DOI:
10.1039/C9CE01411J
(Paper)
CrystEngComm, 2020,
22, 95-104
Influence of counterions on the supramolecular frameworks of isoquinoline-based silver(I) complexes†
Received
6th September 2019
, Accepted 22nd October 2019
First published on 29th October 2019
Abstract
Silver coordination compounds have been widely studied, among others, because of their fascinating structures where the counterions can play an essential role in the construction of supramolecular networks. Herein, seven new Ag(I) complexes containing bis(1-isoquinolinecarboxamide)ethane (L1), bis(1-isoquinoline-N,N′-dimethylcarboxamide)ethane (L2) and bis(1-isoquinolinecarboxamide)propane (L3) as ligands have been synthesized and their crystal structures were obtained by single crystal X-ray diffraction, with ClO4−, NO3− and CF3CO2− as counterions. These species were analysed from three points of view: first, the differences and similarities among the amide-to-amide hydrogen bond patterns when compared to those of the bis(1-isoquinolinecarboxamide)alkane ligands; second, their structural patterns based on the coordinative ability of the counterions; finally, the analysis of the robustness of the amide-to amide intermolecular hydrogen bonds when a coordination complex is formed. Among the most critical features of anions, it is their coordinating ability and participation in non-covalent interactions with the ligands that are contextualized in this research.
Introduction
Counterions play an important role in a large number of areas, such as organocatalysis modulation,1,2 chemical reactions' energy profiles,3,4 metal cluster aromaticity,5 spectroscopic properties of organic compounds,6 surfactant micellization,7 membrane permeation,8 gelation,9 supramolecular assemblies,10–12 crystal structures,13 and chromatographic separation.14 It has also been recently demonstrated that changes in the counterion can have a great effect on the solid-state structures of a series of compounds, often with surprising results.15–17 On the other hand, N-donor heterocyclic compounds have been demonstrated to have an important contribution in building supramolecular architectures, where studies have been focused on evaluating the role of hydrogen bonding,18 π–π stacking,19 and C–H⋯π contacts20 in the presence of several functional groups, in particular, amide functional groups. Bispyridine21 and bisquinoline22 moieties have been widely studied as groups able to interfere with the amide-to-amide hydrogen bonds. Considering that in many cases the N-donor atom of the isoquinoline ring is not involved in intermolecular interaction22,23 and the silver(I) ion has a linear coordination geometry, this could act as a linker between the N-donor heteroatoms to produce structures where a coordinative bond may coexist with the amide-to-amide synthon, i.e., producing 2D coordination nets by coordinative linking of β-sheets or 3D systems by connecting (4,4)-nets.24 Recently, we have explored the crystal structure of a homologous series of bis(1-isoquinolinecarboxamide)alkane25 derivatives and their perchlorate silver(I) coordination compounds, where the Ag–amide O coordinative bond replaces the amide-to-amide hydrogen bond in almost the entire series as the main interaction in the self-assembly process. Furthermore, the perchlorate ions support the amide-to-amide interference, forming H-bonding with the NH moieties. In order to analyse comprehensively the influence of counterions on the formation of amide-to-amide hydrogen bonds, herein, we report the self-assembly and resulting structures of seven silver(I) complexes of bis(1-isoquinolinecarboxamide)alkanes using nitrate (NO3−) and trifluoroacetate (CF3CO2−) counterions which were compared with those of perchlorate (ClO4−) previously reported (Scheme 1).25 Additionally, a methyl derivative of the carboxamide ligand L1 was synthesized (L2). This ligand cannot establish amide-to-amide interactions and therefore, we can elucidate the importance of the N–H hydrogen bond donor in the supramolecular arrangements of its silver(I) complexes.
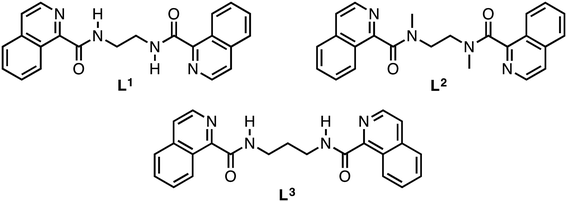 |
| Scheme 1 Schematic representation of bis(1-isoquinolinecarboxamide)ethane (L1), bis(1-isoquinoline-N,N′-dimethylcarboxamide)ethane (L2) and bis(1-isoquinolinecarboxamide)propane (L3). | |
Experimental
General
The full synthesis of the ligands 1,2-bis(1-isoquinolinecarboxamide)ethane (L1) and 1,3-bis(1-isoquinolinecarboxamide)propane (L3), and the complexes [Ag(L1)]ClO4 (1) and [Ag(L3)]ClO4 (7) has been already reported.25 The ligand 1,2-bis(1-isoquinoline-N,N′-dimethylcarboxamide)ethane (L2) has been synthesized using the same procedure. 1H NMR spectra were recorded using a Bruker Avance II 600 MHz spectrometer, with CDCl3 as a solvent. Melting points were determined on an Olympus BX41 optical microscope equipped with an RTV QIMAGING MicroPublisher 3.3 camera. The temperature was controlled with a Linkam THMS600 stage. ATR-FTIR spectra were recorded using a Shimadzu IRAffinity 1 spectrophotometer equipped with a Pike Technologies GradiATR on powder samples (450–4000 cm−1). Elemental analyses were carried out on a Fison EA 1108 analyzer.
Synthetic procedures
N,N′-Dimethyl-1,2-bis(1-isoquinolinecarboxamide)ethane (L2)
Yield: 26%. M.P.: 254–256 °C. ATR-FTIR (cm−1): 3054 (Csp2–H); 2983 (Csp3–H); 1632 (C
O amide). 1H NMR (CDCl3) δ (ppm): 8.54 (2H, d, J = 3.6); 8.44 (2H, d, J = 3.6); 8.15 (2H, d, J = 5.6); 8.10 (2H, d, J = 5.6); 7.95 (4H, s); 3.07 (6H, s). Elem. An. C24H44N4O2: calcd. C, 72.34; H, 5.57; N, 14.06. Found. C, 72.31; H, 5.49; N, 14.01.
General procedure for Ag(I) complexes (1–9)
An aqueous solution of 0.010 g (0.05 mmol) AgX (X = ClO4−, NO3−, CF3CO2−) was added dropwise to an ethanol solution of the corresponding ligand L1, L2 or L3 (0.05 mmol) under stirring and heated to 50 °C for 10 min. The resulting mixture was allowed to evaporate at room temperature, without shielding from light.
[Ag(L1)]NO3 (2).
Colorless needle-like crystals were obtained after one week. Yield: 85%. ATR-FTIR (cm−1): 3229 (N–H); 3069 (Csp2–H); 2933 (Csp3–H); 1634 (C
O amide); 1309 (NO3−). Elem. An. C44H36N10O10Ag2: calcd. C, 48.91; H, 3.36; N, 12.96. Found. C, 48.87; H, 3.29; N, 12.02.
[Ag(L1)]CF3CO2 (3).
Colorless needle-like crystals were obtained after one week. Yield: 85%. ATR-FTIR (cm−1): 3290 (N–H); 3058 (Csp2–H); 2927 (Csp3–H); 1660 (C
O amide); 1651 (C
O counterion); 1085 (C–F). Elem. An. C24H18N4O4F3Ag: calcd. C, 48.75; H, 3.07; N, 9.48. Found. C, 48.71; H, 2.99; N, 8.96.
[Ag(L2)]ClO4 (4).
Colorless needle-like crystals were obtained after one week. Yield: 60%. ATR-FTIR (cm−1): 3072 (Csp2–H); 2941 (Csp3–H); 1621 (C
O amide); 1076 (ClO4−). Elem. An. C48H44N8O12Cl2Ag2: calcd. C, 47.59; H, 3.66; N, 9.25. Found. C, 47.60; H, 3.65; N, 9.15.
[Ag(L2)]NO3 (5).
Colorless needle-like crystals were obtained after two weeks. Yield: 50%. ATR-FTIR (cm−1): 3045 (Csp2–H); 2933 (Csp3–H); 1640 (C
O amide); 1310 (NO3−). Elem. An. C24H22N5O5Ag: calcd. C, 50.72; H, 3.90; N, 12.32. Found. C, 50.68; H, 3.88; N, 12.22.
[Ag(L2)H2O]CF3CO2·3H2O (6).
Colorless needle-like crystals were obtained after 10 days. Yield: 85%. ATR-FTIR (cm−1): 3287 (N–H); 3003 (Csp2–H); 2940 (Csp3–H); 1664 (C
O amide); 1620 (C
O counterion); 1092 (C–F). Elem. An. C26H30N4O8F3Ag: calcd. C, 45.17; H, 4.37; N, 8.10. Found. C, 45.06; H, 4.31; N, 7.99.
[Ag(L3)H2O]n·nNO3 (8).
Colorless needle-like crystals were obtained after 14 days. Yield: 90%. ATR-FTIR (cm−1): 3325 (N–H); 3069 (Csp2–H); 2933 (Csp3–H); 1643 (C
O amide); 1304 (NO3−). Elem. An. C23H22N5O6Ag: calcd C, 48.27; H, 3.87; N, 12.24. Found. C, 48.21; H, 3.85; N, 12.11.
[Ag(L3)]2n·2nCF3O2·nH2O (9).
Colorless needle-like crystals were obtained after 8 days. Yield: 90%. ATR-FTIR (cm−1): 3277 (N–H); 3069 (Csp2–H); 2939 (Csp3–H); 1661 (C
O amide); 1617 (C
O counterion); 1085 (C–F). Elem. An. C50H42N8O9F6Ag2: calcd. C, 48.88; H, 3.45; N, 9.12. Found. C, 48.85; H, 3.41; N, 9.00.
Crystallography
The X-ray diffraction experiments for 1–9 were performed on an Agilent Supernova diffractometer with Cu radiation (λ = 1.5418 Å) at 293 K (except for 2 that was measured at 150 K). Data were indexed, integrated and scaled with the CrysAlisPRO program.26 The crystal structures were solved by direct methods and refined with the full-matrix least-squares technique on F2 by using the SHELXS-2013 and SHELXL-2013 programs included in the WINGX software package.27 C- and N-bound H atoms were placed in their expected positions and refined as riding with Uiso(H) = 1.5Ueq(C) for methyl H atoms and 1.2Ueq(N,C) otherwise. In the structures of 6 and 8, the H atoms of the water molecules were found from Fourier differences and refined as riding with Uiso(H) = 1.5Ueq(O), and the O–H bond distance was restrained to 0.85 Å with DFIX. The crystal used in the data collection of 2 developed a twin during the measurement, causing bad equivalents to arise in the process, and we cannot eliminate all the runs with this issue to keep the completeness as high as possible. Even though the completeness of the final data collection is below the standard and there are a number of reflections missing in the whole theta range, the model proposed fits well the reflections collected, having a final R-value of 0.0459. Two positions were found for the ethylene bridge in 2, the s.o.f.'s were allowed to refine and the N–C and C–C bond distances were restrained. The trifluoroacetate anions in 3, 6 and 9 were disordered into three (3) and two positions (6 and 9). The s.o.f.'s were allowed to refine and the C–C, C–O and C–F bond distances were restrained to 1.50(2), 1.25(2) and 1.32(2) Å, respectively. A SADI soft restraint was included to keep all the F⋯F separations equal. Equal anisotropic displacement parameters were assigned to the C and O atoms of the three disordered CF3COO groups in 3 and 9. The perchlorate anion in 4 is disordered and three positions were found. The s.o.f. was allowed to refine and DFIX restraints were applied to the Cl–O bond lengths [1.42(2) Å] and O⋯O separations [2.32(2) Å]. Also, a rigid body restraint was applied to the Cl and O atoms. A summary of the crystallographic data and structure refinement is given in Table 1. The final geometrical calculations and the graphical manipulation were carried out with the DIAMOND28 and MERCURY29 programs, respectively. CCDC numbers 1869695 (2), 1869696 (3), 1869697–1869699 (4–6), 1869670 (8) and 1869671 (9) contain the supplementary crystallographic data for this paper.
Table 1 Crystallographic data for compounds 2–6, 8 and 9
Crystal data |
2
|
3
|
4
|
5
|
6
|
8
|
9
|
Chemical formula |
C44H36N10O10Ag2 |
C24H18N4O4F3Ag |
C48H44N8O12Cl2Ag2 |
C24H22N5O5Ag |
C26H30N4O8F3Ag |
C23H22N5O6Ag |
C50H42N8O9F6Ag2 |
M
r
|
1080.57 |
591.29 |
1211.55 |
568.33 |
691.41 |
572.32 |
1228.64 |
Crystal system, space group |
Monoclinic, C2/c |
Monoclinic, C2/c |
Triclinic, P![[1 with combining macron]](https://www.rsc.org/images/entities/char_0031_0304.gif) |
Monoclinic, P21/n |
Triclinic, P![[1 with combining macron]](https://www.rsc.org/images/entities/char_0031_0304.gif) |
Triclinic, P![[1 with combining macron]](https://www.rsc.org/images/entities/char_0031_0304.gif) |
Orthorhombic, Pbcn |
a (Å) |
20.536(6) |
22.6683(6) |
9.2321(3) |
7.2584(2) |
8.7388(3) |
7.3244(6) |
37.8693(14) |
b (Å) |
12.539(2) |
11.9842(2) |
11.4380(4) |
14.4126(3) |
11.7437(4) |
8.2198(7) |
7.4572(4) |
c (Å) |
7.7893(14) |
17.9923(5) |
11.8530(4) |
21.3749(5) |
15.1730(4) |
19.5570(10) |
17.8043(8) |
α (°) |
90 |
90 |
96.574(3) |
90 |
89.580(3) |
87.931(5) |
90 |
β (°) |
91.11(2) |
111.403(3) |
91.848(2) |
91.472(2) |
74.494(3) |
86.794(6) |
90 |
γ (°) |
90 |
90 |
104.559(3) |
90 |
80.378(3) |
88.681(7) |
90 |
V (Å3) |
2005.3(8) |
4550.7(2) |
1201.05(7) |
2235.34(9) |
1478.21(8) |
1174.56(15) |
5027.9(4) |
Z
|
2 |
8 |
1 |
4 |
2 |
2 |
4 |
μ (mm−1) |
8.492 |
7.695 |
8.181 |
7.651 |
6.112 |
7.317 |
7.003 |
ρ
cal (g cm−3) |
1.790 |
1.726 |
1.675 |
1.689 |
1.553 |
1.618 |
1.620 |
T (K) |
150.0(1) |
293(2) |
293(2) |
293(2) |
293(2) |
293(2) |
293(2) |
λ (Å) |
1.54184 |
1.54184 |
1.54184 |
1.54184 |
1.54184 |
1.54184 |
1.54184 |
Crystal size (mm) |
0.98 × 0.05 × 0.04 |
0.64 × 0.12 × 0.09 |
0.19 × 0.14 × 0.07 |
0.25 × 0.05 × 0.04 |
0.29 × 0.18 × 0.18 |
0.64 × 0.07 × 0.02 |
0.17 × 0.07 × 0.03 |
Data collection |
Measured reflections |
3391 |
18 357 |
11 671 |
7502 |
37 075 |
7581 |
12 025 |
Independent reflections |
1551 |
4517 |
4444 |
3908 |
5893 |
4340 |
4705 |
Obs. [I > 2σ(I)] reflections |
1471 |
4248 |
4127 |
3355 |
4702 |
3533 |
3303 |
R
int
|
0.0409 |
0.0221 |
0.0197 |
0.0209 |
0.0790 |
0.0744 |
0.0371 |
Refinement |
R[F2 > 2σ(F2)], wR(F2), S |
0.046, 0.123, 1.055 |
0.024, 0.064, 1.027 |
0.024, 0.066, 1.023 |
0.031, 0.081, 1.033 |
0.049, 0.149, 1.048 |
0.063, 0.182, 1.043 |
0.045, 0.129, 1.044 |
Reflections |
1551 |
4517 |
4444 |
3908 |
5893 |
4340 |
4705 |
Parameters |
155 |
412 |
420 |
318 |
469 |
322 |
432 |
Restraints |
3 |
91 |
145 |
0 |
91 |
3 |
184 |
Δρmax, Δρmin (e Å−3) |
0.68, −1.10 |
0.21, −0.74 |
0.24, −0.46 |
0.52, −0.37 |
0.57, −0.72 |
1.34, −1.53 |
0.84, −0.42 |
Results and discussion
Description of the structures
[Ag(L1)]NO3 (2).
Compound 2 consists of molecular entities of Ag(L1) and nitrate counterions, where the Ag1, N3 and O2 atoms are located on a two-fold symmetry axis (4e position). The bis(isoquinoline) ligand in the trans-conformation (apolar disposition of the two amide groups) coordinates to the silver ion through the two nitrogen atoms of the isoquinoline moiety in a crab-like conformation (Fig. 1a). The isoquinoline groups rotate (the dihedral angle between the isoquinoline rings is 36.1° for 2), accommodating the silver ion in the middle of two isoquinoline N-atoms [N2–Ag1–N2a, (a) = −x + 1, y, −z + 1/2] with an angle of 177.9(2)° and a N2–Ag1 bond distance of 2.152(5) Å (Table S1†). The nitrate ion is weakly bound to the silver ion with an O3–Ag1 bond distance of 2.976(5) Å. These [Ag(1)NO3] mononuclear entities are stacked along the c axis, linking the amide NH groups to the nitrate ion of the adjacent molecules [N1–H1⋯O3b donor⋯acceptor separation and DH⋯A angle being 2.929(8) Å and 169°, respectively, (b) = x, −y + 1, z + 1/2] (Table 2 and Fig. 2). These supramolecular chains are connected to their four close neighbours through hydrogen bonds between the isoquinoline CH groups as donors and amide oxygen atoms as acceptors [C7–H7⋯O1c; D⋯A separation of 3.337(7) Å and D–H⋯A angle of 152°; (c) = −x + 3/2, y − 1/2, −z + 1/2] (Fig. S1†). The shortest separation among silver ions is 4.1053(7) Å [Ag1⋯Ag1d; (d) = −x + 1, −y + 1, −z + 1], out of the range for argentophilic interactions.
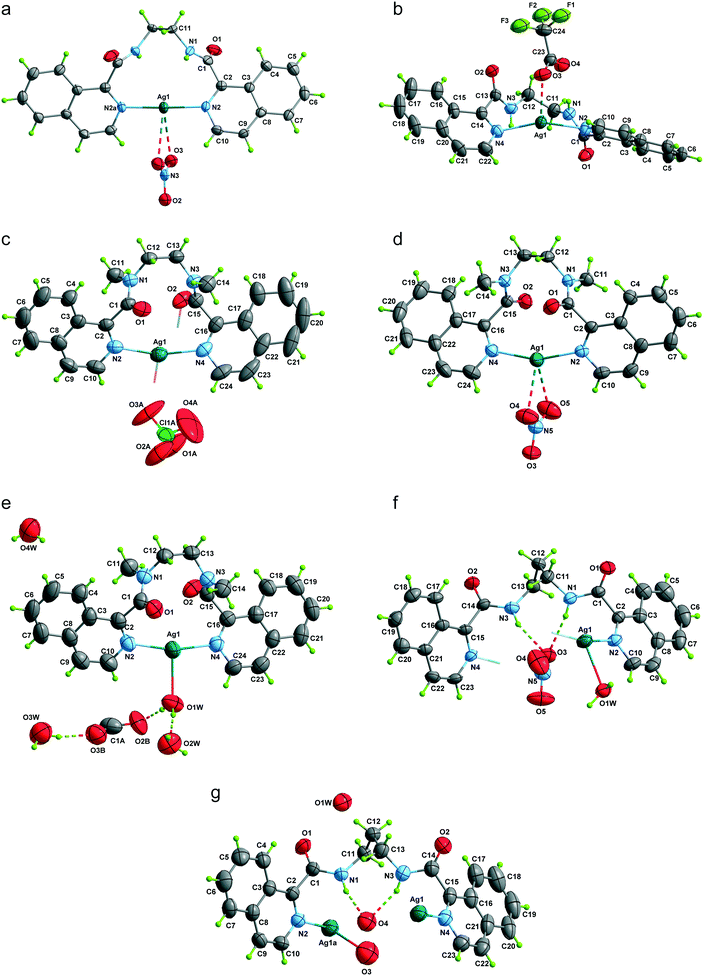 |
| Fig. 1 Views of the molecular structures of 2 (a) [(a) = −x + 1, y, −z + 1/2], 3 (b), 4 (c), 5 (d), 6 (e), 8 (f) and 9 (g) with the numbering scheme. Atomic displacement ellipsoids are drawn at the 50% probability level (the numbered fragment corresponds to the asymmetric unit and, for 6 and 9, the trifluoroacetate counterions have been omitted for clarity). | |
Table 2 Geometrical parameters of the relevant hydrogen bonds in compounds 2–6, 8 and 9
Compounds |
Type |
H⋯A (Å) |
D⋯A (Å) |
D–H⋯A (°) |
Symmetry codes for 2, (b) = x, −y + 1, z + 1/2; (c) = −x + 3/2, y − 1/2, −z + 1/2. 3: (b) = −x + 1, y, −z + 1/2. 4: (b) = −x + 2, −y + 1, −z + 2. 5: (a) = −x + 1/2, y − 1/2, −z + 3/2; (b) = −x + 1, −y + 1, −z + 1. 6: (a) = −x + 1, −y + 1, −z; (b) = x + 1, y, z; (c) = x, y + 1, z; (d) = −x, −y + 1, −z. 8: (a) = x + 1, y, z; (b) = x, y − 1, z. 9: (a) = x, y − 1, z.
|
2
|
N1–H1⋯O3b |
2.08 |
2.929(8) |
169 |
C7–H7⋯O1c |
2.49 |
3.337(7) |
152 |
3
|
N1–H1⋯O4 |
1.96 |
2.784(8) |
161 |
N3–H3⋯O1b |
2.05 |
2.867(2) |
157 |
4
|
C14–H14A⋯O1b |
2.29 |
3.202(3) |
157 |
5
|
C5–H5⋯O1a |
2.61 |
3.464(4) |
154 |
C9–H9⋯O2b |
2.39 |
3.308(4) |
170 |
6
|
O1W–H1WB⋯O2A |
1.87(7) |
2.70(3) |
171(8) |
O1W–H1WA⋯O2W |
1.96(6) |
2.783(6) |
170(8) |
O2W–H2WA⋯O1a |
1.98(6) |
2.814(6) |
169(7) |
O2W–H2WB⋯O3Wb |
1.89(7) |
2.697(9) |
161(9) |
O3W–H3WB⋯O1A |
2.21(9) |
2.99(3) |
151(8) |
O3W–H3WA⋯O4Wc |
1.94(8) |
2.732(8) |
154(9) |
O4W–H4WA⋯O1Ad |
2.18(10) |
2.80(2) |
130(9) |
O4W–H4WB⋯O2Wd |
2.05(7) |
2.868(8) |
162(8) |
8
|
O1W–H1W⋯O5a |
1.87(4) |
2.821(9) |
173(13) |
O1W–H2W⋯O1b |
1.94(8) |
2.834(7) |
155(9) |
N1–H1⋯O3 |
2.20 |
3.040(8) |
165 |
N3–H3⋯O3 |
2.07 |
2.889(8) |
158 |
9
|
O1W⋯O1a |
— |
2.829(8) |
— |
N1–H1⋯O4C |
1.86 |
2.703(14) |
166 |
N3–H3⋯O4C |
2.13 |
2.936(14) |
156 |
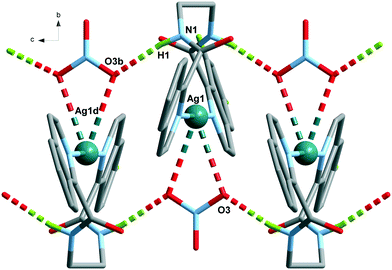 |
| Fig. 2 A view of mononuclear [Ag(L1)NO3] (2) entities stacked along the c axis through N–H(amide)⋯O(nitrate) interactions [(b) = x, −y + 1, z + 1/2; (d) = −x + 1, −y + 1, −z + 1] (all hydrogen atoms have been omitted for clarity, except those involved in intermolecular interactions). | |
[Ag(L1)]CF3CO2 (3).
The crystal structure of compound 3 consists of centrosymmetric dinuclear [Ag(L1)CF3O2]2 units which are linked by amide-to-amide hydrogen bonds along the c axis to form supramolecular chains. In this case, as that occurring in 2, the bis(isoquinoline) ligand in the trans-conformation coordinates one silver(I) ion with the isoquinoline nitrogen atoms in a crab-like conformation [N2–Ag1–N4 angle being 158.15(12)°, and N2–Ag1 and N4–Ag1 bond distances are 2.244(2) and 2.247(2) Å, respectively] (Fig. 1b). The four-coordination around the Ag1 cation is completed with two oxygen atoms from two trifluoroacetate counterions, with one Ag–O bond longer than the other [O3–Ag1 and O3a–Ag1 being 2.560(16) and 2.721(2) Å; (a) = −x + 1, −y + 1, −z + 1] to form a seesaw environment (parameter = 0.72).30 This situation leads to a Ag⋯Ag separation within the dinuclear unit of 4.1802(3) Å. One of the amide NH donor groups links the dinuclear entities to form supramolecular chains running along the c axis [N3–H3⋯O1b; D⋯A is 2.867(2) Å and D–H⋯A is 157°; (b) = −x + 1, y, −z + 1/2]; the other, however, establishes an intradinuclear H-bond with the uncoordinated oxygen atom of the CF3CO2 anion [N1–H1⋯O4; D⋯A and D–H⋯A being 2.784(8) Å and 161°, respectively] (Table 2 and Fig. 3). In the crystal packing, a sandwiched feature along the a axis is observed with a layer of isoquinoline groups separating the more hydrophilic silver(I) environments and the CF3O2 anions. These supramolecular chains are connected to their four close neighbours, as what occurs in the 2 chains, through π⋯π stacking interactions among the isoquinoline rings (see Table S2 and Fig. S2†).
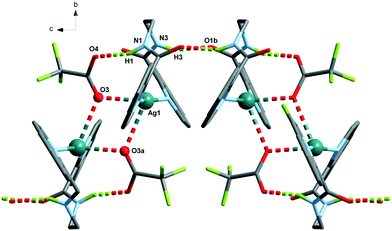 |
| Fig. 3 A view of dinuclear [Ag(L1)CF3CO2]2 (3) entities forming supramolecular chains along the c axis through amide-to-amide interactions [(a) = −x + 1, −y + 1, −z + 1; (b) = −x + 1, y, −z + 1/2] (all hydrogen atoms have been omitted for clarity, except those involved in intermolecular interactions). | |
[Ag(L2)]ClO4 (4).
Compound 4 is formed by centrosymmetric dinuclear Ag2(L2)2 molecular entities and perchlorate anions. The bis(isoquinoline) ligand adopts a trans-conformation as in the 1–3 series, and the aromatic rings rotate to allow their nitrogen atoms to coordinate the Ag(I) ion in a crab-like fashion [N2–Ag1–N4 angle being 156.74(7)° and the N2–Ag1 and N4–Ag1 bond distances are 2.196(2) and 2.192(2) Å, respectively] (Fig. 1c). The three-coordinate T-like environment of the silver(I) ion is completed with an amide oxygen atom from the adjacent L2 ligand [Ag1–O2a bond distance is 2.5126(16) Å; (a) = −x + 1, −y + 1, −z + 2]. Within the dinuclear unit, the Ag⋯Ag separation is 3.4854(4) Å, which is close to the sum of van der Waals radii for two silver(I) ions indicating that argentophilic interactions may drive the formation of these dinuclear systems. The dinuclear entities are connected to their neighbours along the a axis through C14–H14A⋯O1b [(b) = −x + 2, −y + 1, −z + 2] interactions involving the methyl groups and the uncoordinated amide oxygen atoms (Table 2 and Fig. 4). These supramolecular chains are further connected to the adjacent ones by means of π–π stacking interactions (Cg⋯Cg [−x + 1, −y, −z + 1]) among the isoquinoline groups (see Fig. S3†), with the perchlorate anions occupying interchain voids.
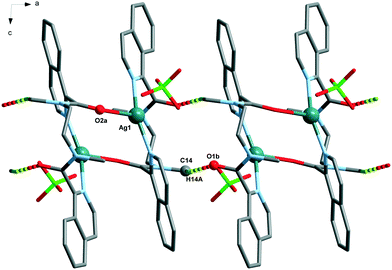 |
| Fig. 4 T-like environment of Ag(I) ions, forming dinuclear [Ag(L2)ClO4]2 (4) entities that are connected through C–H⋯O(amide) supramolecular interactions along the a axis [(a) = −x + 1, −y + 1, −z + 2; (b) = −x + 2, −y + 1, −z + 2] (all hydrogen atoms have been omitted for clarity, except those involved in intermolecular interactions). | |
[Ag(L2)]NO3 (5).
The crystal structure of compound 5 is similar to that of 2. The bis(isoquinoline) ligand in the trans-conformation coordinates one silver(I) ion like a crab [N2–Ag1–N4 angle is 158.61(8)° and the bond distances N2–Ag1 and N4–Ag1 are 2.169(2) and 2.187(2) Å, respectively] leading to an almost linear two-coordinate silver(I) ion (Table S1†). The nitrate anion is located close to the silver(I) ion as in 2, being weakly bound to the Ag(I) ion [O4–Ag1 and O5–Ag1 separations are 2.696(3) and 2.677(3) Å, respectively] (Fig. 1d). Aromatic C–H⋯O(amide) hydrogen bond interactions link these mononuclear entities with the adjacent ones to build supramolecular layers in the [101] plane [C5–H5⋯O1a; D⋯A being 3.464(4) Å and D–H⋯A angle of 154°, and C9–H9⋯O2b; D⋯A being 3.308(4) Å and D–H⋯A angle of 170°; (a) = −x + 1/2, y − 1/2, −z + 3/2, (b) = −x + 1, −y + 1, −z + 1]. The shortest Ag⋯Ag separation occurs within this supramolecular layer being 6.5702(5) Å (Fig. 5).
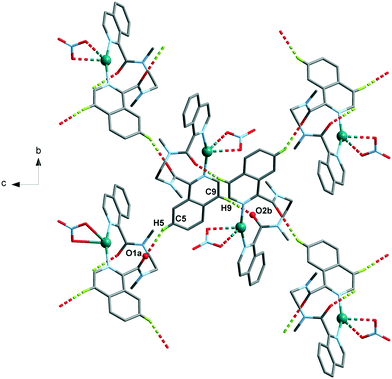 |
| Fig. 5 A view of a supramolecular layer growing in the [101] direction built from dinuclear [Ag(L2)NO3]2 (5) entities connected to four others by C–H⋯O(amide) supramolecular interactions [(a) = −x + 1/2, y − 1/2, −z + 3/2, (b) = −x + 1, −y + 1, −z + 1] (all hydrogen atoms have been omitted for clarity, except those involved in intermolecular interactions). | |
[Ag(L2)H2O]CF3CO2·3H2O (6).
The crystal structure of compound 6 consists of mononuclear silver(I) complexes, trifluoroacetate counterions and water molecules (Fig. 1e). As that occurring in previous compounds, the bis(isoquinoline) ligand in the trans-conformation coordinates the silver ion by the two isoquinoline nitrogen atoms [N2–Ag1–N4 angle being 164.11(13)° and the N2–Ag1 and N4–Ag1 bond distances are 2.156(4) and 2.154(3) Å, respectively]. The three-coordinate Ag(I) ion T-geometry is filled by a water molecule [O1W–Ag1 bond distance being 2.533(4) Å]. The amide oxygen atoms act as acceptors to form a chain running through the b axis (Fig. 6a). A complex network of hydrogen bonds involving the water molecules as donors and the CF3O2 anions connects the chains forming supramolecular layers in the ab plane (Fig. 6b), which are then further linked through π–π interactions involving the isoquinoline groups of the ligand. Interestingly, a centrosymmetric water hexamer is formed by the crystallization water molecules (Fig. 6b, insert) with a chair conformation.31 The shortest silver⋯silver separation occurs between molecules of the same layer, being 7.5376(5) Å.
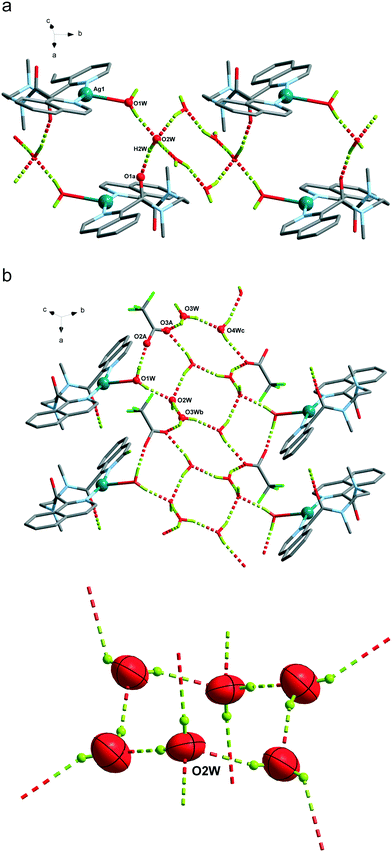 |
| Fig. 6 (a) Supramolecular chain growing along the b axis in 6 (trifluoroacetate counterions have been omitted for clarity). (b) Hydrogen bonding network that connects the chains forming layers at the ab-plane (insert: centrosymmetric water hexamer, atomic displacement ellipsoids are drawn at the 50% probability level) [(a) = −x + 1, −y + 1, −z; (b) = x + 1, y, z; (c) = x, y + 1, z] (all hydrogen atoms have been omitted for clarity, except those involved in intermolecular interactions and only one location of the disordered trifluoroacetate anion is presented). | |
[Ag(L3)H2O]n·nNO3 (8).
The crystal structure of 8 consists of bis(isoquinoline) coordinated silver(I) chains running along the a axis and nitrate counterions and it is isomorphous with 7. The silver(I) ion is three-coordinated in a T geometry where two isoquinoline nitrogen atoms from two different ligands occupy the trans-positions [N2–Ag1 and N4a–Ag1 bond distances being 2.227(5) and 2.223(4) Å and N2–Ag1–N4a angle being 167.47(18)°; (a) = x + 1, y, z], while the cis-position is occupied by a water molecule [Ag1–O1W bond distance is 2.580(5) Å]. In this case, the bis(isoquinoline) group adopts a cis-conformation, i.e. the two amide NH groups pointing to the same side of the aliphatic chain (Fig. 1f). However, the two isoquinoline groups are not coplanar to form a ring when the Ag(I) ion is coordinated, as that occurring in the L1 and L2 ligand series of complexes, but they twist leading to a coil when the silver ion is coordinated. The amide oxygen atoms act as acceptors of hydrogen bonds involving the water molecule and the aromatic C(23)–H group, and these interactions connect the chains along the b direction (Fig. 7). Due to the presence of an inversion center, chains of opposite handedness alternate in the c direction. The chains are also linked to those of opposite handedness through π–π interactions [shortest centroid⋯centroid separation of 3.628(6) Å and offset angle of 20.9(7)°] (Fig. S4†). The amide NH groups and O1W establish H-bonds with the nitrate anion (see Table 2 and Fig. 7). The separation of the silver ions within each chain is 7.3244(9) Å, which is shorter than the interchain Ag⋯Ag separation [8.9797(7) Å].
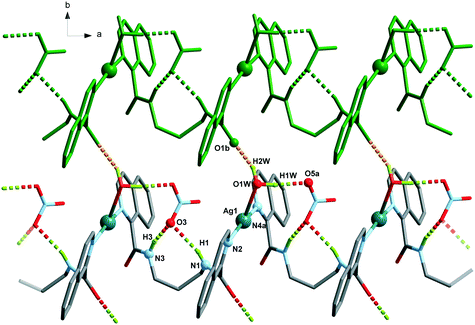 |
| Fig. 7 A view of the silver coordination chain of 8 running along the a axis. The water molecules that complete the T-like environment of Ag(I) ions connect each chain through O–H⋯O(amide) interactions along the b axis [(a) = x + 1, y, z; (b) = x, y − 1, z] (all hydrogen atoms have been omitted for clarity, except those involved in intermolecular interactions). | |
[Ag(L3)]2n·2nCF3O2·nH2O (9).
Although 9 is not isomorphous with 8 and 7, its crystal structure is very similar with chains of bis(isoquinoline) bridged silver(I) ions running along the b axis, trifluoroacetate anions and water molecules. The ligand L3 in 9 adopts the cis-conformation with the two amide NH groups pointing to the same side of the aliphatic chain (Fig. 1g). As what occurs in 7 and 8, the twist of the ligand generates a chiral coil-like chain, with the two handedness present in the unit cell due to an inversion center. The silver(I) ion is three-coordinated with a T-geometry where two isoquinoline nitrogen atoms from two L3 ligands bond the silver ion in the trans-position [Ag1–N2a and Ag1–N4 distances being 2.178(3) and 2.165(4) Å, respectively; (a) = x, y − 1, z] while the cis-position is occupied by a trifluoroacetate molecule [Ag1–O3a bond distance being 2.574(12)]. The hydrogen bonds involving the water molecule as a donor and the amide oxygen atom [O1] as an acceptor connect two chains of opposite directions (Fig. 8), and these two-chain structures are further linked through π–π interactions among the isoquinoline groups giving rise to the supramolecular packing (see Fig. S5 and Table S2†). The silver⋯silver separation within the chain is 7.4572(9) Å, which is a value shorter than the shortest interchain Ag⋯Ag distance [8.9734(9) Å].
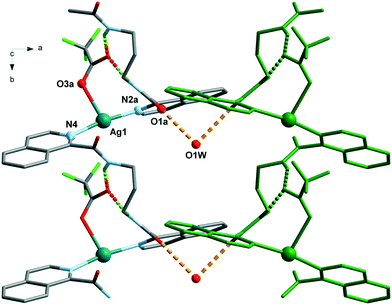 |
| Fig. 8 A view of the Ag(I) coordination chain of 9 growing along the b axis. Two chains are connected through water molecule hydrogen bonding interactions [(a) = x, y − 1, z] (all hydrogen atoms have been omitted for clarity, except those involved in intermolecular interactions. Only one trifluoroacetate position has been presented). | |
Same ligand, varying the counterion
The Ag(L1) series of complexes show little resemblance among them. Compound 1 is formed by a polymeric chain of silver(I) ions linked by ligand L1,25 while in the case of 2, there are mononuclear discrete entities and these discrete units become dinuclear in 3, given that CF3CO2− acts as a bridge. This situation may be explained if we consider the coordination ability of the counterions that runs in the order CF3CO2− > NO3− > ClO4−, with perchlorate being the one that seldom enters in the coordination sphere of the silver(I) complexes. In a CSD32 search of Ag coordination complexes with at least one Ag–N bond, 135, 733, and 270 exhibit a perchlorate, a nitrate or a trifluoroacetate anion coordinated, respectively, while in 1085, 1039 and 170 complexes, the ClO4−, NO3− or CF3CO2− anion remains uncoordinated. In the cases of 2 and 3, supramolecular chains are formed via hydrogen bonds involving the amide group, and these chains in both cases pack in the crystal through interchain π⋯π interactions, which are stronger in 3 (see Table S2†). However, the polymeric chains in 1 interact with their neighbours through H-bond interactions involving the perchlorate anions.
The crystal packing of the Ag(L2) (L2 = methylated L1) series is, again, quite different when varying the counterion, and it is influenced by the methylation of the amide nitrogen atom which now cannot establish H-bond interactions. In this series, both perchlorate (4) and trifluoroacetate (6) do not enter in the coordination sphere of the silver(I) ion and occupy the interchain space, while in 1085 the H-bond network. Among the two series, Ag(L1) and Ag(L2), 6 is the only hydrated compound, where a water molecule occupies a coordination position in the silver(I) environment and even a water hexamer in the chair conformation is also formed.
The Ag(L3) series, where the alkane chain length of the ligand is increased to three carbon atoms (L3), is characterized by the cis arrangement of the amide groups. The cis orientation of the amide group in odd chain lengths is a general trend observed in alkane(bisamides) regardless its coordination to metal ions and the conformational result obtained from the double gauche (synclinal) conformation in the center of the hydrocarbon bridge (see Table S3 and, Fig. S6 and S7†). This situation makes the two isoquinoline groups of each ligand in Ag(L3) do not coordinate the same Ag(I) ion as it occurs in the Ag(L1) and Ag(L2) series, leading to coil-like chains. The three counterions in Ag(L3) act as acceptors of the NH hydrogen bonds, but only in the trifluoroacetate case (9) do they also participate in the coordination sphere of the silver(I) ion. In the three cases [Ag(L3)], the interchain interactions occur through π⋯π stacking among the isoquinoline moieties (Table S2†). Among the three series, Ag(L3) is less influenced by the change in the counterion. This kind of coil-like chains observed in Ag(L3) have been reported previously, despite the presence of other ligands, for bis(pyridinecarboxamide)propane coordinated to transition metal ions,33,34 indicating the robustness of the supramolecular structure.
Same counterion, varying the ligand
The analysis of the structures from the counterion point of view reveals that perchlorate seems to be the more innocent counterion, seldom involved in the coordination, but it does participate in the H-bonding network being the primary acceptor for the NH amide hydrogen bond in all the cases. This situation produces a similar coordination complex in 1 and 4, but in 1, it is extended in one-dimension, and in 4, only one loop of the 1 chain is formed. The coordination network in 7 is influenced by the third carbon atom of the alkane chain and its cis-arrangement, so it has little resemblance to the other ones.
The case of the nitrate anion is intermediate, being located in the neighbourhood of the Ag(I) cation as in 2 and 5, but not too close to be part of its coordination sphere. In the case of 8, the coordination sphere of the Ag(I) ion is filled by a water molecule and nitrate is located in the intermolecular space. The 2 and 5 complexes are similar mononuclear complexes, because the nitrate anion located close to the Ag(I) cation precludes the polymerization. The case of 8, however, is different since the cis-arrangement of the propane bis(carboxamide) moiety forms the helicoidal chains described above. The increased binding ability of CF3COO− is observed in complexes 3 and 9 where it participates in the coordination sphere of the Ag(I) cation. However, in 3, the coordination results in a dinuclear complex, whereas in the case of 9, a chain is formed similarly to those of the Ag(L3) series.
Conclusions
The structural analysis of the seven silver(I) complexes reported here shows that counteranions have a considerable effect on the molecular and supramolecular structures of coordination compounds. The counteranions can exert an influence on the crystal structure depending on their coordination ability and the non-covalent interactions they establish with the ligands. Even when the molecular volume among the ClO4−, NO3− or CF3CO2− anions change significantly (49.6, 34.5 and 72.6 cm3 mol−1, respectively), the steric effect does not play an important role in this series of compounds, as in other silver complexes.35 However, the coordinating ability of the counteranion is important in the construction of crystal structures. For example, trifluoroacetate anions act as μ-oxo bridges between Ag(I) ions in 3 and as monodentate ligands in 9, whereas 6 is the only structure where the competition with water molecules moves it to the background without participation in the coordination sphere of the silver(I) ions. On the other hand, nitrate with an intermediate coordinative ability interacts weakly in a bidentate symmetrical mode (chelating) in 2 and 5, moving to a monodentate mode in 8. Perchlorate anions do not participate in the coordination sphere of the Ag(I) ion, having a poor influence on the supramolecular packing. Finally, the increase of conformational freedom in the aliphatic chain [Ag(L1) to Ag(L3)] has a substantial impact on the increase of the dimensionality of the structure (mono- and dinuclear entities to 1D chains).
Conflicts of interest
There are no conflicts to declare.
Acknowledgements
This research was funded by Project CONICYT/FONDECYT No. 1150747 and CONICYT scholarship No. 21120908, Project VRID No. 219.023.055-INV and ENLACE UdeC 216023053-1. Funding for this work was also provided by the Spanish Ministerio de Ciencia e Innovación through projects MAT2014-57465-R and MAT2017-89207-R.
Notes and references
- S. Gupta, D. Koley, K. Ravikumar and B. Kundu, J. Org. Chem., 2013, 78, 8624–8633 CrossRef CAS.
- S. L. Dabb, J. H. Ho, R. Hodgson, B. A. Messerle and J. Wagler, Dalton Trans., 2009, 634–642 RSC.
- Y. Ren, X. Wang, S. Y. Chu and N. B. Wong, Theor. Chem. Acc., 2008, 119, 407–411 Search PubMed.
- A. Ebrahimi, M. Habibi and A. Amirmijani, J. Mol. Struct.: THEOCHEM, 2007, 809, 115–124 CrossRef CAS.
- A. Chakraborty, S. Giri, S. Duley, A. Anoop, P. Bultinck and P. K. Chattaraj, Phys. Chem. Chem. Phys., 2011, 13, 14865–14878 RSC.
- G. M. A. Junqueira, W. R. Rocha, W. B. De Almeida and H. F. Dos Santos, Phys. Chem. Chem. Phys., 2003, 5, 437–445 RSC.
- B. Naskar, A. Dey and S. P. Moulik, J. Surfactants Deterg., 2013, 16, 785–794 CrossRef CAS.
- S. Kubota, O. Shirai, T. Hibi, Y. Tozawa and K. Kano, Anal. Sci., 2013, 29, 161–164 CrossRef CAS.
- M. C. Morán, T. Alonso, F. S. Lima, M. P. Vinardell, M. G. Miguel and B. Lindman, Soft Matter, 2012, 8, 3200–3211 RSC.
- M. A. Withersby, A. J. Blake, N. R. Champness, P. Hubberstey, W. S. Li and M. Schroder, Angew. Chem., Int. Ed. Engl., 1997, 36, 2327–2329 CrossRef CAS.
- S. Noro, R. Kitaura, M. Kondo, S. Kitagawa, T. Ishii, H. Matsuzaka and M. Yamashita, J. Am. Chem. Soc., 2002, 124, 2568–2583 CrossRef CAS PubMed.
- O. S. Jung, Y. J. Kim, Y. A. Lee, K. M. Parkand and S. S. Lee, Inorg. Chem., 2003, 42, 844–850 CrossRef CAS PubMed.
- Y. Kim, E. J. Mckinley, K. E. Christensen, N. H. Rees and A. L. Thompson, Cryst. Growth Des., 2014, 14, 6294–6301 CrossRef CAS.
- S. P. Porras, M. L. Riekkola and E. Kenndler, Electrophoresis, 2002, 23, 367–374 CrossRef CAS.
- Y. Kang, S. S. Lee, K.-M. Park, S. H. Lee, S. O. Kang and J. Ko, Inorg. Chem., 2001, 40, 7027–7031 CrossRef CAS.
- D. L. Reger, R. F. Semeniuc and M. D. Smith, Inorg. Chem., 2001, 40, 6545–6546 CrossRef CAS.
- P. L. Caradoc-Davies, L. R. Hanton and W. J. Henderson, J. Chem. Soc., Dalton Trans., 2001, 2749–2755 RSC.
- R. Bishop, CrystEngComm, 2015, 17, 7448–7460 RSC.
- C. Janiak, J. Chem. Soc., Dalton Trans., 2000, 3885–3896 RSC.
- M. Nishio, CrystEngComm, 2004, 6, 130–158 RSC.
-
K. Biradha and L. Rajput, Organic Crystal Engineering: Frontiers in Crystal Engineering, Crystal Engineering with the Molecules Containing Amide and Pyridine Functionalities, ed. E. R. T. Tiekink and J. Vittal, John Wiley & Sons, Ltd, Chichester, UK, 2010, ch. 7, pp. 215–238 Search PubMed.
- N. Parra, L. Guarda, J. B. Belmar, P. I. Hidalgo, C. A. Jiménez, J. Pasán and C. Ruiz-Pérez, CrystEngComm, 2013, 15, 7212–7221 RSC.
- H. Xu, R. Wang, C. Fan, G. Liu and S. Pu, Turk. J. Chem., 2016, 40, 38–53 CrossRef CAS.
- S. Muthu, J. H. K. Yip, J. J. Vittal, B. F. Abrahams and B. F. Hoskins, J. Chem. Soc., Dalton Trans., 2001, 3577–3584 RSC.
- N. Parra, J. B. Belmar, C. A. Jiménez, J. Pasán and C. Ruiz-Pérez, CrystEngComm, 2017, 19, 1076–1088 RSC.
-
Agilent, CrysAlis PRO, Agilent Technologies UK Ltd, Yarnton, England, 2011 Search PubMed.
- L. J. Farrugia, J. Appl. Crystallogr., 1999, 32, 837–838 CrossRef CAS.
-
DIAMOND 2.1d, Crystal Impact GbR, CRYSTAL IMPACT, K. Brandenburg & H. Putz GbR, Bonn, Germany, 2000 Search PubMed.
- J. van de, S. C. F. Macrae, P. R. Edgington, P. McCabe, E. Pidcock, G. P. Shield, R. Taylor and M. Towler, J. Appl. Crystallogr., 2006, 39, 453–457 CrossRef.
- L. Yang, D. R. Powell, R. P. Houser, D. S. Cati and H. Stoeckli-Evans, Dalton Trans., 2007, 955–964 RSC.
- L. Infantes and S. Motherwell, CrystEngComm, 2002, 4, 454–461 RSC.
- C. R. Groom, I. J. Bruno, M. P. Lightfoot and S. C. Ward, Acta Crystallogr., Sect. B: Struct. Sci., Cryst. Eng. Mater., 2016, 72, 171–179 CrossRef CAS.
- X. Wang, Z. Chang, H. Lin, A. Tian, G. Liu, J. Zhang and D. Liu, RSC Adv., 2015, 5, 14020–14026 RSC.
- J. Z. Travis, S. R. Pumford, B. L. Martínez and R. L. LaDuca, Polyhedron, 2018, 142, 25–37 CrossRef CAS.
- C.-Y. Niu, B.-L. Wu, X.-F. Zheng, X.-S. Wan, H.-Y. Zhang, Y.-Y. Niu and L.-Y. Meng, CrystEngComm, 2009, 11, 1373–1382 RSC.
|
This journal is © The Royal Society of Chemistry 2020 |
Click here to see how this site uses Cookies. View our privacy policy here.