DOI:
10.1039/C9CE01464K
(Paper)
CrystEngComm, 2020,
22, 85-94
Investigation on temperature-dependent electrical properties of La1−xAxCoO3 (A – La, Li, Mg, Ca, Sr, Ba)†
Received
17th September 2019
, Accepted 19th November 2019
First published on 20th November 2019
Abstract
This study focuses on temperature-dependent electrical property investigation of undoped and defect induced A (La) site-doped LaCoO3 with Li, Mg, Ca, Sr and Ba synthesized by a sol–gel method. X-ray diffraction analysis of the samples revealed a phase pure rhombohedral crystal structure with slight change in unit cell parameters, indicating the successful incorporation of the dopants. HRTEM analysis of the samples revealed the polycrystalline nature of the samples. Warpage-free, well-sintered dense ceramic pellets as revealed by SEM images were further analyzed using impedance spectroscopy. Temperature-dependent electrical studies showed a significant variation in resistance with the increase in temperature in all studied perovskites. The Cole–Cole plot showed a clear contribution of grains and grain boundaries of the materials to the electrical conductivity which was further analyzed using modulus spectroscopy. The Arrhenius plot drawn for all the studied compositions clearly indicated that doping alkali and alkaline earth metals improves the electrical conductivity of LaCoO3. Among the dopants, calcium and barium substitution resulted in maximum enhancement in conductivity in the range of 9 × 10−8–8 × 10−4 S cm−1 from 300 to 750 °C.
1. Introduction
Polycrystalline perovskite oxides have attracted much interest in recent years owing to their outstanding properties. They are represented by the general formula ABO3 with the A cation having a dodecahedral coordination and the B cation having an octahedral coordination with oxygen atoms. When elements with multiple oxidation states are present in the A or B site, they play a significant role in the property enhancement of the material due to the inherent defects that are generated in the crystal. Generally, perovskite materials are widely used in many applications such as supercapacitors, solar cells, gas sensors, solid oxide fuel cells, etc.1–4 LaCoO3 is one such material which has attracted widespread interest due to its thermally induced spin state transitions from low spin to intermediate and high spin states at low temperatures.5,6 Cobalt, a close neighbour of iron in the periodic table, is an element with a partially filled d-orbital due to which it exhibits multiple oxidation states. This feature makes cobalt switch its oxidation states depending on the external conditions such as temperature, pressure, gas atmosphere, etc. This behavior can significantly influence the material properties. The electronic structure and local geometry influence the parameters such as Co–O distances and O–Co–O angles of LaCoO3. The change in the Co–O distance due to thermal displacement is reflected in the shape of the Co 2p state.7,8 It is predictable that mixed electronic and ionic conductors may be formed when the A site atoms are replaced by a suitable class of elements in these perovskites.9 The substitution of elements like Sr for La sites in LaCoO3 perovskite materials leads to a rich point defect chemistry10,11 which promotes oxygen ionic conductivity due to oxygen vacancies and considerable electronic conductivity due to a mixed valence state of the transition metal Co.12,13 Replacement of lanthanum by alkali or alkaline earth elements is chemically feasible.14–18 Particularly, monovalent and divalent elements like lithium, magnesium, calcium, strontium and barium are certainly promising substituents in the position of trivalent La in LaCoO3 to achieve significant enhancement in electronic and ionic transport properties. However, formation of a phase pure material after doping with possible modification in the crystal structure is always challenging.
Impedance spectroscopy, which measures frequency-dependent electrical transport properties, is a powerful technique for investigating the transport mechanisms. Grain and grain boundary effects affect the transport properties in various manners. In addition, temperature also influences the grain and grain boundary properties. To the best knowledge of the authors, there are no literature studies on the electrical properties of LaCoO3 with various A-site dopants and also on the use of modulus spectroscopy for differentiating the grain boundary conduction process from the electrode polarization effect. The present work deals with a systematic exploration of the electrical behavior of LaCoO3 (LCO), La0.8Li0.2CoO3 (LLCO), La0.8Mg0.2CoO3 (LMCO), La0.8Ca0.2CoO3 (LCCO), La0.8Sr0.2CoO3 (LSCO) and La0.8Ba0.2CoO3 (LBCO) in the operating temperature range of 573 K to 1023 K.
2. Experimental work
2.1 Powder synthesis
LaCoO3 was synthesized using lanthanum nitrate (Himedia, La(NO3)3·6H2O) and cobalt nitrate (LobaChemie, Co(NO3)2·6H2O) as the starting precursors by using a sol–gel method. The chemical reaction occurring during the synthesis can be stated as follows: |  | (1) |
Citric acid (complexing agent) and polyethylene glycol (PEG) in a ratio of 1
:
1 were added to the solution containing a stoichiometric amount of the precursors and the resulting mixture was heated at 80 °C accompanied by constant stirring for 8 hours to obtain the gel. Stoichiometric amounts of lithium acetate (LobaChemie, CH3COOLi·2H2O), magnesium nitrate hexahydrate (Himedia, Mg(NO3)2·6H2O), calcium nitrate tetrahydrate (Himedia, Ca(NO3)2·4H2O), and strontium nitrate (Merck, Sr(NO3)2) were carefully weighed and added to separate batches of the above solution as dopants according to the composition La0.8A0.2CoO3 (A – Li, Mg, Ca, Sr, Ba) before heating. The wet gel was dried in an oven at 80 °C for 6 hours to obtain the dried gel. A small amount of this dried gel was crushed and subjected to thermogravimetry/differential scanning calorimetry analysis (TG/DSC) using a NETZSCH STA 449 F3 Jupiter from room temperature to 1200 °C in an ambient atmosphere at a heating rate of 10 °C min−1 for determining the phase formation temperature. Later all dried gels were calcined at 800 °C for 2 hours to obtain phase pure perovskites.
2.2 Crystallographic phase identification
Calcined powders were transformed into the form of cylindrical pellets and subsequently sintered at 1200 °C for 2 hours with a heating ramp of 5 °C min−1. Powders of the synthesized perovskites, after calcination and sintering, were subjected to structural analysis by the X-ray diffraction (XRD) technique on a GE inspection technology X-ray diffraction system 3003 TT instrument at an operating voltage of 40 kV using CuKα radiation of wavelength 1.54 Å with a scan rate of 0.001 degrees per second and a step size of 0.05°. The crystallographic phase of the crushed pellets was analyzed and phase purity was confirmed using this technique. Rietveld analysis was performed by using X'pert Highscore Plus software to set the precise values of the cell parameters as well as the cell volume.
2.3 Microstructure analysis
The morphology and elemental composition of the sintered pellets were analyzed using scanning electron microscopy (SEM, Zeiss EVO 18) at an accelerating voltage of 15 kV. The average grain size of the pellets was calculated from SEM images using ImageJ software (provided in the ESI†). Interfacial studies of the sintered pellets were examined using high resolution transmission electron microscopy (HRTEM) analysis (JEOL JEM 2100) at an accelerating voltage of 200 kV. Chemical analysis was performed by using an OXFORD energy dispersive X-ray spectrometer (EDS). Selected area electron diffraction (SAED) patterns were analyzed using the Digital Micrograph software version 1.85.1535. Raman spectroscopic studies of the samples were performed at room temperature by using a WITec Alpha Control 300R, Germany.
2.4 Electrical transport measurements
The pellets were polished and coated with high temperature silver paste for better ohmic contact, which also acted as electrodes during impedance measurements. The electrical properties of the samples were studied by using a Keysight E4990A impedance analyzer in a frequency range of 20 Hz to 20 MHz from 300 °C to 750 °C.
The complex impedance (Z*) and complex electric modulus (M*) are related to each other as follows:
|  | (3) |
| 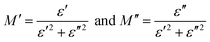 | (4) |
where
Z′,
M′, and
ε′ are the real and
Z′′,
M′′, and
ε′′ are the imaginary parts of the impedance and electric modulus, respectively.
C0 is the geometric capacitance (
C0 =
ε0A/
l),
ω is the angular frequency (
ω = 2π
f),
M∞ is the high frequency limiting modulus,
ε* is the complex dielectric constant and the function
φ(
t) gives the time evolution of the electric field within the material. DC electrical conductivity was calculated by using the resistance values by applying the equation
|  | (5) |
where
R is the bulk resistance in Ohms, and
l and
A are the thickness and area of the pellets in cm and cm
2, respectively. The Arrhenius equation relating the DC electrical conductivity of the material to temperature and activation energy is as follows:
| 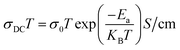 | (6) |
where
σ0 is the pre-exponential factor,
Ea is the activation energy,
KB is the Boltzmann constant and
T is the temperature.
3. Results and discussion
3.1 Crystallographic phase analysis
The X-ray diffractograms taken from powders of sintered LaCoO3 and La0.8A0.2CoO3 (A – Li, Mg, Ca, Sr, Ba) samples are shown in Fig. 1. The peaks can be indexed based on a rhombohedral unit cell with the space group R
c(167) according to PCPDF database number #480123. The data show no evidence of secondary phases. In addition, a slight shift in the peaks corresponding to doped compositions when compared to that of undoped LCO indicates that the dopants are effectively incorporated in the crystal structure.
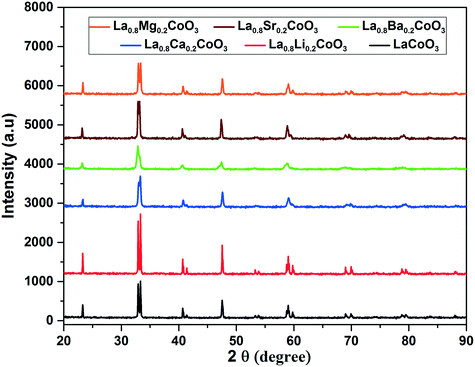 |
| Fig. 1 X-ray diffractograms of doped and undoped LaCoO3. | |
For identifying the influence of the dopants on the crystal structure, refined cell parameters have been obtained by using the Rietveld method (provided in the ESI†) and are listed in Table 1. A typical unit cell of LaCoO3 (rhombohedral R
c(167)) as shown in Fig. 2 was used as a reference for the refinement. Variation in cell dimensions when compared to those of the undoped perovskite is simultaneously reflected in the unit cell volume which is in agreement with the ionic radii of the dopants (given in Table 1). As lithium and magnesium have smaller ionic radii compared to lanthanum, a decrease in unit cell volume is observed for LLCO and LMCO, whereas for LCCO and LSCO, due to the higher ionic radii of the dopants, an increased cell volume is observed. However, variations in the a and c axes of the rhombohedral unit cell indicate the possibility of dopant incorporation along both axes. Variation in cell volume can also be attributed to the oxide ion vacancy and defect formation due to the replacement of the trivalent lanthanum ions with divalent/monovalent dopants.
Table 1 Cell parameters of synthesized La0.8A0.2CoO3 (A = La, Li, Mg, Ca, Sr, Ba)
Compound |
Lattice parameters |
Ionic radii of dopants (pm) |
Cell volume (Å3) |
a = b (Å) |
c (Å) |
LaCoO3 |
5.4400 (1) |
13.1300 (4) |
103 |
336.49 |
La0.8Li0.2CoO3 |
5.4418 (7) |
13.0850 (3) |
74 |
335.57 |
La0.8Mg0.2CoO3 |
5.4320 (1) |
13.1200 (2) |
72 |
335.26 |
La0.8Ca0.2CoO3 |
5.4220 (9) |
13.2300 (4) |
100 |
336.83 |
La0.8Sr0.2CoO3 |
5.4430 (2) |
13.1900 (2) |
118 |
338.41 |
La0.8Ba0.2CoO3 |
5.4440 (9) |
13.0840 (1) |
161 |
335.93 |
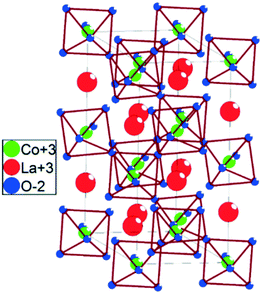 |
| Fig. 2 Idealized structure of LaCoO3. | |
3.2 Microstructural and density analysis
The HRTEM images of the crushed powders of sintered pellets are shown in Fig. 3. The images in Fig. 3a show a flaky morphology with grains and grain boundaries. Lattice fringes in the high resolution images (Fig. 3b) confirm individual grains separated by distinct boundaries with different orientations of the same crystal on both sides.19 Spot patterns observed in all SAED data (Fig. 3c, taken from the large area shown in Fig. 3a) confirm the crystalline nature of the sample. The reflections in the SAED patterns and lattice spacing in the HRTEM images can be indexed/interpreted based on the crystal structure obtained by the XRD analysis.
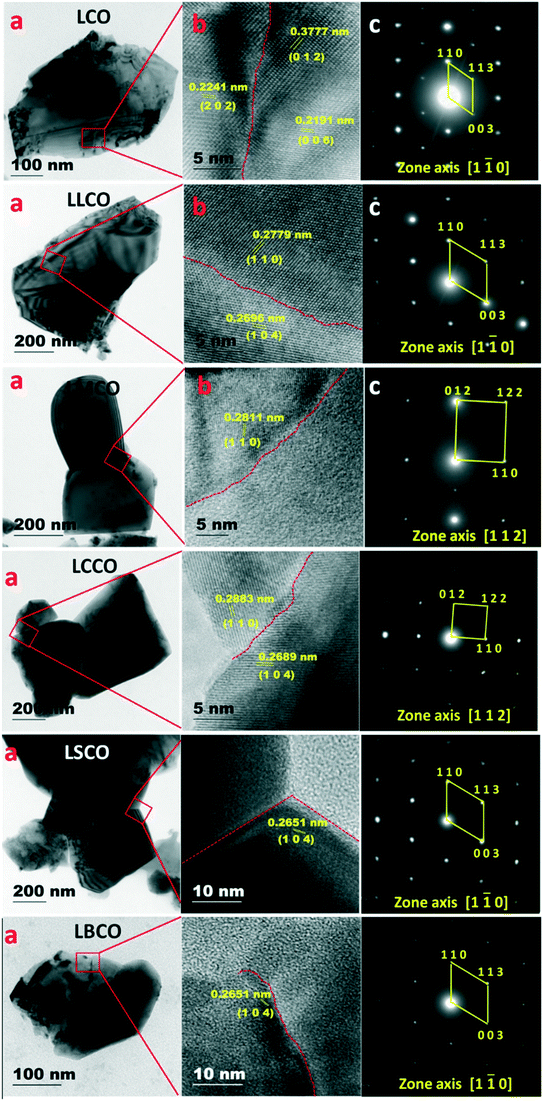 |
| Fig. 3 HRTEM data of compounds: (a) images revealing particle size, (b) high resolution images, and (c) SAED patterns. | |
Investigation of the grain morphology and microstructure of the sintered pellets was conducted using SEM and the results are shown in Fig. 4. Since most of the materials are formed from aggregates of differently oriented crystals or grains, their interface can significantly affect the physical properties in the bulk form. The SEM micrographs taken from the surface of the sintered pellets of undoped and doped lanthanum cobaltites reveal that the particles are well sintered with defined grains and discrete grain boundaries. Larger grain sizes were observed for LSCO, LLCO and LCO, when compared to that for the LBCO sample. The geometric density of each pellet has a good correlation with the relative density (with respect to water) as shown in Table 2. The pellets of doped compositions exhibited higher densities when compared to those of the undoped composition LCO. The highest density was observed for the LCCO, LBCO and LLCO samples possibly due to their grain growth which appreciably reduced the pores forming dense ceramics.
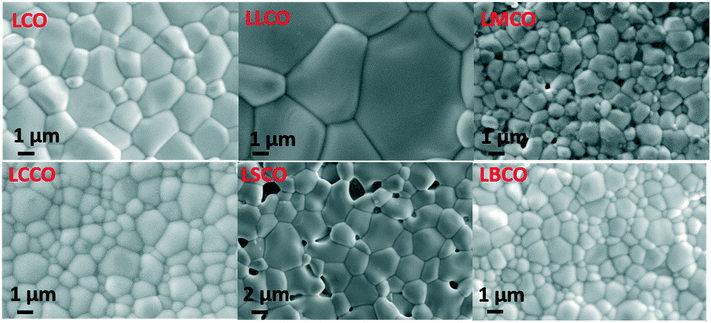 |
| Fig. 4 Scanning electron micrographs of sintered pellets. | |
Table 2 Grain size and density measurements of the sintered pellets
Compound |
Average grain size (nm) |
Geometric density (g cm−3) |
Relative density |
LCO |
2376 |
5.6024 |
96.3 |
LLCO |
2750 |
6.0883 |
98.1 |
LMCO |
1125 |
6.0747 |
96.6 |
LCCO |
1217 |
6.1449 |
98.5 |
LSCO |
3741 |
6.0565 |
96.1 |
LBCO |
971 |
6.0947 |
97.8 |
3.3 Raman spectroscopy analysis
Raman spectroscopy analysis was performed to study the dynamic local distortions and vibrational mode of phonons which are not possible to detect using diffraction experiments.20,21 The dynamic distortion in LCO depends upon the intermediate state (IS) of Co(III): t5e1 which is a strong Jahn–Teller (JT) ion. Depending on the JT effect, the crystal structure changes from rhombohedral to monoclinic. According to the factor group analysis, the rhombohedral perovskite exhibits irreducible five Raman active phonon modes representing A1g + 4Eg. Analysis of the Raman spectra of pure LaCoO3 was conducted with a laser power of 0.5 mW. The data show the presence of a vibrational peak at 630 cm−1 which corresponds to the local JT distortions in the semiconducting state and Eg stretching vibration of the rhombohedral phase.22Fig. 5(b) clearly shows that upon increasing the laser power to 5 mW the intensity of the band is decreased. This might be associated with the material transition from semiconducting to metallic states where the more distorted e electrons in the transition stage change to less distorted σ* electrons with intermediate state IS Co(III): t5σ*. This occurs due to laser heating on the surface of LCO.20 Subsequently, LLCO, LMCO, LCCO, LSCO and LBCO were analyzed with a laser power of 0.5 mW as shown in Fig. 5(a). Upon doping with magnesium, calcium, strontium, and barium, the observed Raman peaks were found at 664 cm−1, 656 cm−1, 660 cm−1, and 651 cm−1, respectively, corresponding to the intermediate state (IS) of Co(III): t5e1 in lanthanum cobaltites. Incorporation of different dopants in LCO is revealed by the slight shift in the peak positions of doped compositions presented in Fig. 5(a).
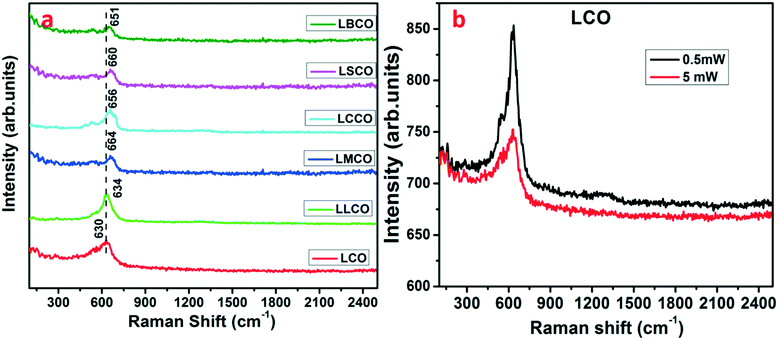 |
| Fig. 5 Raman spectroscopic data of (a) sintered pellets at a laser power of 0.5 mW and (b) LCO at different laser powers. | |
3.4 Electrical characterization
Impedance spectroscopy stands as one of the useful techniques for investigating the substitutional effect of various elements in the host material on electron and ion transport mechanisms. The charge carrier dynamics of intergrains, intragrains and electrodes can be easily and effectively analysed using impedance spectroscopy.23,24 The Nyquist plots drawn with the real and imaginary parts of impedance at different temperatures are shown in Fig. 6. The low frequency intercept of the Nyquist plot on the real axis indicates the DC resistance of the material. For low frequencies and low temperatures, Z′ is high and vice versa. Hence when the temperature increases the resistance decreases (provided in the ESI†) which can be due to thermal activation of electrons resulting in the drift from one atomic site to another.25–28 The presence of two semicircles in the Nyquist plot stands as the evidence of multiple relaxation processes occurring in the material. The high frequency intercept corresponds to the grain boundary resistance and the low frequency intercept provides the resistance of grain interiors. Grain boundaries are found to be more resistive than grains due to the presence of unfavorable defects at the grain boundaries.
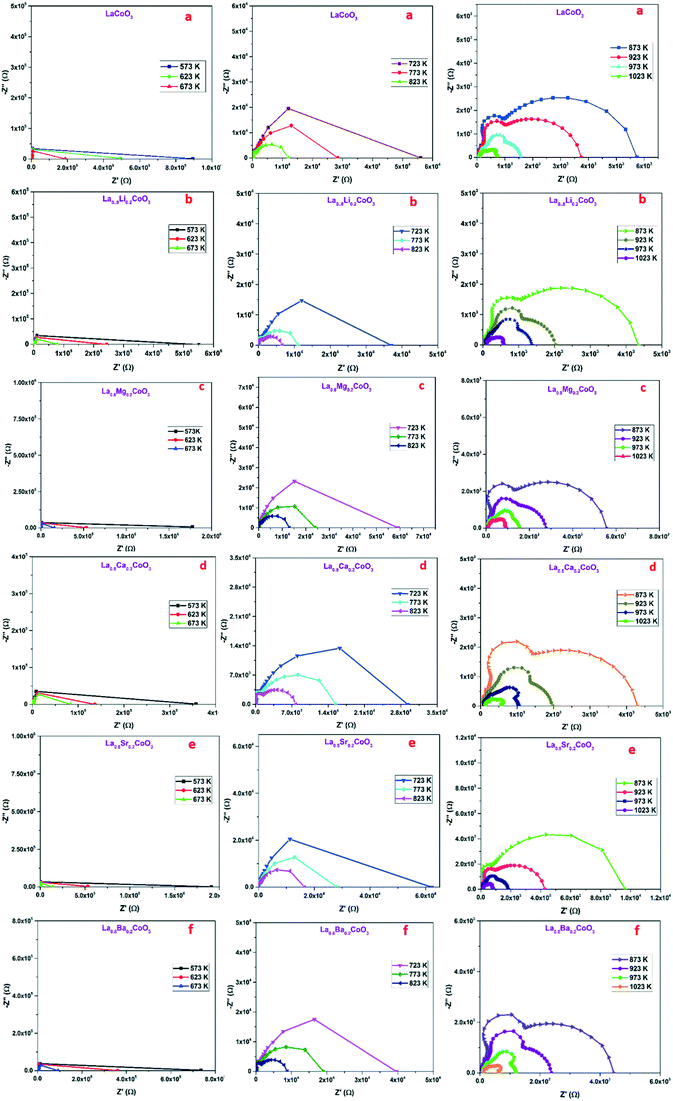 |
| Fig. 6 Nyquist plots of (a) LCO, (b) LLCO, (c) LMCO, (d) LCCO, (e) LSCO and (f) LBCO in a wide temperature range from 573 K to 673 K, 723 K to 823 K and 873 K to 1023 K from left to right. | |
However, variation in the diameter of the semicircles corresponding to grains and grain boundaries at different temperatures leads to the behavioral investigation by modulus spectroscopy as shown in Fig. 7. While impedance spectroscopy highlights the resistive elements, modulus spectroscopy stresses on capacitive components.29 Upon increasing the temperature, a low value of M′ in the low frequencies and a monotonic dispersion in the higher frequencies can be characterized in the modulus spectroscopic analysis. The decrease in the maximum peak of M′ upon the increase in temperature shows the uniform relaxation behavior of the sample. Also, the presence of the maximum peak at a fixed point suggests that relaxation is independent of temperature.30,31 At higher frequencies all the well-defined peaks of M′ show a converging behavior towards the real axis. Symmetrical peaks suggest the Debye-type behavior of the samples; however, multiple relaxation phenomena (τ = RC and ωRC = 1) occurring in the sample are evident through the presence of two distinct peaks. The symmetric maximum at higher frequencies also suggests the absence of space-charge polarization for all the samples.32
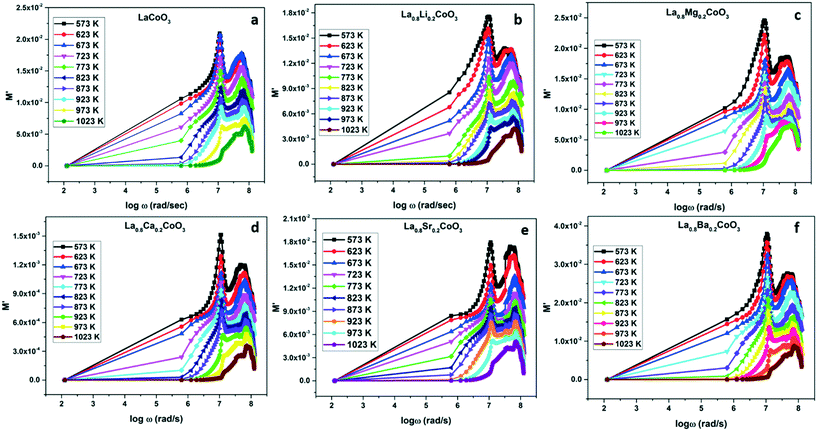 |
| Fig. 7
M′ versus log ω plots of (a) LCO, (b) LLCO, (c) LMCO, (d) LCCO, (e) LSCO and (f) LBCO in a wide temperature range from 573 K to 1023 K. | |
Fig. 8 shows the total DC conductivity (log
σT) versus the inverse of temperature (1000/T) of sintered perovskites. A linear plot revealing Arrhenius behavior can be observed according to eqn (6). From the figure, it is clear that after doping monovalent and divalent anions in the La site, there is an increase in total conductivity with the increase in temperature. The defects in the form of oxygen vacancies, which are already present due to the existence of cobalt in multiple oxidation states or induced by doping divalent/monovalent cations in the trivalent La site, play a prominent role in the enhancement of conductivity.33 In addition, the increased carrier mobility due to the increase in temperature also contributes to the improved conductivity at higher temperature. However, the decreased carrier mobility due to the decreased unit cell volume in LLCO and LMCO and also the formation of neutral defects in the case of LLCO could be the reason for their lower total conductivity values when compared to that of LCCO. The presence of larger substituent Ba and Sr atoms in the position of smaller La atoms may also affect the mobility of the carriers, thus decreasing the total conductivity of LBCO and LSCO when compared to that of LCCO as evident from their Arrhenius plots. Comparable values of activation energies of all the compositions imply that the ion blocking mechanisms of grain boundaries among the studied materials are similar.33 The measured values of activation energies for the electrical conductivity are in the range of 0.72–0.85 eV. The activation energy in this range and also the single slope feature of the Arrhenius plots indicate that the conductivity in these materials could be predominantly ionic.34–41
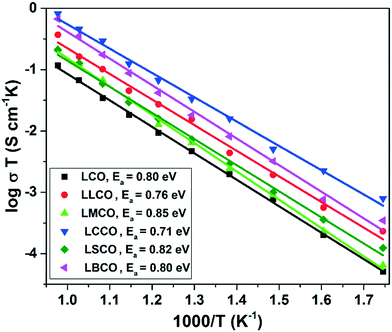 |
| Fig. 8 Variation of conductivity with respect to temperature for the synthesized compounds. | |
4. Conclusion
The present work comprises the temperature-dependent electrical conductivity studies of LaCoO3 and La0.8A0.2CoO3 (A – Li, Mg, Ca, Sr, Ba) prepared by a sol–gel method. The effective incorporation of the dopants was confirmed by the phase purity and variation in cell dimensions of each individual composition as observed by XRD analysis and also the peak shift in Raman spectroscopy analysis. Temperature-dependent electrical conductivity studies revealed that the dopants are effective in enhancing the total conductivity of LaCoO3. Contribution from grains and grain boundaries has significantly influenced the material as evident from the Nyquist plot and subsequently confirmed by modulus spectroscopy. Among the dopants, calcium and barium are more effective in improving the electrical properties of LaCoO3 than the other dopants. The cobaltites reported in this work are inexpensive materials which can be synthesized by fast processing methods and can be used for energy related applications like supercapacitors, thermoelectrics, solid oxide fuel cells (SOFCs), etc.
Conflicts of interest
There are no conflicts to declare.
Acknowledgements
The authors would like to thank the following members from the PSG Institute of Advanced Studies, Coimbatore: Rakkiraj R for the SEM analysis, Rajesh R for Raman spectroscopy, and Haripriya M and Althaf R for extending help during the overall research work.
Notes and references
- X. Lang, H. Mo, X. Hu and H. Tian, Supercapacitor performance of perovskite La1−xSrxMnO3, Dalton Trans., 2017, 46, 13720–13730 RSC.
- W. S. Yang, J. H. Noh, N. J. Jeon, N. J. Jeon, Y. C. Kim, S. Ryu, J. Seo and S. IlSeok, High-performance photovoltaic perovskite layers fabricated through intramolecular exchange, Science, 2015, 348, 1234–1237 CrossRef CAS PubMed.
- Q. Rong, Y. Zhang, C. Wang, Z. Zhu, J. Zhang and Q. Liu, A high selective methanol gas sensor based on molecular imprinted Ag-LaFeO3 fibers, Sci. Rep., 2017, 7, 12110–12119 CrossRef.
- R. Lan, P. I. Cowin, S. Sengodan and S. Tao, A perovskite oxide with high conductivities in both air and reducing atmosphere for use as electrode for solid oxide fuel cells, Sci. Rep., 2016, 6, 31839–31849 CrossRef CAS.
- R. Schmidt, J. Wu, C. Leighton and I. Jerry, Dielectric response to the low temperature magnetic defect structure and spin transition in polycrystalline LaCoO3, Phys. Rev. B: Condens. Matter Mater. Phys., 2009, 79, 125105–125114 CrossRef.
- M. Imada, A. Fujimori and Y. Tokura, Metal-insulator transitions, Rev. Mod. Phys., 1998, 70, 1039–1263 CrossRef CAS.
- T. Ishimoto, Y. Ito, T. Tada, R. Oike, T. Nakamura, K. Amezawa and M. Koyama, Theoretical study on temperature effect of electronic structure and spin state in LaCoO3 by using density functional theory, Solid State Ionics, 2015, 285, 195–201 CrossRef.
- T. Ishimoto, Y. Ito, H. Kohno and M. Koyama, Density Functional Theory Calculation of Spin-State Transition in LaCoO3, ECS Trans., 2013, 57(1), 2655–2660 CrossRef.
- S. Thirumalairajan, K. GirijaValmor, R. Mastelaro and N. Ponpandiyan, Investigation on magnetic and electric properties of morphologically different perovskites LaFeO3 nanostructures, J. Mater. Sci.: Mater. Electron., 2015, 26, 8652–8662 CrossRef CAS.
- J. Sunarso, S. Baumann, J. M. Serra, W. A. Meulenberg, S. Liu and J. C. Diniz da costa, Mixed Ionic-Electronic Conducting (MIEC) ceramic-based membranes for oxygen separation, J. Membr. Sci., 2008, 320, 13–41 CrossRef CAS.
- J. Mizusaki, M. Yoshihiro, S. Yamauchi and K. Fueki, Nonstoichiometry and Defect Structure of the Perovskite-Type Oxides La1−xSrxFeO3, J. Solid State Chem., 1985, 58(2), 257–266 CrossRef CAS.
- W. Yang, H. Wang, X. Zhu and L. Lin, Development and Application of Oxygen Permeable Membrane in Selective Oxidation of Light Alkanes, Top. Catal., 2005, 35, 155–167 CrossRef CAS.
- X. Chen and T. Grande, Anisotropic and Nonlinear Thermal and Chemical Expansion of La1−xSrxFeO3−δ (x = 0.3, 0.4, 0.5) Perovskite Materials, Chem. Mater., 2013, 25, 3296–3306 CrossRef CAS.
- B.-K. Park, R.-H. Song, S.-B. Lee, T.-H. Lim, S.-J. Park, C.-O. Park and J.-W. Lee, Facile Synthesis of Ca-Doped LaCoO3 Perovskite via Chemically Assisted Electrodeposition as a Protective Film on Solid Oxide Fuel Cell Interconnects, J. Electrochem. Soc., 2016, 163, 1066–1071 CrossRef.
- R. Kun, S. Populoh, L. Karvonen, J. Gumbert, A. Weidenkaff and M. Busse, Structural and thermoelectric characterization of Ba substituted LaCoO3 perovskite-type materials obtained by polymerized gel combustion method, J. Alloys Compd., 2013, 579, 147–155 CrossRef CAS.
- H. Masuda, T. Fujita, T. Miyashita, M. Soda, Y. Yasui, Y. Kobayashi and M. Sato, Transport and Magnetic Properties of R1xAxCoO3 (R@La, Pr and Nd; A@Ba, Sr and Ca), J. Phys. Soc. Jpn., 2003, 72, 873–878 CrossRef CAS.
- R. Robert, M. H. Aguirre, P. Hug, A. Reller and A. Weidenkaff, High-temperature thermoelectric properties of Ln(Co, Ni)O3 (Ln@La, Pr, Nd, Sm, Gd and Dy) compounds, Acta Mater., 2007, 55, 4695–4972 CrossRef.
- K. Muta, Y. Kobayashi and K. Asai, Magnetic, electronic transport, and calorimetric investigations of La1xCaxCoO3 in comparison with La1xSrxCoO3, J. Phys. Soc. Jpn., 2002, 71, 2784–2791 CrossRef CAS.
- J.-M. Li and X.-L. Zeng, Partial cationic inversion-induced magnetic hardening of densely packed 23-nm-sized nanocrystalline-interacting nickel ferrite electrospun nanowires, Appl. Phys. Lett., 2013, 103, 232410 CrossRef.
- N. Orlovskaya and D. Steinmetz, Detection of temperature- and stress-induced modifications of LaCoO3 by micro-Raman spectroscopy, Phys. Rev. B: Condens. Matter Mater. Phys., 2005, 72, 014122 CrossRef.
- J.-M. Li and C. H. A. Huan, Magnetic-field-tunable charge carrier localization in sintered polycrystalline La0.75Ca0.25MnO3, Phys. Rev. B: Condens. Matter Mater. Phys., 2000, 63, 024416 CrossRef.
- V. Gnezdilov and A. V. Yeremenko, Low temperature mixed spin state of Co3+ in LaCoO3evidenced from Jahn-Teller lattice distortions; AIP Low temp, Physics, 2006, 32, 162 CAS.
- Z. Ahmad, S. Atiq, S. K. Abbas, S. M. Ramay, S. Riaz and S. Naseem, Structural and complex impedance spectroscopic studies of Mg-substituted CoFe2O4, Ceram. Int., 2016, 42, 18271–18282 CrossRef CAS.
- S. Kumail Abbas, M. Adnan Aslam, M. Amir, S. Atiq, Z. Ahmed, S. A. Siddiqi and S. Naseem, Electrical impedance functionality and spin orientation transformation of nanostructured Sr-substituted BaMnO3 hexagonal perovskites, J. Alloys Compd., 2017, 712, 720–731 CrossRef.
- K. Kavitha and A. Ashok, Solid-state synthesis and electrical conductivity properties of Ba3SrTa2O9 complex perovskite, Mater. Charact., 2017, 133, 17–24 CrossRef CAS.
- V. D. Nithya and R. KalaiSelvan, Synthesis, electrical and dielectric properties of FeVO4 nanoparticles, Phys. B, 2011, 406, 24–29 CrossRef CAS.
- P. S. Anantha and K. Hariharan, Structure and ionic transport studies of sodium borophosphate glassy system, Mater. Chem. Phys., 2005, 89, 428–437 CrossRef CAS.
- V. Vasanthi, A. Shanmugavani, C. Sanjeeviraja and R. KalaiSelvan, Microwave assisted combustion synthesis of CdFe2O4: magnetic and electrical properties, J. Magn. Magn. Mater., 2012, 324, 2100–2107 CrossRef CAS.
- N. Padmamalini and K. Ambujam, Impedance and modulus spectroscopy of ZrO2eTiO2eV2O5 nanocomposite, Karbala International Journal of Modern Science, 2016, 1–5, DOI:10.1016/0022-4596(90)90271-X.
- A. Shukla, R. N. P. Choudary, A. K. Thakur and D. K. Pradhan, Structural, microstructural and electrical studies of La and Cu doped BaTiO3 ceramics, Phys. B, 2010, 405, 99–106 CrossRef CAS.
- B. Madhavan and A. Ashok, Characterisation and dielectric property analysis of A-site doped LaTiO3-δ perovskites synthesised by ball milling method, Adv. Mater. Lett., 2015, 6, 395–401 CrossRef CAS.
- H. Zhang, T.-Y. Sun, Y. Wang, L. B. Kong, K.-W. Kwok, T. Karaki, K.-H. Lam, C.-L. Mak and F. Hou, Studies of interface characteristics of fine grain ferroelectric based glass-ceramic composites using impedance spectroscopy, J. Alloys Compd., 2016, 682, 196–202 CrossRef CAS.
- A. Rai, P. Mehta and S. Omar, Ion conduction behavior in SmxNd0.15-xCe0.85O2-δ, Solid State Ionics, 2014, 263, 190–196 CrossRef CAS.
- W. H. Kan, M. Roushanafshar, A. Vincent, T. Fürstenhaupt, M. Parvez, J. Luo and V. Thangadurai, Effect of substitution of B-sites by Mn, Fe and Co in double perovskite-type Ba3CaNb2O9 on structure andelectrical properties, RSC Adv., 2013, 3, 23824–23832 RSC.
- A. S. Nowick and K. C. Liang, Effect of non-stoichiometry on the protonic and oxygen-ionic conductivity of Sr2(ScNb)O6: a complex perovskites, Solid State Ionics, 2000, 129, 201–207 CrossRef CAS.
- Y. Du and A. S. Nowick, High-temperature protonic conductors with perovskite-related
structures, Solid State Ionics, 1994, 77, 137–146 Search PubMed.
- H. D. A. L. Viana and J. T. S. Irvine, Characterisation of lower temperature sintered zinc-doped barium calcium niobate proton conducting electrolytes, J. Mater. Chem., 2010, 20, 8506–8511 RSC.
- S. Wang, F. Zhao, L. Zhang, K. Brinkman and F. Chen, Doping effects on complex perovskite Ba3Ca1.18Nb1.82O9−ı intermediate temperature proton conductor, J. Power Sources, 2011, 196, 7917–7923 CrossRef CAS.
- S. S. Bhella and V. Thangadurai, Synthesis and characterization of carbon dioxide and boiling water stable proton conducting double perovskite-type metal oxides, J. Power Sources, 2009, 186, 311–319 CrossRef CAS.
- T. T. Trinh and V. Thangadurai, Effect of Ti substitution for Nb in double perovskite-type Ba 3CaNb2O9 on chemical stability and electrical conductivity, Electrochim. Acta, 2010, 56, 227–234 CrossRef CAS.
- M. Li, M. J. Pietrowski, R. A. De Souza, H. Zhang, I. M. Reaney, S. N. Cook, J. A. Kilner, D. C. Sinclair, M. Li and D. C. Sinclair, A family of oxide ion conductors based on the ferroelectric perovskite Na0.5Bi0.5TiO3, nature, Mater. Lett., 2013, 13, 31–35 CrossRef.
Footnote |
† Electronic supplementary information (ESI) available. See DOI: 10.1039/c9ce01464k |
|
This journal is © The Royal Society of Chemistry 2020 |
Click here to see how this site uses Cookies. View our privacy policy here.