disorder.3–8 Rüttinger and co-workers reported that inhibiting macrophage infiltration by a macrophage colony-stimulating factor 1-targeting antibody caused a significant delay in tumor growth.9 Tacke and co-workers found that the pharmacological inhibition of Ly-6C(+) monocyte recruitment using the CCL2-inhibitor accelerates regression from toxic and metabolic liver fibrosis in two independent experimental models.10 However, an efficient and less toxic macrophage targeted drug delivery system is essential for translating this approach into clinical therapies.
Macrophages are phagocytic and can endocytose nanometer- to micrometer-scale particles. This facilitates drug delivery into these cells,11 and novel drug delivery systems are needed to improve the pharmacological and therapeutic properties of i.v.-administered drugs.12,13 Nanoparticle carriers have been prepared and tested using a wide variety of materials including proteins, polysaccharides, synthetic polymers, metals, and many other organic/inorganic materials.14–16 Of these, red blood cells (RBCs) are a potentially attractive and unique carrier for drug delivery.17
Zhang and co-workers have recently developed a new drug delivery platform that pairs nanoscale RBC membrane-derived vesicles with polymeric nanoparticles made from poly(lactic-co-glycolic acid) (PLGA). This can enable the delivery of slow-releasing drug payloads in vivo with a carrier circulation half-life beyond that of PEG.18 The CD47 on the RBC surface actively signals macrophages and prevents nanoparticle uptake.19 There is an assumption that CD47 may contribute to the long circulation of RBC membrane-coated nanoparticles,20 but there is no direct evidence to support it. Interestingly, mouse studies showed that RBC membrane-coated nanoparticles still ultimately accumulated in the liver (60–80%)18—this tissue is enriched with macrophages and is the main place where damaged or aged RBCs are cleared.21 Therefore, RBC-derived nanoparticles may provide an efficient drug delivery system to target macrophages.
Here, we investigated the potential of wild-type (WT) and CD47KO RBC membrane-formed nanoparticles to achieve macrophage-targeted drug delivery in vivo. Nanovesicles derived from both WT and CD47 KO mouse RBCs (denoted as WT-RDNVs and KO-RDNVs, respectively) could encapsulate hydrophilic drugs (e.g., clodronate, a hydrophilic bisphosphonate) with good stability in PBS for long-term storage. CD47 on the cell membrane plays an important role in prolonging the blood circulation of cell membrane-related nanoparticles.20 The KO-RDNVs were cleared more rapidly and completely in vivo. Furthermore, clodronate-loaded WT-RDNVs (WT-RDNV/CLD) achieved more durable macrophage depletion in vivo than clodronate-loaded KO-RDNVs (KO-RDNV/CLD). More importantly, RBC-derived nanovesicles were more biocompatible and less toxic in vivo than clodronate-encapsulated liposomes—the current gold-standard macrophage-depleting reagent.
Experimental section
Chemicals and materials
Clodronate and rhodamine B (RhoB) were purchased from Sigma-Aldrich (St Louis, MO, USA). Bio-Gel P-100 was purchased from Bio-Rad (Hercules, CA, USA). DAPI-fluoromount-G was purchased from Southern Biotech (Birmingham, AL, USA). RPMI 1640 medium, phosphate buffer saline (PBS), penicillin/streptomycin, and L-glutamine were all obtained from Invitrogen (Carlsbad, CA, USA). Annexin V, APC conjugate (APC Annexin V), Alexa Fluor™ 488 Phalloidin, cell trace™ CFSE cell proliferation kit (CFSE) and fetal bovine serum (FBS) were purchased from Thermo Fisher Scientific (Rockford, IL, USA).
Cell line and cell culture
The mouse macrophage cell line RAW264.7 was purchased from American Type Culture Collection (ATCC). Peritoneal macrophages were isolated from C57BL/6 mice 4 days after injection of 2% Bio-Gel P100 gel (1 mL per mouse). RAW264.7 cells and peritoneal macrophages isolated from C57BL/6 mice with intraperitoneal (i.p.) injection of Bio-Gel P100 were maintained in RPMI 1640 supplemented with 10% FBS, 100 U mL−1 penicillin, and 100 μg mL−1 streptomycin at 37 °C under a humidified atmosphere containing 5% CO2.
Animals
4–6 Weeks old female C57BL/6 and NOD.CB17-Prkdcscid (NOD/SCID) mice were obtained from Charles River (Beijing, China). Female C57BL/6 CD47−/− (CD47 KO) mice were obtained from Jackson Laboratory (Bar Harbor, ME, USA). All mice were raised in a specific pathogen-free environment with free access to food and water and received care in accordance with the guidelines outlined in the Guide for the Care and Use of Laboratory Animals. All procedures were approved by the Jilin University Animal Care and Use Committee.
Preparation and characterization of RDNVs
The erythrocyte-derived nanovesicles (RDNVs) were prepared following previously published protocols with modifications.9 Whole blood was collected from healthy C57BL/6 and CD47 KO mice and centrifuged at 800g for 5 min at 4 °C following which the plasma and buffy coat were carefully removed. The resulting packed red blood cells were washed once with cold 1× PBS and suspended in 0.25× PBS on ice for 2 h. The solution was centrifuged twice at 9000 rpm for 10 min to remove the released hemoglobin, and the light pink pellet was resuspended in 1× PBS. The collected RBC ghosts were sonicated in a glass vial with a lid for 5 min using a bath sonicator. To fuse the erythrocyte-derived nanovesicles with drugs, clodronate was added into the RBC-membrane-derived vesicle solution at a concentration of 200 mg mL−1. The mixture was extruded through a 400 nm polycarbonate porous membrane using an Avanti mini extruder (Avanti Polar Lipids, Inc., Alabaster, AL, USA) 10 times. The unencapsulated drug molecules were removed by repeated concentration in ultrafiltration tubes (MWCO 3 kDa, Millipore).
The hydrodynamic diameter and zeta potential of RDNVs were measured by dynamic light scattering (DLS, Zetasizer Nano ZS90, Malvern, Southborough, UK) at 25 °C. The NP morphologies were observed with a transmission electron microscope (TEM) operating at 120 kV (HT7700, Hitachi, Tokyo, Japan). DLS was also used to monitor the stability of drug-loaded RDNVs in PBS. The drug loading capacities of WT-RDNVs and KO-RDNVs were observed by direct stochastic optical reconstruction microscopy (dSTORM). The RhoB encapsulated WT-RDNVs or KO-RDNVs were mounted on pre-cleaned glass slides. STORM imaging was performed on a Nikon Ti-E microscope with a 100 × 1.49 NA TIRF lens (Nikon, Japan). Fluorescence signals were collected via a cooled EMCCD camera (Photometrics, Cascade II).
Cell uptake assay
Mouse peritoneal macrophages (PMs) collected from C57BL/6 mice 4 days after i.p. injection of 1 mL 2% Bio-Gel P100 were used as effector cells.13 Then, 100 μL RhoB-loaded WT-RDNVs or KO-RDNVs were added into the culture medium of 2 × 106 mouse PMs. Cell uptake was assessed by confocal microscopy (Carl Zeiss LSM880, Oberkochen, Germany) and flow cytometry (BD FACS Canto II, New Jersey, USA) after 2 h or 24 h of incubation.
Cell viability assay
The in vitro cytotoxicity of clodronate-loaded WT-RDNVs or KO-RDNVs on RAW264.7 cells was measured via an MTS assay. Briefly, RAW264.7 cells were seeded in 96-well plates at a density of 5000 cells per well and cultured overnight. WT-RDNV/CLD and KO-RDNV/CLD were diluted using an RPMI 1640 culture medium with a final clodronate concentration of 1, 5, 25, 50, 100 or 500 ng mL−1. The culture medium was replaced with fresh RPMI 1640 containing WT-RDNV/CLD, KO-RDNV/CLD, or liposomal clodronate (Clodrosome) at different concentrations. A drug-free culture medium served as the control. After 24 h of incubation, the cells were incubated with MTS at 37 °C for 2 h, and formazan crystals were produced in metabolically active cells with analysis performed at 490 nm using a multi-mode microplate reader (Synergy H1, BioTek, USA).
Apoptosis assay
RAW264.7 cells were seeded in 6-well plates at a density of 2 × 105 cells per well and allowed to grow to 70–80% confluence. The cells were then incubated with a culture medium containing Clodrosome, WT-RDNV/CLD, or KO-RDNV/CLD at a clodronate concentration of 200 ng mL−1 at 37 °C for 2 h. The PI/Annexin V-APC assay was used to measure the apoptotic cells using flow cytometry according to the manufacturer's instruction. Briefly, the cells were harvested by centrifugation at 500g for 5 min and washed three times with ice-cold PBS. The suspended cells were incubated in 300 μL binding buffer containing 5 μL APC-Annexin V and 5 μL PI for 30 min at room temperature in the dark. The apoptotic cells were measured by using BD FACS Canto II, and the data were analyzed with the FlowJo software.
In vivo pharmacokinetics studies
To evaluate the circulation half-life of erythrocyte derived nanovesicles in vivo, 200 μL RhoB-loaded WT-RDNVs or KO-RDNVs were administered to C57BL/6 mice by i.v. injection. Peripheral blood was collected at 0.5, 1, 2, 4, 6, 8, 24, and 48 h after injection. Each group contained three mice. The collected blood samples were diluted with 90 μL PBS in a 96-well plate before fluorescence measurements.
Measurement of tissue distribution
To study the biodistribution of the nanoparticles in mice, C57BL/6 mice received an i.v. injection of 500 μL RhoB loaded WT-RDNVs or KO-RDNVs at RhoB concentrations of 1 mg mL−1. The in vivo distribution of RhoB was measured at 1, 2, 6, 10, 12, and 24 h post-injection with a Xenogen IVIS Lumina system (PerkinElmer, WA, USA). The results were analyzed using the Living Image® 3.1 software. Mice were sacrificed 24 h post-injection, and the livers, spleens, lungs, lymph nodes, and arteries were harvested and imaged for RhoB-loaded RDNV distribution using a Xenogen IVIS Lumina system.
Histopathological analysis
Hearts, lungs, spleens, livers, and kidneys were harvested from C57BL/6 mice at 6 and 24 h after the administration of Clodrosome, WT-RDNV/CLD, KO-RDNV/CLD, or PBS. The organs were fixed in 4% paraformaldehyde or cryopreserved in an optimal cutting temperature (OCT) compound for immunofluorescence. Paraffin sections were prepared for hematoxylin and eosin (H&E) staining. The slides were observed and photographed with an optical microscope (IX71, Olympus, Tokyo, Japan). For immunofluorescence, frozen tissue sections were prepared and stained with the Alexa Fluor 488 anti-mouse CD68 antibody. Images were captured with a Zeiss LSM 880 confocal laser scanning microscope using a 20× objective (Carl Zeiss, Oberkochen, Germany).
Macrophage depletion in mice
Macrophage depletion in vivo used Clodrosome, WT-RDNV/CLD, KO-RDNV/CLD, and the PBS control with equal amounts of clodronate. The doses of injected clodronate were 25 and 12.5 μg per g body weight per day for day 0 and day 1, respectively. Mice in each group were injected with a mixture of 5 × 107 CFSE-labeled CD47−/− mouse RBCs and 5 × 107 CFSE-labeled CD47+/+ mouse RBCs via the tail vein. Five microliters of blood were collected at various times, and the percentages of CFSE+ RBCs were determined by flow cytometry.
In vivo toxicity test
The toxicity tests of WT-RDNV/CLD and KO-RDNV/CLD were performed on female NOD/SCID mice. Liposomal clodronate was used as a positive control. Mice were administered Clodrosome, WT-RDNV/CLD, or KO-RDNV/CLD with equal amounts of clodronate every day for 21 days: the doses of injected clodronate were 25 μg per g body weight on day 0 and 12.5 μg per g body weight per day for the remaining 20 days. The body weight changes of mice were observed every other day.
Statistical analysis
The data are presented as the mean ± standard error of mean. The level of significant differences between group means was determined by the Student's t test. All statistical analysis was performed using the GraphPad Prism software (GraphPad Software Inc.). A p value of less than 0.05 was considered statistically significant.
Results and discussion
Preparation and characterization of drug-loaded erythrocyte derived nanovesicles
To evaluate whether RBC membrane-derived nanovesicles can be used as drug delivery vehicles, we synthesized clodronate-loaded RDNVs using red blood cells harvested from wild type and CD47-deficient C57BL/6 mice (denoted as WT-RDNV/CLD and KO-RDNV/CLD, respectively) following a procedure of hypotonic dialysis and isotonic resealing as described with slight modifications.18 Transmission electron microscopy (TEM) analysis revealed that both WT-RDNV/CLD and KO-RDNV/CLD exhibited a hollow spherical morphology with similar diameters (Fig. 1a). The hydrodynamic diameters were 258.3 ± 9.5 nm for WT-RDNV/CLD and 265.3 ± 9.7 nm for KO-RDNV/CLD (Fig. 1b). The zeta potentials for WT-RDNV/CLD and KO-RDNV/CLD were −16.7 ± 1.5 mV and −16.0 ± 3.0 mV (Fig. 1c), respectively, which indicates that the surface CD47 has negligible effects on the physical properties of the RDNV.
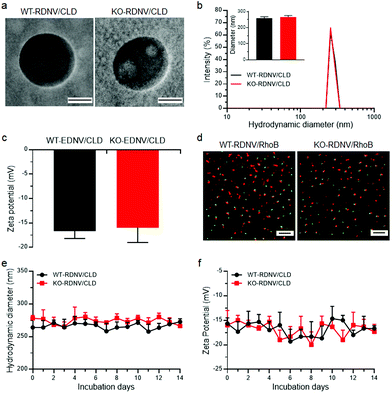 |
| Fig. 1 Characterization of drug-loaded WT-RDNVs and KO-RDNVs. (a) TEM images of WT-RDNV/CLD and KO-RDNV/CLD. Scale bar = 100 nm. (b, c) Mean values of the (b) particle size and (c) zeta potential of WT-RDNV/CLD and KO-RDNV/CLD measured by dynamic light scattering (mean ± SD; n = 3 per group). (d) dSTORM images showing the drug-loading efficiency of rhodamine B by WT-RDNVs and KO-RDNVs. WT-RDNVs or KO-RDNVs were labeled with DiD (Ex = 644 nm/Em = 665 nm) and marked as red, and hydrophilic rhodamine B (Ex = 553 nm/Em = 627 nm) was marked as green. Scale bar = 2 μm. (e, f) Changes of the (e) particle sizes and (f) zeta potentials of WT-RDNV/CLD and KO-RDNV/CLD in PBS solution over two weeks. | |
The drug loading capacities of WT-RDNVs and KO-RDNVs were evaluated by dSTORM. The two-dimensional dSTORM images demonstrated that almost all nanovesicles encapsulated rhodamine B (Fig. 1d)—a hydrophilic dye characteristic of water-soluble drugs. In addition, the long-term stability under physiological conditions is one of the major challenges in the development of nanoparticles for drug delivery. Thus, the particle sizes and zeta potentials of WT-RDNV/CLD and KO-RDNV/CLD incubated in PBS at 4 °C were measured at different time points for 14 days. Fig. 1e and f show that both the diameters and zeta potentials of the WT-RDNV/CLD and KO-RDNV/CLD fluctuated over a relatively small range of values indicating that the clodronate-loaded nanovesicles can be stored stably for a long time. These data suggested that erythrocyte-derived nanovesicles are suitable for hydrophilic therapeutic drug delivery, and CD47 does not significantly affect the physical properties of these nanovesicles.
CD47 reduced the interaction between erythrocyte derived nanovesicles and macrophages in vitro
Cell surface CD47 inhibits phagocytosis by macrophages.22 However, there is no direct experimental evidence proving that surface CD47 prevents phagocytosis of erythrocyte-derived nanovesicles by macrophages. To address this question, we first determined the contribution of surface CD47 to the cellular uptake of drug-loaded RDNVs by macrophages in vitro. The distributions of rhodamine B encapsulated WT-RDNVs and KO-RDNVs in RAW264.7 cells were measured by confocal laser scanning microscopy and flow cytometry at different time points. Fig. 2a shows that RAW264.7 cells treated with either WT-RDNV/RhoB or KO-RDNV/RhoB showed detectable rhodamine B fluorescence after 2 hours of incubation with almost all signals distributed in the cytoplasm. There was no significant difference between the amount of WT-RDNV/RhoB and KO-RDNV/RhoB phagocytized by RAW264.7 (Fig. 2b). However, the rhodamine B fluorescence signal distributed in the cytoplasm of RAW264.7 was significantly enhanced after 24 hours of incubation (Fig. 2c). This was detected under the same experimental conditions as in Fig. 2a. The MFI of rhodamine B of KO-RDNV/RhoB treated cells was significantly higher than that of WT-RDNV/RhoB treated cells (Fig. 2d). These data indicate that surface CD47 on erythrocyte-derived nanovesicles only slightly inhibits the phagocytosis by macrophages, which is different from the function of CD47 on RBCs. The differences in physical properties between RDNVs and RBCs (such as size, shape and surface protein) explain why the activity of CD47 is weakened.
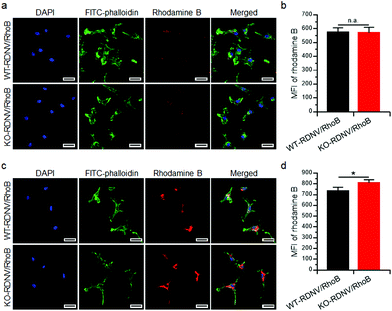 |
| Fig. 2 Cellular uptake of drug-loaded WT-RDNVs and KO-RDNVs by macrophages. (a) CLSM images shows the distribution of RDNVs in RAW264.7 cells after culture with WT-RDNV/RhoB or KO-RDNV/RhoB at 37 °C for 2 h. The cell nuclei were stained with DAPI (blue), the cytoskeleton was stained with FITC-phalloidin (green), and the RDNVs were labeled with rhodamine B (red). (b) The intracellular rhodamine B in RAW264.7 cells cultured with WT-RDNV/RhoB or KO-RDNV/RhoB for 2 h was measured by flow cytometry. (c) CLSM images show the distribution of RDNVs in RAW264.7 cells after culture with WT-RDNV/RhoB or KO-RDNV/RhoB at 37 °C for 24 h. The cell nuclei were stained with DAPI (blue), the cytoskeleton was stained with FITC-phalloidin (green), and the RDNVs were labeled with rhodamine B (red). (d) The intracellular rhodamine B in RAW264.7 cells cultured with WT-RDNV/RhoB or KO-RDNV/RhoB for 24 h was measured by flow cytometry. The data are shown as the mean ± SD, *: p < 0.05. | |
We further determined whether a lack of CD47 could improve the toxicity of clodronate-encapsulated RDNVs to macrophages via increased drug delivery efficiency. RAW264.7 cells were cultured with WT-RDNV/CLD, KO-RDNV/CLD, Clodrosome, or the PBS control at clodronate concentrations of 200 ng mL−1 for 24 h. The apoptosis was measured by flow cytometry. Fig. 3a shows that more apoptotic (Annexin-V+) RAW264.7 cells were observed in the KO-RDNV/CLD-treated group than in the WT-RDNV/CLD, which was similar to that of Clodrosome—a commercial macrophage-depletion reagent. We further evaluated the effect of clodronate-loaded WT-RDNVs and KO-RDNVs at different concentrations on the cell viability of macrophages using the MTS assay. A strong and dose-dependent inhibition of RAW264.7 was observed in both the WT-RDNV/CLD and KO-RDNV/CLD groups (Fig. 3b The WT-RDNV/CLD had significantly less cytotoxicity to macrophages than Clodrosome at a high concentration of clodronate. KO-RDNV/CLD had a similar effect to that of Clodrosome. These data indicate that CD47 played a key role in the phagocytosis of erythrocyte-derived nanovesicles by macrophages.
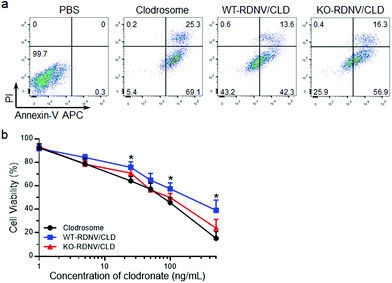 |
| Fig. 3 Clodronate-loaded WT-RDNVs and KO-RDNVs have significant cytotoxicity to macrophages in vitro. (a) RAW264.7 cells were cultured with WT-RDNV/CLD, KO-RDNV/CLD, or Clodrosome for 24 h, and analyzed for apoptosis by Annexin-V/PI staining using flow cytometry. The concentration of clodronate was 200 ng mL−1. (b) The cell viability of RAW264.7 cells cultured with WT-RDNV/CLD, KO-RDNV/CLD or Clodrosome for 24 h was measured by the MTS assay (n = 3). The concentration of clodronate was 1 to 500 ng mL−1. The data are presented as mean ± SD, *: p < 0.05. | |
CD47 prolonged the blood circulation and enhanced the targeted macrophage drug delivery of erythrocyte-derived nanovesicles in vivo
Previous studies have shown that CD47 is an essential marker of “self”, and its signaling through signal regulatory protein-α (SIRPα) prevents inappropriate self-phagocytosis. Hematopoietic cells not expressing CD47 are rapidly cleared from the blood stream by macrophages and DCs.19,22 Moreover, CD47 has also been implicated in apoptotic cell phagocytosis by macrophages for mis-localized CD47, which cannot send “self-signal” via SIRPα.23 Thus, the status of surface CD47 may significantly influence the biodistribution and pharmacokinetics of erythrocyte-derived nanovesicles. Nanoparticles typically have a significantly longer plasma circulation half-life than their micrometer-sized counterparts in vivo, and thus we compared the biodistribution and pharmacokinetics between WT-RDNVs and KO-RDNVs. First, we assessed the effects of CD47 on the biodistribution of RDNV using fluorescent dye rhodamine B-loaded WT-RDNVs and KO-RDNVs. The rhodamine B fluorescence in mice administered WT-RDNV/RhoB was stronger than that in KO-RDNV/EhoB at each time point within 24 hours after i.v. injection (Fig. 4a). At 24 h post-injection, the WT-RDNV/RhoB-treated mice had significantly increased accumulation of rhodamine B in the lungs, liver, and inguinal lymph nodes compared to KO-RDNV/RhoB—these are macrophage-rich organs8,24 (Fig. 4b). However, there was no detectable rhodamine B signal in the spleens treated with these two RDNVs. However, a stronger rhodamine B fluorescence signal was found in the aorta of mice administered KO-RDNV/RhoB (Fig. S1†) indicating that CD47 should be one of the key factors which affected the accumulation of EDNVs in blood vessels. Furthermore, we compared the in vivo pharmacokinetic behavior of these two RDNVs to investigate the function of CD47 on the blood circulation of RDNVs. Rhodamine B-loaded WT-RDNVs or KO-RDNVs were injected into wild type C57BL/6 mice at a rhodamine B dose of 10 mg kg−1. At various time points following the injection, 20 μL blood was collected from the tail vein of the mice for fluorescence measurements. Fig. 4c shows that the WT-RDNVs had better blood retention than the KO-RDNVs. The fluorescence signal of WT-RDNV/RhoB was undetectable 48 h after injection while KO-RDNV/RhoB was not detected after 8 hours indicating that WT-RDNV/RhoB had significantly slower elimination from the blood circulation. These data demonstrated that CD47 significantly affects the in vivo fate of RDNVs.
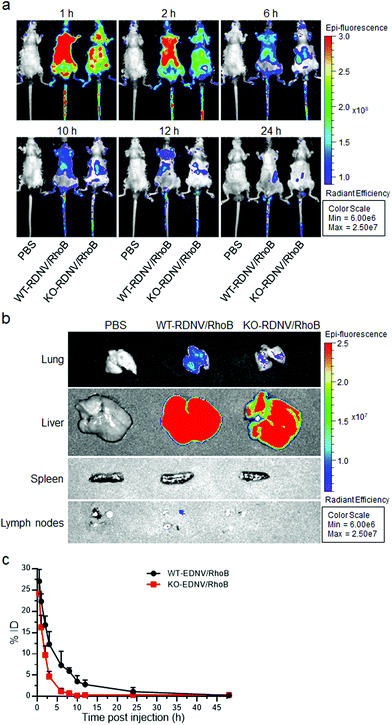 |
| Fig. 4 Biodistribution and metabolism of drug-loaded WT-RDNVs or KO-RDNVs in C57BL/6 mice. C57BL/6 mice were administered with WT-RDNV/RhoB, KO-RDNV/RhoB, or the PBS control once by i.v. injection. (a) The time-lapse rhodamine B fluorescence images of the mice, and (b) the fluorescence images of the indicated tissues harvested at 24 h after the injection initiated with the IVIS Xenogen imaging system. (c) Pharmacokinetic data of WT-RDNV/RhoB or KO-RDNV/RhoB in C57BL/6 mice (n = 3). The data are presented as mean ± SD. | |
Clodronate can induce irreversible damage leading to apoptosis in macrophages after exceeding a threshold intracellular concentration. We further studied whether CD47 affects the efficacy of clodronate-loaded RDNVs in targeting macrophage delivery by evaluating the phagocytosis and number of macrophages in C57BL/6 mice. The phagocytosis ability of macrophages in vivo was assessed by comparing the survival of CD47−/− RBCs in C57BL/6 mice administered WT-RDNV/CLD or KO-RDNV/CLD; mice treated with PBS or Clodrosome were the negative and positive controls, respectively. CFSEhi CD47−/− RBCs were rapidly cleared and became undetectable by 48 hours after injection into the PBS-treated mice. This process was postponed for 96 hours in Clodrosome-treated mice (Fig. 5a and b). In contrast, the clearance of CD47−/− RBCs was significantly limited in mice treated with WT-RDNV/CLD or KO-RDNV/CLD (Fig. 5a and b). In mice administered KO-RDNV/CLD, the CD47−/− RBCs began to be cleared 72 hours after injection and remained at 28.6 ± 8.5% at the end of this experiment (Fig. 5b).
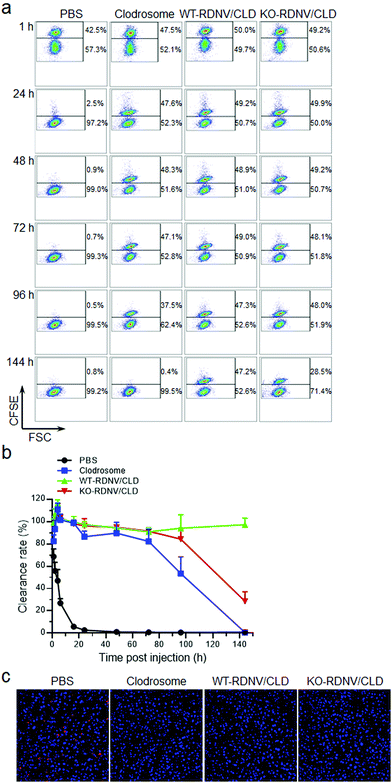 |
| Fig. 5 Macrophage depletion by WT-RDNV/CLD and KO-RDNV/CLD in C57BL/6 mice. C57BL/6 mice were administered with Clodrosome, WT-RDNV/CLD, KO-RDNV/CLD, or the PBS control with equal amounts of clodronate by i.v. injection every day for 2 days. The doses of injected clodronate in each group were 25 and 12.5 μg per g body weight per day, respectively. (a, b) The mixture of CFSE-stained CD47−/− RBCs (1 × 108) and CD47+/+ RBCs (1 × 108) was intravenously injected into the mice at 6 h after the last injection of WT-RDNV/CLD, KO-RDNV/CLD, Clodrosome or the PBS control (n = 4). Blood was collected at the indicated time points, and the levels (percentages) of injected CD47−/− and CD47+/+ RBCs were analyzed by flow cytometry. (a) Representative flow cytometry profiles. (b) Ratio (mean ± SD) of CFSEhi CD47−/− to CFSElow CD47+/+ RBCs at the indicated times. (c) CLSM images show the CD68+ Kupffer cells in the liver of mice at 24 h after the last injection of WT-RDNV/CLD, KO-RDNV/CLD, Clodrosome, or the PBS control. The cell nuclei were stained with DAPI (blue), and the macrophages were stained with Alexa Fluor488-CD68 (red). | |
The livers were harvested from WT-RDNV/CLD-, KO-RDNV/CLD-, Clodrosome-, and PBS-treated mice at 24 h after i.v. injection. The macrophages in these organs were visualized by immunohistochemistry staining of CD68—a marker of phagocytic myeloid cells including macrophages, dendritic cells, and neutrophils.25–28 Confocal laser scanning microscopy revealed a marked reduction in CD68+ cells in the livers of mice treated with WT-RDNV/CLD, KO-RDNV/CLD, or Clodrosome (Fig. 5c). Phagocytosing Kupffer cells in the liver were almost depleted at 24 h after i.v. injection of WT-RDNV/CLD or KO-RDNV/CLD—this was similar to Clodrosome. These data indicate that these erythrocyte-based nanovesicles can be excellent macrophage-targeted drug delivery systems. The significantly longer macrophage clearance results from the prolonged circulation of clodronate-loaded WT-RDNVs demonstrating the key role of surface CD47 for RDNVs as a drug carrier.
Safety of clodronate-loaded erythrocyte derived nanovesicles
The safety of nanomedicine is important and has attracted increasing attention.29,30 To evaluate the in vivo toxicity of clodronate-loaded WT-RDNVs and KO-RDNVs, C57BL/6 mice were administered WT-RDNV/CLD, KO-RDNV/CLD, Clodrosome, and PBS at clodronate doses of 25 and 12.5 μg per g body weight at day 0 and day 1, respectively. The histological findings of the tissues collected from mice 24 h after the final injection are shown in Fig. S2.† All tissues from mice treated with WT-RDNV/CLD or KO-RDNV/CLD appeared normal similar to those of the PBS controls. An emphysema-like phenotype was found in the lungs of Clodrosome-treated mice. Moreover, Clodrosome is believed to have no apparent direct effects on cells other than mononuclear phagocytes, but it has obvious toxicity to immunodeficient mice (such as NSG mice) when administered chronically. Therefore, it is critical to evaluate the long-term safety of clodronate-loaded erythrocyte-derived nanovesicles using NSG mice. NSG mice were administered WT-RDNV/CLD, KO-RDNV/CLD, Clodrosome or PBS every day for 21 days. The dose of clodronate was 25 μg per g body weight at day 0 and 12.5 μg per g body weight per day for the remaining 20 days. The Clodrosome treatment caused significant body-weight loss starting on the 10th day (Fig. 6a) and death on the 16th day (Fig. 6b) in the NSG mice. However, the administration of WT-RDNV/CLD or KO-RDNV/CLD had no in vivo toxicity to NSG mice (Fig. 6a and b). These results suggest that erythrocyte-derived nanovesicles as in vivo drug delivery vehicles have better biocompatibility and safety versus artificially prepared drug carriers.
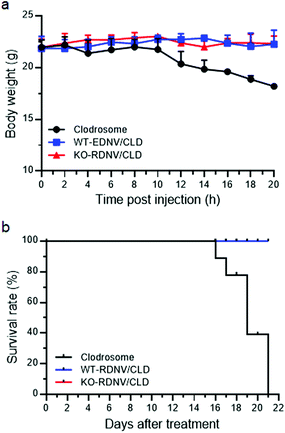 |
| Fig. 6 Lack of significant toxicity in mice receiving long-term injection of WT-RDNV/CLD or KO-RDNV/CLD. (a) Kinetics of body weight changes and (b) the survival ratio of NSG mice that received Clodrosome, WT-RDNV/CLD, or KO-RDNV/CLD with equal amounts of clodronate every day for 21 days (n = 5 per group). The doses of injected clodronate were 25 μg per g body weight at day 0 and 12.5 μg per g body weight per day for the remaining 20 days. | |
Conclusions
This study showed that nanovesicles derived from autologous erythrocytes represent a new class of long-circulating nanocarriers and can be used to specifically deliver drug payloads into macrophages—a therapeutic target for many diseases. More importantly, to the best of our knowledge, this is the first report to show that CD47 plays a crucial role in the long circulation and biodistribution of erythrocyte-derived nanovesicles. Compared to KO-RDNV/CLD, clodronate-encapsulated WT-RDNVs offer efficient, stable, and long-term macrophage depletion in homologous mice indicating the excellent ability of WT-RDNVs to target macrophages for drug delivery. Finally, erythrocyte-derived nanovesicles show no obvious toxicity to mice even in animals receiving long-term administration. In summary, this RBC membrane-based nanoparticle technology has significant clinical potential.
Conflicts of interest
There are no conflicts to declare.
Acknowledgements
This work was supported by grants from the Chinese MOST (973 Program 2015CB964400), the National Key Research and Development Program of China (2017YFA0208100), the NSFC (81571798, 81422026, 91642208, 81570145, 81871478, 81870091, 91742107), the Special Fund Project of Jilin Province Provincial Industrial Innovation (2018C052-1), the Norman Bethune Program of Jilin University (2015207) and the Interdisciplinary Innovation Project of the First Hospital of Jilin University (JDYYJCHX001).
References
- K. R. Peterson, M. A. Cottam, A. J. Kennedy and A. H. Hasty, Macrophage-Targeted Therapeutics for Metabolic Disease, Trends Pharmacol. Sci., 2018, 39(6), 536–546 CrossRef CAS PubMed.
- H. Herwald and A. Egesten, Macrophages: Past, Present and Future, J. Innate Immun., 2013, 5, 657–658 CrossRef PubMed.
- V. Bronte and P. J. Murray, Understanding local macrophage phenotypes in disease: modulating macrophage function to treat cancer, Nat. Med., 2015, 21(2), 117–119 CrossRef CAS PubMed.
- A. Mantovani, F. Marchesi, A. Malesci, L. Laghi and P. Allavena, Tumour-associated macrophages as treatment targets in oncology, Nat. Rev. Clin. Oncol., 2017, 14(7), 399–416 CrossRef CAS PubMed.
- C. Ju and F. Tacke, Hepatic macrophages in homeostasis and liver diseases: from pathogenesis to novel therapeutic strategies, Cell. Mol. Immunol., 2016, 13(3), 316–327 CrossRef CAS PubMed.
- S. K. Patel and J. M. Janjic, Macrophage targeted theranostics as personalized nanomedicine strategies for inflammatory diseases, Theranostics, 2015, 5(2), 150–172 CrossRef CAS PubMed.
- E. L. Mills and L. A. O'Neill, Reprogramming mitochondrial metabolism in macrophages as an anti-inflammatory signal, Eur. J. Immunol., 2016, 46(1), 13–21 CrossRef CAS PubMed.
- A. E. Pentecost, C. E. Witherel, Y. Gogotsi and K. L. Spiller, Anti-inflammatory effects of octadecylamine-functionalized nanodiamond on primary human macrophages, Biomater. Sci., 2017, 5(10), 2131–2143 RSC.
- C. H. Ries, M. A. Cannarile, S. Hoves, J. Benz, K. Wartha, V. Runza, F. Rey-Giraud, L. P. Pradel, F. Feuerhake, I. Klaman, T. Jones, U. Jucknischke, S. Scheiblich, K. Kaluza, I. H. Gorr, A. Walz, K. Abiraj, P. A. Cassier, A. Sica, C. Gomez-Roca, K. E. de Visser, A. Italiano, C. Le Tourneau, J. P. Delord, H. Levitsky, J. Y. Blay and D. Rüttinger, Targeting tumor-associated macrophages with anti-CSF-1R antibody reveals a strategy for cancer therapy, Cancer Cell, 2014, 25(6), 846–859 CrossRef CAS PubMed.
- C. Baeck, X. Wei, M. Bartneck, V. Fech, F. Heymann, N. Gassler, K. Hittatiya, D. Eulberg, T. Luedde, C. Trautwein and F. Tacke, Pharmacological inhibition of the chemokine C-C motif chemokine ligand 2 (monocyte chemoattractant protein 1) accelerates liver fibrosis regression by suppressing Ly-6C(+) macrophage infiltration in mice, Hepatology, 2014, 59(3), 1060–1072 CrossRef PubMed.
- R. Weissleder, M. Nahrendorf and M. J. Pittet, Imaging macrophages with nanoparticles, Nat. Mater., 2014, 13, 125 CrossRef CAS PubMed.
- M. Medina-Sanchez, H. Xu and O. G. Schmidt, Micro- and nano-motors: the new generation of drug carriers, Ther. Delivery, 2018, 9(4), 303–316 CrossRef CAS PubMed.
- X. Liu, M. J. Yaszemski and L. Lu, Expansile crosslinked polymersomes for pH sensitive delivery of doxorubicin, Biomater. Sci., 2016, 4(2), 245–249 RSC.
- T. Sun, Y. S. Zhang, B. Pang, D. C. Hyun, M. Yang and Y. Xia, Engineered nanoparticles for drug delivery in cancer therapy, Angew. Chem., Int. Ed., 2014, 53(46), 12320–12364 CAS.
- K. Cai, J. Yen, Q. Yin, Y. Liu, Z. Song, S. Lezmi, Y. Zhang, X. Yang, W. G. Helferich and J. Cheng, Redox-Responsive Self-Assembled Chain-Shattering Polymeric Therapeutics, Biomater. Sci., 2015, 3(7), 1061–1065 RSC.
- T. Z. Chang, S. S. Stadmiller, E. Staskevicius and J. A. Champion, Effects of ovalbumin protein nanoparticle vaccine size and coating on dendritic cell processing, Biomater. Sci., 2017, 5(2), 223–233 RSC.
- V. R. Muzykantov, Drug delivery by red blood cells: vascular carriers designed by mother nature, Expert Opin. Drug Delivery, 2010, 7(4), 403–427 CrossRef CAS PubMed.
- C. M. Hu, L. Zhang, S. Aryal, C. Cheung, R. H. Fang and L. Zhang, Erythrocyte membrane-camouflaged polymeric nanoparticles as a biomimetic delivery platform, Proc. Natl. Acad. Sci. U. S. A., 2011, 108(27), 10980–10985 CrossRef CAS PubMed.
- P. A. Oldenborg, A. Zheleznyak, Y. F. Fang, C. F. Lagenaur, H. D. Gresham and F. P. Lindberg, Role of CD47 as a marker of self on red blood cells, Science, 2000, 288(5473), 2051–2054 CrossRef CAS PubMed.
- R. H. Fang, C. M. Hu and L. Zhang, Nanoparticles disguised as red blood cells to evade the immune system, Expert Opin. Biol. Ther., 2012, 12(4), 385–389 CrossRef CAS PubMed.
- K. Jin, Z. Luo, B. Zhang and Z. Pang, Biomimetic nanoparticles for inflammation targeting, Acta Pharm. Sin. B, 2018, 8(1), 23–33 CrossRef PubMed.
- H. Wang, M. L. Madariaga, S. Wang, N. Van Rooijen, P. A. Oldenborg and Y. G. Yang, Lack of CD47 on nonhematopoietic cells induces split macrophage tolerance to CD47null cells, Proc. Natl. Acad. Sci. U. S. A., 2007, 104(34), 13744–13749 CrossRef CAS PubMed.
- S. Jaiswal, M. P. Chao, R. Majeti and I. L. Weissman, Macrophages as mediators of tumor immunosurveillance, Trends Immunol., 2010, 31(6), 212–219 CrossRef CAS PubMed.
- P. J. Murray and T. A. Wynn, Protective and pathogenic functions of macrophage subsets, Nat. Rev. Immunol., 2011, 11(11), 723–737 CrossRef CAS PubMed.
- S. Liu, H. Chen, T. Wu, G. Pan, C. Fan, Y. Xu and W. Cui, Macrophage infiltration of electrospun polyester fibers, Biomater. Sci., 2017, 5(8), 1579–1587 RSC.
- E. Kunisch, R. Fuhrmann, A. Roth, R. Winter, W. Lungershausen and R. W. Kinne, Macrophage specificity of three anti-CD68 monoclonal antibodies (KP1, EBM11, and PGM1) widely used for immunohistochemistry and flow cytometry, Ann. Rheum. Dis., 2004, 63(7), 774–784 CrossRef CAS PubMed.
- S. W. Cho, F. N. Soki, A. J. Koh, M. R. Eber, P. Entezami, S. I. Park, N. van Rooijen and L. K. McCauley, Osteal macrophages support physiologic skeletal remodeling and anabolic actions of parathyroid hormone in bone, Proc. Natl. Acad. Sci. U. S. A., 2014, 111(4), 1545–1550 CrossRef CAS PubMed.
- V. A. Kumar, J. M. Caves, C. A. Haller, E. Dai, L. Li, S. Grainger and E. L. Chaikof, Collagen-Based Substrates with Tunable Strength for Soft Tissue Engineering, Biomater. Sci., 2013, 1(11), 1193–1202 RSC.
- N. Desai, Challenges in development of nanoparticle-based therapeutics, AAPS J., 2012, 14(2), 282–295 CrossRef CAS PubMed.
- A. Vashist, A. Kaushik, A. Vashist, R. D. Jayant, A. Tomitaka, S. Ahmad, Y. K. Gupta and M. Nair, Recent trends on hydrogel based drug delivery systems for infectious diseases, Biomater. Sci., 2016, 4(11), 1535–1553 RSC.
Footnote |
† Electronic supplementary information (ESI) available. See DOI: 10.1039/c8bm01258j |
|
This journal is © The Royal Society of Chemistry 2019 |
Click here to see how this site uses Cookies. View our privacy policy here.