DOI:
10.1039/C9RA00622B
(Paper)
RSC Adv., 2019,
9, 10554-10560
Highly selective and sensitive fluorescent probe for the rapid detection of mercury ions
Received
24th January 2019
, Accepted 20th March 2019
First published on 4th April 2019
Abstract
Mercury (Hg) is one of the major toxic heavy metals, harmful to the environment and human health. Thus, it is significantly important to find an easy and quick method to detect Hg2+. In this study, we designed and synthesized a simple fluorescent probe with excellent properties, such as high sensitivity and selectivity, rapid response, and outstanding water solubility. When Hg2+ (5 μM) was added to the probe solution, it exhibited a very large fluorescent enhancement (about 350-fold stronger than the free probe) with the help of hydrogen peroxide (H2O2). Probe HCDC could quantitatively detect Hg2+ in the range of 0–10 μM using the fluorescence spectroscopy method and the detection limit was measured to be about 0.3 nM (based on a 3σ/slope). Analytical application was also studied, and the probe HCDC exhibited excellent response to Hg2+ with the addition of H2O2 in real water samples. So, our proposed probe HCDC provided a practical and promising method for determining Hg2+ in the environment.
1. Introduction
Heavy metal ion pollution has become a global environmental problem.1–3 As a common metal element, mercury (Hg) is extremely toxic and shows a great threat to the environment and human health due to its bioaccumulation, durability, and easy absorption by aquatic organisms.4–10 Once the mercury enters the organism, it will lead to the dysfunctions of the central nervous systems, the brain, and the kidneys.11–14 As we all know, the maximum contaminant level of mercury in drinking water is 2 ppb (0.01 μM) which was set by the Environment Protection Agency (EPA).15–18 It can be seen that mercury could pose a serious threat to humans at very low concentrations. Thus, developing a convenient, rapid, highly selective and sensitive method for the determination of Hg2+ is vitally important.
Nowadays, several classical methods have been applied to determine Hg2+ including electrochemical analysis, atomic absorption/emission spectroscopy, electrochemical analysis, and inductively coupled plasma mass spectrometry.19–23 Though these methods are selective and accurate to detect ions, most of them require long time, expensive instruments, and tedious sample pre-treatment.24–26 Thus, the techniques of fluorescent probes were widely studied due to the advantages of highly selective, operational simplicity, and relatively low-cost.27–33 Many fluorescent probes for detecting Hg2+ have been reported; however, most of them were still limited by the disadvantages of low sensitivity, bad water solubility, and long response time.34–38 So, a simple water-soluble fluorescent probe for determining Hg2+ with high sensitivity and quick response became our primary task.
Very recently, we have reported several carbonothioate-based fluorescent probe for detecting Hg2+. These probes respectively chose the 2-(2′-hydroxyphenyl)benzothiazole (HBT),39 dichlororesorufin,40 and seminaphthorhodafluor41 as the fluorophores, and a carbonothioate moiety was used as the recognition receptor. All these showed quick response and high selectivity for detecting Hg2+. In connection with our continuing research, we designed a new probe HCDC (Scheme 1) for detecting Hg2+ with superior properties in this paper, and the property of probe HCDC was compared with other reported studies in Table 1. A dimethyl-thiocarbamic ester group and 7-hydroxycoumarin were respectively chosen as the recognition receptor and the fluorophore. We assumed that the thioester group changed to ester moiety in the presence of Hg2+, and the rapid cleavage of ester group was achieved with the help of H2O2. As a result, this restored the blue fluorescence of 7-hydroxycoumarin. The experimental results demonstrated that this probe possessed the advantages of (1) a very low detection limit of 0.3 nM (on the basis of 3σ/slope); (2) quicker response to Hg2+; (3) excellent water solubility, selectivity and anti-interference; (4) practical applicability in real water samples.
 |
| Scheme 1 Synthesis of probe HCDC. | |
Table 1 Comparison of Fluorescent Probes for Hg2+
Probe |
LOD (nM) |
Solution (v/v) |
Time (min) |
References |
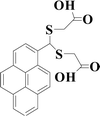 |
1.8 |
Ethanol : PBS = 2 : 1 |
30 |
34 |
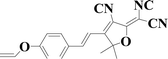 |
10 |
C2H5OH : water = 1 : 1 |
40 |
35 |
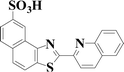 |
34 |
CH3CN : water = 1 : 1 |
— |
36 |
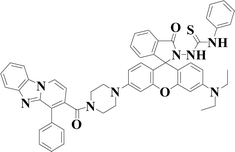 |
18.8 |
C2H5OH : water = 2 : 8 |
20 |
37 |
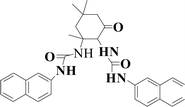 |
100 |
DMSO : H2O = 9 : 1 |
30 |
38 |
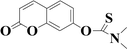 |
0.3 |
Aqueous solution |
10 |
This work |
2. Experimental
2.1 Materials and general methods
All the chemicals were gained from commercial suppliers and were used without further purification. The Sartorius Arium 611DI system can produce the ultra-pure water which was used during the entire process of the experiment. Column chromatography using Silica gel 200–300 mesh from the Qingdao Haiyang Chemical Co was used to purify the reaction product. The 1H NMR and 13C NMR were all recorded on the Bruker AV-400 spectrometer with chemical shifts reported as ppm (in DMSO-d6, TMS as internal standard). Fluorescence spectra were gained from a Horiba FluoroMax-4 spectrophotometer. All the fluorescence spectra were uncorrected. Probe HCDC was prepared according to the previous paper.42
2.2 General procedure for analysis
Stock solutions of probe HCDC (1 mM) were prepared in ethanol. And the parent stock solutions (1 mM) of Hg2+, other metal ions and common anions species (10 mM) were prepared in ultrapure water. The test solution contained 50 μL of the probe stock solution and moderate amount of each stock solution, and eventually diluted to 10 mL in a test tube with 100% ultrapure water containing HEPES (5 mM, pH 7.4). Before Hg2+ and other ions were added to the test solution, H2O2 (3 mM) was added. All the tests were conducted at room temperature (25 °C).
2.3 Determination of the detection limit
According to the previous paper,43,44 the method of fluorescence titration was used to calculate the detection limit. The fluorescence emission spectrum of probe HCDC was measured by fifteen times in the absence of Hg2+ in order to achieve the standard deviation of blank measurement. To gain the slope, the fluorescence intensity at 455 nm was plotted as a concentration of Hg2+. Therefore, the formula for calculating the detection limit is described as following:
where σ is the standard deviation of the five spectrums among the fifteen spectrums which peaks were nearest, k is the slope between the Hg2+ concentration versus the fluorescence intensity.
2.4 Analytical application
Three water samples were chosen to test the analytical application of the probe HCDC. One water sample was collected from Jia Zi Lake, University of Jinan, China, and the other two samples were respectively collected from the Xiu Jiang River and Yellow River, at Jinan, China. All the samples needed to filter through filter paper before the measurement.
3. Results and discussion
3.1 Characteristic spectra
In this paper, the fluorescence and absorption spectra of probe HCDC was shown as Fig. 1. In the absence of Hg2+ and H2O2, the probe solution exhibits a very weak fluorescence emission peak at 455 nm. When only Hg2+ was added to the probe solution, the reaction was very slow. And, the reaction was not carried out when only the H2O2 was added. Once Hg2+ and H2O2 were all added to the probe HCDC solution, the maximum fluorescence emission peak exhibits a very large enhancement at 455 nm (Fig. 1a). The fluorescence intensity peak of the probe solution containing Hg2+ and H2O2 was about 350-fold stronger than that of the blank probe solution. Meanwhile, in the absorption spectra, with the addition of Hg2+ and H2O2, the probe solution exhibited a new absorption peak at 364 nm (Fig. 1b). The result showed that Hg2+ could generate the cleavage of an eater group in the presence of H2O2 (Scheme 2).40
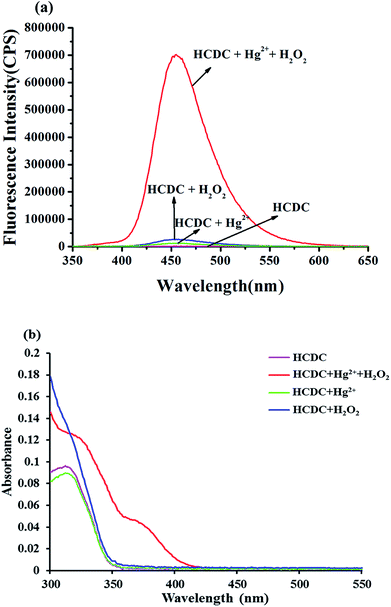 |
| Fig. 1 Fluorescence spectra (a) and absorption spectra (b) of probe HCDC (5 μM) toward Hg2+ (5 μM) and H2O2 (3 mM) under the ultra-pure water containing HEPES (5 mM, pH 7.4). | |
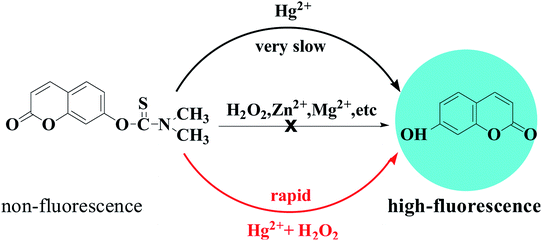 |
| Scheme 2 Reaction Mechanism of probe HCDC for Hg2+ with the help of H2O2. | |
3.2 Effect of H2O2
Different concentrations of H2O2 was respectively added to the solution of probe HCDC (5 μM) and Hg2+ (5 μM) in the aqueous solution which contained HEPES (5 mM, pH 7.4). It was shown in inset of Fig. 2, the fluorescence intensity was increased first and then decreased with the increasing concentrations of H2O2 (the range of 1 to 40 mM). The enhancement of fluorescence reached the maximum when the concentration of H2O2 was 3 mM. The results implied that the low concentration of H2O2 (1 to 3 mM) could promote the cleavage of ester group and amplify the response of probe HCDC to Hg2+. But when the concentration is over 3 mM (4 to 40 mM), H2O2 will inhibit the response of probe HCDC to Hg2+. Thus, we chose 3 mM H2O2 as the amplification reagent in the detection of Hg2+.
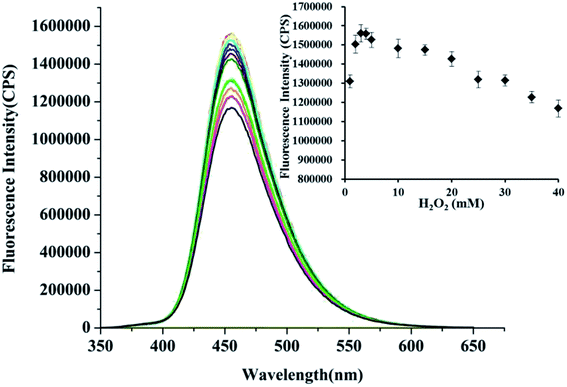 |
| Fig. 2 Fluorescence spectra of probe HCDC (5 μM) in the presence of Hg2+ (5 μM) and different concentrations of H2O2 (final concentration: 0, 1, 2, 3, 4, 5, 10, 15, 20, 25, 30, 35, 40 mM) under HEPES (5 mM, pH 7.4) aqueous solution. Excitation wavelength = 330 nm. Error bar = RSD (n = 3). | |
3.3 Time dependence of detecting Hg2+
Response time is a significant element to test the effect of the probe. So, the response time of probe HCDC was investigated under the analytical conditions which were mentioned above. The result was shown in Fig. 3. When only Hg2+ was added to the probe solution, the reaction was very slow. While, the fluorescence intensity at 455 nm was increased as soon as Hg2+ and H2O2 were added, and increased gradually until the reaction time exceeded 10 min. Thus, all the measurements of the spectrum were carried out 10 minutes after the addition of Hg2+. The results showed that the probe HCDC could provide a rapid analytical method for detecting Hg2+.
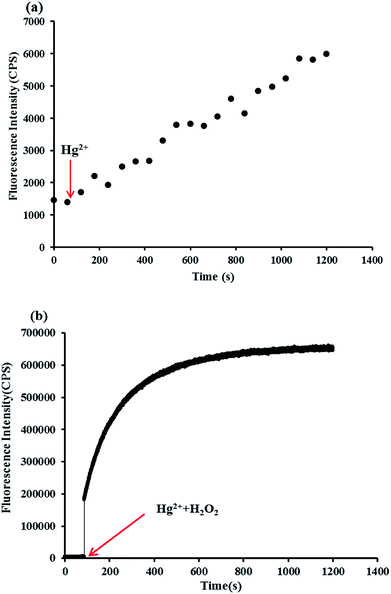 |
| Fig. 3 Reaction time for probe HCDC (5 μM) with the addition of only Hg2+ (5 μM) (a), and Hg2+ (5 μM) and H2O2 (3 mM) (b). | |
3.4 Quantification of Hg2+
As shown in Fig. 4, the continuous enhancement of fluorescence intensity at 455 nm can be seen with the continuous addition of Hg2+ (the range of 0 to 10 μM). And, there was a good linearity (y = 225439x + 3899.2, R2 = 0.998) between the Hg2+ concentrations of 0–2 μM and the fluorescence intensity with a detection limit of 0.3 nM (based on 3σ/slope). The detection limit of the probe HCDC was far lower than the standard of Hg2+ (10 nM) in drinking water which set up by the Environmental Protection Agency (EPA). The above results showed that the probe HCDC could detect Hg2+ quantitatively by the fluorescence spectrometry method with excellent sensitivity.
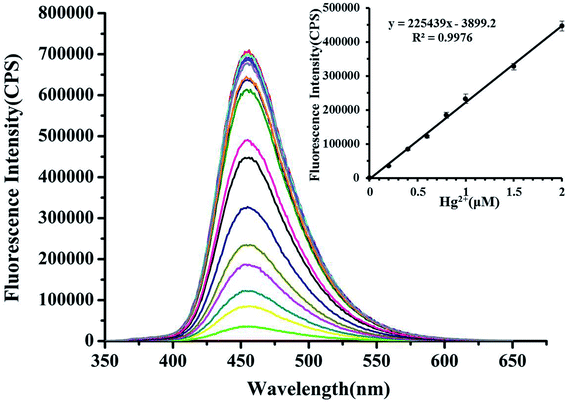 |
| Fig. 4 Fluorescence spectra of probe HCDC (5 μM) toward the various concentrations of Hg2+ (final concentration: 0, 0.2, 0.4, 0.6, 0.8, 1, 1.5, 2, 2.5, 3, 3.5, 4, 4.5, 5, 6, 7, 8, 9, 10 μM) and H2O2 (3 mM). The fluorescence intensity at 455 nm of probe HCDC increased with the increasing concentrations of Hg2+. Excitation wavelength = 330 nm, emission wavelength = 455 nm. Conditions: in HEPES (5 mM, pH 7.4). Solution: ultrapure water. Error bar = RSD (n = 3). | |
3.5 Selectivity to Hg2+
High selectivity is also an important factor for an excellent probe. The selectivity of HCDC toward Hg2+ was evaluated under the above-mentioned conditions. The effect of the metal ions (Mg2+, Zn2+, Ni2+, Ca2+, Na+, K+, Pb2+, Cd2+, Cr6+, Fe2+, Fe3+, Sn2+) and the common anions species (NO2−, NO3−, HCO3−, H2PO4−, F−, CO32−, Cl−, SO42−) on fluorescence spectra of probe HCDC were investigated. Firstly, Hg2+, other metal ions and common anions species were respectively added to the solution, and only Hg2+ resulted in a considerable fluorescence enhancement (Fig. 5a and c). The results exhibited that HCDC possesses high selectivity toward Hg2+.
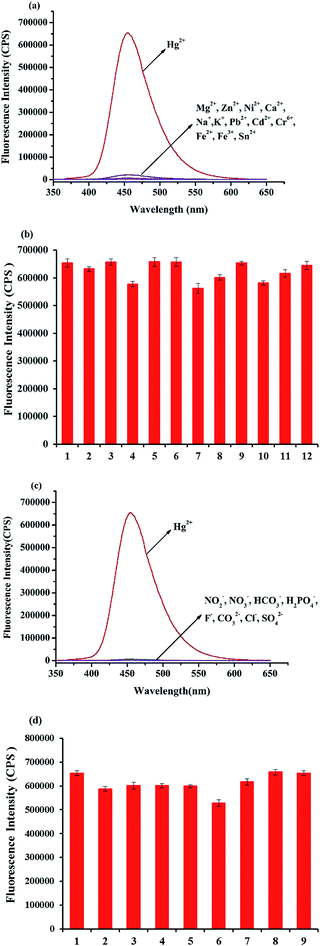 |
| Fig. 5 (a) Fluorescence responses of probe HCDC (5 μM) toward Hg2+ (5 μM), and other metal ions (100 μM). (b) Fluorescence responses of probe HCDC toward Hg2+ and in presence of other metal ions. (1) Hg2+; (2) Mg2+; (3) Zn2+; (4) Sn2+; (5) Ca2+; (6) Na+; (7) Fe3+; (8) Pb2+; (9) Cd2+; (10) Fe2+; (11) K+; (12) Ni2+. (c) Fluorescence responses of HCDC (5 μM) toward Hg2+ (5 μM), and common anions species (100 μM). (d) Fluorescence responses of probe HCDC toward Hg2+ and in presence of other common anions species (1) Hg2+; (2) NO2−; (3) NO3−; (4) HCO3−; (5) H2PO4−; (6) F−; (7) CO32−; (8) Cl−; (9) SO42−. The solution: in H2O2 (3 mM), HEPES (5 mM, pH 7.4) aqueous solution. Error bar = RSD (n = 3). | |
The interference of the metal ions and common anions species on the detection of Hg2+ was also studied. Even though the mental ions and common anions species were respectively existed in the solution, the probe HCDC also had a good response to Hg2+ (Fig. 5b and d). These studies clearly indicated that the probe HCDC could be used for the selective detection of Hg2+ without interference from anions.
3.6 Analytical application
Based on the above results, the probe HCDC owned the excellent properties containing a very low detection limit, quicker response, excellent water solubility, selectivity and anti-interference. So, we attempted to investigate the practical application of the probe for the selective detection of Hg2+ in three real water samples. The results were shown in Table 2. At first, there were no Hg2+ in three water samples, and then 2 μM, 5 μM, or 10 μM Hg2+ was respectively added to the solution of real water (5 mM, HEPES, pH 7.4) containing 5 μM probe HCDC and 3 mM H2O2. Every sample was repeatedly configured and tested for 3 times. Therefore, we obtained a good method for detecting Hg2+ in the environment.
Table 2 Analysis results of Hg2+ in three real water samples a
Real water sample |
Found Hg2+ |
Addition Hg2+(μM) |
Found (μM) |
Recovery (%) |
RSD (n = 3) (%) |
Sample A, lake water from JiaZi Lake, University of Jinan; sample B, from the Xiu Jiang River, at Jinan, China; sample C, from the Yellow River, at Jinan, China. |
Sample A |
No |
2 |
1.82 ± 0.06 |
91.06 |
2.89 |
|
5 |
4.52 ± 0.11 |
90.47 |
2.10 |
|
10 |
9.61 ± 0.40 |
96.06 |
4.02 |
Sample B |
No |
2 |
1.85 ± 0.11 |
92.52 |
5.36 |
|
5 |
4.97 ± 0.29 |
99.33 |
5.83 |
|
10 |
10.11 ± 0.25 |
101.11 |
2.51 |
Sample C |
No |
2 |
2.24 ± 0.13 |
111.93 |
6.56 |
|
5 |
5.15 ± 0.21 |
102.96 |
4.19 |
|
10 |
9.73 ± 0.37 |
97.28 |
3.72 |
4. Conclusion
In a word, we have designed and synthesized a highly sensitive and selective probe for detecting Hg2+ by the fluorescence spectrum. The enhancement of fluorescence intensity of the probe solution containing Hg2+ and H2O2 was very significant, which could reach 350-fold stronger than that of the blank probe solution. There was a good linear relationship in the range of 0–2 μM Hg2+, and the low detection limit was 0.3 nM (on the basis of 3σ/slope). The probe showed excellent solubility in water. More importantly, the response of probe HCDC to Hg2+ in real water samples was also satisfactory. All these remarkable advantages of this new probe suggest that this probe will have excellent application prospect in the detection of mercury.
Conflicts of interest
There are no conflicts of interest to declare.
Acknowledgements
This work was supported by the National Nature Science Foundation of China (No. 21777053), Major Livelihood Projects of Jinan (SHMS2015-3-01), the Municipal Development and Reform Commission Project of Jinan (2016JSFW02Z0307), and Water Conservancy Science and Technology project of Shandong Province (SDSLKY201606).
References
- J. Wu, W. Jiang, A. Deng, Y. Shen, W. Jiang and R. Tian, Sens. Actuators, B, 2016, 234, 691–702 CrossRef CAS.
- R. Zhang and W. Chen, Biosens. Bioelectron., 2014, 55, 83–90 CrossRef CAS PubMed.
- B. Zhu, J. Zhao, H. Yu, L. Yan, Q. Wei and B. Du, Opt. Mater., 2013, 35, 2220–2225 CrossRef CAS.
- K. Kala, P. Vineetha and N. Manoj, New J. Chem., 2017, 41, 5176–5181 RSC.
- Y. Ge, X. Xing, A. Liu, R. Ji, S. Shen and X. Cao, Dyes Pigm., 2017, 146, 136–142 CrossRef CAS.
- Y. Zhou, X. He, H. Chen, Y. Wang, S. Xiao, N. Zhang, D. Li and K. Zheng, Sens. Actuators, B, 2017, 247, 626–631 CrossRef CAS.
- R. Zou, X. Zeng, G. Luo, Y. Qiu, B. Zhang, Y. Xu, H. Wu and H. Yao, Fuel, 2016, 186, 215–221 CrossRef CAS.
- W. Shi, S. Zhao, Y. Su, Y. Hui and Z. Xie, New J. Chem., 2016, 40, 7814–7820 RSC.
- S. Ding, M. Dong, Y. Wang, Y. Chen, H. Wang, C. Su and W. Wang, J. Am. Chem. Soc., 2016, 138, 3031–3037 CrossRef CAS PubMed.
- S. Zhang, J. Geng, W. Yang and X. Zhang, RSC Adv., 2014, 4, 12596–12600 RSC.
- Q. Mei, R. Tian, Y. Shi, Q. Hua, C. Chen and B. Tong, New J. Chem., 2016, 40, 2333–2342 RSC.
- J. Zang, C. Li, K. Zhou, H. Dong, B. Chen, F. Wang and G. Zhao, Anal. Chem., 2016, 88, 10275–10283 CrossRef CAS PubMed.
- C. Zhang, L. Zou, D. Wang and L. Zhang, J. Photochem. Photobiol., A, 2017, 332, 440–452 CrossRef CAS.
- A. Afaneh and G. Schreckenbach, J. Phys. Chem. A, 2015, 119, 8106–8116 CrossRef CAS PubMed.
- A. Kora and L. Rastogi, Sens. Actuators, B, 2018, 254, 690–700 CrossRef CAS.
- M. Wani, N. Thakur, M. Pandey and R. Pandey, New J. Chem., 2017, 41, 10000–10008 RSC.
- N. Sakly, W. Marzouk, H. Ouada and H. Majdoub, Sens. Actuators, B, 2017, 253, 918–927 CrossRef CAS.
- L. Yang, Y. Su, Y. Geng, H. Xiong, J. Han, Q. Fang and X. Song, Org. Biomol. Chem., 2018, 16, 5036–5042 RSC.
- N. Wang, M. Lin, H. Dai and H. Ma, Biosens. Bioelectron., 2016, 79, 320–326 CrossRef CAS PubMed.
- N. Mir, A. Heidari, H. Beyzaei, S. Mirkazehi-Rigi and P. Karimi, Chem. Eng. J., 2017, 327, 648–655 CrossRef CAS.
- H. Cheng, C. Wu, L. Shen, J. Liu and Z. Xu, Anal. Chim. Acta, 2014, 828, 9–16 CrossRef CAS PubMed.
- H. Agarwalla, P. Mahajan, D. Sahu, N. Taye, B. Ganguly, S. Mhaske, S. Chattopadhyay and A. Das, Inorg. Chem., 2016, 55, 12052–12060 CrossRef CAS PubMed.
- D. Dinda, B. Shaw and S. Saha, ACS Appl. Mater. Interfaces, 2015, 7, 14743–14749 CrossRef CAS PubMed.
- H. Zhou, L. Sun, W. Chen, G. Tian, Y. Chen, Y. Li and J. Su, Tetrahedron, 2016, 72, 2300–2305 CrossRef CAS.
- Q. Wang, Y. Xu, Y. Hou, Y. Wang, M. Yan, X. Zhang and H. Wang, RSC Adv., 2016, 6, 114685–114689 RSC.
- W. Liu, D. Zhang, B. Ni, J. Li, H. Weng and Y. Ye, Sens. Actuators, B, 2019, 284, 330–336 CrossRef CAS.
- X. Yang, W. Liu, J. Tang, P. Li, H. Weng, Y. Ye, M. Xian, B. Tang and Y. Zhao, Chem. Commun., 2018, 54, 11387–11390 RSC.
- B. Zhu, Z. Wang, Z. Zhao, W. Shu, M. Zhang, L. Wu, C. Liu, Q. Duan and P. Jia, Sens. Actuators, B, 2018, 262, 380–385 CrossRef CAS.
- B. Zhang, X. Yang, R. Zhang, Y. Liu, X. Ren, M. Xian, Y. Ye and Y. Zhao, Anal. Chem., 2017, 89, 10384–10390 CrossRef CAS PubMed.
- B. Zhu, L. Wu, H. Zhu, Z. Wang, Q. Duan, Z. Fang, P. Jia, Z. Li and C. Liu, Sens. Actuators, B, 2018, 269, 1–7 CrossRef CAS.
- J. Xu, J. Pan, X. Jiang, C. Qin, L. Zeng, H. Zhang and J. Zhang, Biosens. Bioelectron., 2016, 77, 725–732 CrossRef CAS PubMed.
- W. Niu, L. Guo, Y. Li, S. Shuang, C. Dong and M. Wong, Anal. Chem., 2017, 89, 11514–11519 CrossRef PubMed.
- B. Zhu, L. Wu, M. Zhang, Y. Wang, C. Liu, Z. Wang, Q. Duan and P. Jia, Biosens. Bioelectron., 2018, 107, 218–223 CrossRef CAS PubMed.
- Y. Gao, T. Ma, Z. Ou, W. Cai, G. Yang, Y. Li, M. Xu and Q. Li, Talanta, 2018, 178, 663–669 CrossRef CAS PubMed.
- B. Zhu, W. Wang, L. Liu, H. Jiang, B. Du and Q. Wei, Sens. Actuators, B, 2014, 191, 605–611 CrossRef CAS.
- M. Jonaghani and H. Zali-Boeini, Spectrochim. Acta, Part A, 2017, 178, 66–70 CrossRef PubMed.
- Y. Ge, A. Liu, R. Ji, S. Shen and X. Cao, Sens. Actuators, B, 2017, 251, 410–415 CrossRef CAS.
- K. Tayade, B. Bondhopadhyay, A. Basu, G. Chaitanya, S. Sahoo, N. Singh, S. Attarde and A. Kuwar, Talanta, 2014, 122, 16–22 CrossRef CAS PubMed.
- J. Xu, Z. Xu, Z. Wang, C. Liu, B. Zhu, X. Wang, K. Wang, J. Wang and G. Sang, Luminescence, 2017, 33, 219–224 CrossRef PubMed.
- Q. Duan, H. Zhu, C. Liu, R. Yuan, Z. Fang, Z. Wang, P. Jia, Z. Li, W. Sheng and B. Zhu, Analyst, 2019, 144, 1426–1432 RSC.
- Q. Duan, X. Lv, C. Liu, Z. Geng, F. Zhang, W. Sheng, Z. Wang, P. Jia, Z. Li, H. Zhu and B. Zhu, Ind. Eng. Chem. Res., 2019, 58, 11–17 CrossRef CAS.
- B. Zhu, L. Wu, M. Zhang, Y. Wang, Z. Zhao, Z. Wang, Q. Duan, P. Jia and C. Liu, Sens. Actuators, B, 2018, 263, 103–108 CrossRef CAS.
- Q. Duan, P. Jia, Z. Zhuang, C. Liu, X. Zhang, Z. Wang, W. Sheng, Z. Li, H. Zhu, B. Zhu and X. Zhang, Anal. Chem., 2019, 91, 2163–2168 CrossRef CAS PubMed.
- P. Jia, Z. Zhuang, C. Liu, Z. Wang, Q. Duan, Z. Liu, H. Zhu, B. Du, B. Zhu, W. Sheng and B. Kang, Anal. Chim. Acta, 2019, 1049, 219–225 CrossRef PubMed.
|
This journal is © The Royal Society of Chemistry 2019 |
Click here to see how this site uses Cookies. View our privacy policy here.