The molecular interactions of organic compounds with tire crumb materials differ substantially from those with other microplastics†
Received
18th September 2019
, Accepted 30th October 2019
First published on 30th October 2019
Abstract
Tire materials are the most commonly found elastomers in the environment and they account for a significant fraction of microplastic pollution. In the discussions on the environmental impact of microplastics tire materials and their sorption properties have been largely overlooked. In this study we used experimental sorption data from six organic probe sorbates sorbing to two tire materials and their major components, styrene butadiene rubber and carbon black, to gain a better understanding of the underlying sorption processes of tire materials. Commonly applied models used to describe non-linear sorption processes were unable to fully explain sorption to tire materials but showed that absorption into the rubber fraction dominated the sorption process. Hydrophobicity was approximated using the hexadecane–water partitioning constant, which correlated very well with the distribution data obtained for styrene rubber, whereas the correlations between hydrophobicity of sorbates and the sorption data to the tire materials were poor. Although hydrophobicity plays an important role in sorption to tire materials, additional interactions must be taken into account. Overall, the processes involved in sorption to tire materials differed significantly from those governing sorption to other microplastics.
Environmental significance
Tire materials are introduced into the environment by various pathways. Although they contain a substantial portion of polymers, their environmental impact has been largely unexplored in the context of microplastics research. Consequently, processes that control the interactions between organic molecules and tire materials in the environment, and how the complex composition of tires influences these interactions, remain poorly understood. In this study, we investigated the sorption of six organic molecular probe sorbates to two tire materials and their major components, styrene-butadiene rubber and carbon black. The results imply that sorption of organic compounds to tire crumb materials highly resembles sorption to the rubber fraction and less sorption to carbon black. This demonstrates that sorption to tire materials differs substantially from sorption to other microplastics.
|
1 Introduction
Unlike thermoplastics and thermosets, elastomers have only very recently been considered as environmental plastic debris,1 although according to the International Organization for Standardization they fulfill the definition of plastics.2 Tire materials account for up to 70% of this diverse group of emerging particulate contaminants released into the environment,3,4 but they are still rarely included in estimations of plastic pollution. Among the many different components of modern tires are different rubber types (40–60%), mostly styrene butadiene rubber and natural rubber,5 that are compounded with carbon black (20–35%) or, more recently, silica. Environmental inputs of tire materials occur via two major input pathways: unintentionally, e.g., due to abrasion followed by road surface runoff,6 and intentionally, such as the use of recycled and shredded tire crumb rubber as filling material for playgrounds or on artificial turf fields.7 Tire materials, especially tire crumb rubber, also have a high sorption capacity for organic molecules and have therefore been proposed as a cost-effective sorbent for the removal of organic pollutants from water.8,9 The processes involved in the interactions between organic molecules and tire materials in the environment, and how the complexity of tire composition affects these interactions, are poorly understood.
As with microplastics, the role of tire materials as a vector for organic contaminants is under debate. Investigations into sorption to tire material have been limited to a restricted range of sorbates at relatively high concentrations.10–12 The organic sorbates used as probes have been mostly limited to compounds detected in tire materials, such as polycyclic aromatic hydrocarbons9,13 and gasoline-derived compounds (e.g., BTEX compounds).10,13 Experiments involving two tire materials with different proportions of rubber components showed that the tire rubber composition had no significant effect on the sorption of hydrophobic organic compounds and that sorption to rubber correlated well with the hydrophobicity of the investigated sorbates (toluene and naphthalene).13 A more diverse set of probe compounds is required to investigate the importance of hydrophobic interactions in sorption to tire materials. Alamo-Nole et al. (2011) examined the sorption of toluene and xylene to tire crumb rubber and to its main components, carbon black and styrene butadiene rubber.10 The observed linear sorption to styrene butadiene rubber was interpreted as being mainly driven by polymer–water partitioning rather than by adsorption onto the sorbent's surface. The sorption to tire crumb rubber (as a composite sorbent) was interpreted as being a combination of adsorption onto carbon black and absorption into the rubber matrix.9 The aim of most of those studies was to assess the performance of tire materials compared to that of activated carbon or tire-derived chars in the removal of organic pollutants from aqueous solutions.14,15 Their results showed that both compound-specific factors and the properties of the tire materials need to be taken into account to achieve a comprehensive understanding of the interactions involved.
The complexity of tire materials and their different input pathways into the environment through unintentional input of tire wear or intentional input of tire crumb rubber makes it particularly important to achieve a comprehensive understanding of the interactions between these materials and organic pollutants.16 An In-depth investigations of the sorption processes affecting organic compounds in environmental systems are critical for environmental risk assessments. Given the complexity of tire materials and the different pathways of their input into the environment, a comprehensive understanding of the interactions between these materials and organic pollutants is particularly important.16 In this study we examined the interactions of tire materials and their major components styrene butadiene rubber and carbon black with organic compounds by conducting sorption experiments using probe sorbates carefully selected on the basis of their physico-chemical properties.
2 Methods
2.1 Materials
Two tire crumb rubber materials were used during this study. One tire material (TCR-S) was collected from a soccer field in Vienna, Austria and milled with liquid nitrogen using a centrifugal mill (Rotsch, UZM 200); the other tire material (TCR-R) was provided by General Recycling GmbH (Vienna, Austria), a tire recycling and shredding company. Both were sieved to a mesh size of 125–250 μm. Polystyrene–polybutadiene rubber containing 30% styrene was obtained from Sigma Aldrich and sieved to a similar mesh size as the tire materials. Carbon black (mean particle size of 95 nm) was obtained from PyroPowders. Apart from milling or sieving, the sorbents were used without any further treatment. The organic probe sorbates used in this study were n-hexane (VWR Chemicals) cyclohexane (Acros Organics), benzene, chlorobenzene, di-n-propylether (all Sigma Aldrich) and 2,6-dimethyl-2-heptanol (Alfa Aesar). Their physico-chemical properties are listed in Table 1. All sorbates were purchased as ≥99% pure chemicals. Sorbate stock solutions were freshly prepared in methanol (residual analysis grade: Acros Organic, Geel Belgium), kept in the dark at 4 °C for ≤3 weeks and used only for determination of one sorption isotherm.
Table 1 Physico-chemical properties of the sorbates used in this studya
Sorbate |
V
m [cm3 mol−1] |
S
w [mg L−1] |
log Kaw [—] |
log Kow [—] |
V
m: molar volume; Sw: aqueous solubility; log Kaw: air–water partitioning constant; and log Kow: octanol–water partitioning constant from ref. 17.
|
n-Hexane |
127.6 |
20 |
1.73 |
3.29 |
Cyclohexane |
106.5 |
70 |
0.78 |
3.21 |
Benzene |
89.4 |
2000 |
−0.64 |
1.99 |
Chlorobenzene |
101.4 |
400 |
−0.80 |
2.64 |
Di-n-propylether |
133.9 |
2130 |
−1.05 |
2.03 |
2,6-Dimethylheptan-2-ol |
175 |
570 |
−2.77 |
3.11 |
2.2 Sorbent characterization
Gas-physisorption isotherms for N2 and CO2 were measured on a Quantachrome Instruments Nova 2000 analyzer. The samples were outgassed overnight (16 h, 105 °C) under vacuum prior to the measurements. The BET specific surface area (SSA) of the N2 isotherms was determined from six points within the pressure region P/P0 = 0.01–0.30 using the BET equation.18 Data analysis was performed using instrument software (NovaWin 11.0, Quantachrome Instruments). The built-in, non-local density functional theory model was used to determine pore size-distribution (for pores sizes of 2.96–13.02 nm) in the pressure region P/P0 = 0.01–0.98, assuming slit-shaped pores. For CO2 isotherms, a built-in grand canonical Monte Carlo simulation was used to determine the cumulative surface area, the cumulative pore volume, and the pore size-distribution (for pore sizes 0.70–2.96 nm) in the pressure region P/P0 = 0.001–0.030, again assuming slit-shaped pores. Sorbent particle size distributions were determined in dispersions using a particle size and -shape analyzer based on laser light shading (time of transition principle) and simultaneous microscopy (EyeTech, Ankersmid Lab, Nijverdal, The Netherlands). Two mL of the dispersions were transferred into 3 mL PMMA semi-microcuvettes and the samples were measured in triplicate.
2.3 Sorption batch experiments
Sorption experiments were performed in three-phase systems to avoid the need for a phase separation step.19,20 The amount of sorbent required to achieve a robust mass balance for sorption (i.e. 30–90% mass of sorbate sorbed) ranged between 5 and 50 mg. The sorbents were pre-equilibrated for 12 h in 10 mL of aqueous 10 mM CaCl2 as background solution using amber 20 mL headspace vials. They were then spiked with methanolic sorbate solutions to obtain single-sorbate concentrations ranging over three orders of magnitude. The methanolic content in the vials did not exceed 0.25% (v/v), to avoid co-solvent effects.17 Duplicates of all samples were analyzed to ensure reproducibility. Loss of analyte and the amount of analyte leaching out of the materials during equilibration was monitored in triplicates samples. Blanks were prepared with or without sorbent materials and no spiking of sorbates. For loss of sorbates vials without sorbent were spiked with sorbates. The sorbent–sorbate mixtures were shaken at room temperature (25 °C, 125 rpm) until (pseudo-) equilibrium was obtained (14 days). Equilibration was determined in preliminary experiments using n-hexane as the probe sorbate. The vials were placed in the tray of an autosampler for at least 2 h before the analysis to ensure equilibrium between the liquid and gaseous phases.21 Sorbate concentrations in the headspace were determined using GC/MS (Agilent 7890A gas chromatography coupled with 5975C mass spectrometry) using in-tube microextraction.22,23 Quantification was achieved by external calibration, using calibration standards prepared one day prior to the analysis. An HP-5ms column (25 m × 250 μm × 0.25 μm, J & W Scientific) was used. Nitrogen (N2, purity 5.5, Linde Gas) served as the carrier gas. Air–water partitioning constants (Kaw in Table 1) were used to calculate the distribution of the sorbates between the headspace, aqueous phase and sorbent.
2.4 Data analysis
Four sorption models were fit to the experimental sorption isotherms; the models' equations and their fitting parameters are listed in Table 2. These models are commonly used to fit sorption data for carbonaceous sorbents, microplastics and tire materials.15,20,24 The curve-fitting results were evaluated using the coefficient of determination (R2), the root mean square error (RMSE), and Akaike's information criterion (AIC). The sum of the squared residuals was used to calculate R2, which does not take the number of fitting parameters into account, unlike AIC. The AIC is based on information theory25 and was used to compare the fit of the different models of sorption to tire materials and their major components based on probabilities and evidence ratios. All data analyses were performed using Sigma Plot 12.0 software for Windows. Standard errors for the models' parameters were computed using reduced chi squared and regressions weighted to a reciprocal y2.
Table 2 Non-linear sorption models used for isotherm data fittinga
Sorption model |
Equation |
Fitting parameters |
p
|
C
s: equilibrium sorbed concentration [μg kg−1]; Cw: equilibrium aqueous concentration [μg L−1]; εsw = −RT ln(Csat/Cw) [kJ mol−1]: effective sorption potential; Vm: molar volume [cm3 mol−1]; R [8.314 × 10−3 kJ mol−1 K]: universal gas constant; T [K]: absolute temperature; Csat: aqueous solubility.
p: number of fitting parameters.
|
Freundlich |
C
s = KFCwn |
K
F: Freundlich affinity coefficient [(μg kg−1)/(μg L−1)]1/n, n: Freundlich exponential coefficient [—] |
2 |
Langmuir |
|
Q
max: maximum capacity [μg kg−1], KL: Langmuir affinity coefficient [μg L−1] |
2 |
Dual mode Langmuir |
|
K
p: partitioning coefficient [L kg−1], Qmax: maximum capacity [μg kg−1], KL: Langmuir affinity coefficient [μg L−1] |
3 |
Polanyi–Manes |
|
Q
max: maximum capacity [μg kg−1], a: fitting parameter, d: fitting parameter |
3 |
3 Results and discussion
3.1 Non-linear sorption models do not fully explain sorption to tire materials
Sorption to carbon black did not follow linear pattern, as indicated by the Freundlich coefficient (n), which ranged between 0.299 and 0.671 (Fig. 1 and Tables S1–S6†). Sorption closer to linearity was obtained for styrene butadiene rubber (n = 0.741–0.963), TCR-R (n = 0.815–1.079), and TCR-S (n = 0.667–1.016). The differences compared to sorption to other microplastics were pronounced; for example, the sorption of a similar set of organic probe sorbates to polyethylene was highly linear (n > 0.96).20 For other polymers such as polystyrene or polyvinyl chloride, non-linear sorption was observed.20 Given the generally non-linear sorption to all four sorbents investigated, the experimental sorption data were fit to commonly used non-linear isotherm models, i.e. the Freundlich model, Langmuir model, dual-mode Langmuir model, and the Polanyi–Manes model.
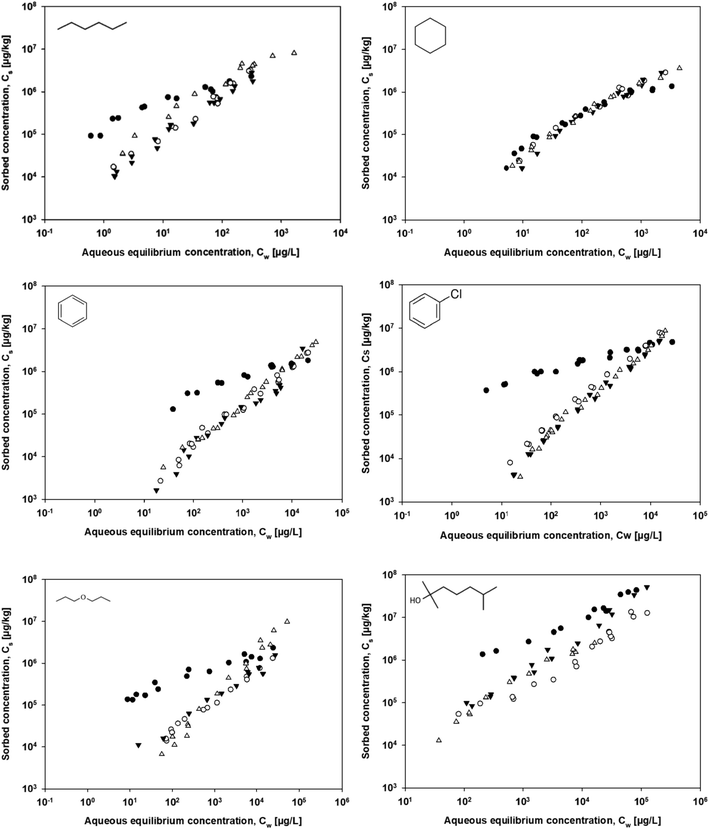 |
| Fig. 1 Isotherms for the sorption of the probe sorbates to (●) carbon black, (○) styrene butadiene rubber, (▲) TCR-S, and (Δ) TCR-R. | |
The goodness of fit for the sorption isotherms of carbon black to the Polanyi–Manes model yielded the highest R2 values (R2 > 0.965) and the lowest RMSE (<0.191) and AIC (<−42.16) values. Among the three quality criteria, the use of the AIC to compare the different sorption models ensured that a better goodness of fit was not due to over-parameterization, as an increase in the degrees of freedom from two to three parameters characterizes the considered sorption models (i.e. from the Freundlich model to the dual mode Langmuir model to the Polanyi–Manes model). The AIC was used to quantify potential discrepancies between sorption models differing in their fitting parameters.24,26 For carbon black, sorption was expected to be mainly driven by adsorption onto the sorbent surface and be far less governed by partitioning into tar-like structures.27 While the best fit results for sorption to carbon black were obtained using the Polanyi–Manes model, for four out of the six sorbates the dual-mode Langmuir model resulted in a statistically better fit than obtained with the Freundlich model. This result implied a contribution of absorption to the process of sorption to carbon black, which could stem from tar-like liquid phases of the carbon black.27 For the other sorbents, the model that best fit the experimental data could not be similarly determined. Literature reports on the optimal sorption model for tire materials are also unclear. The sorption of triclosan to tire crumb and styrene butadiene rubber was better described by the Langmuir model than by the Freundlich model,11 whereas the sorption of gasoline, toluene, and o-xylene to tire crumb was better fit using the Freundlich than the Langmuir model.9 Sorption to tire materials is expected to be a combination of adsorption onto carbonized carbon black and absorption into the non-carbonized rubber phase and therefore should best be described using more complex sorption models that take both ad- and absorption into account, as is the case with the dual-mode Langmuir model.15 A comparison of the AIC values calculated for the Langmuir and dual-mode Langmuir models showed little improvement in the goodness of fit achieved when the latter was used. The use of the dual-mode Langmuir model fit parameters to calculate the amounts of ad- and absorption by the two tire materials at an aqueous equilibrium concentration of 0.001 of their solubility, as previously done for tire materials and their chars,15 did not reveal whether one process dominates over the other. In the case of carbon black, however, the contribution of adsorption clearly exceeded that of absorption.
The significance of the statistical fit of the experimental data and the conclusion that the data are better fit using one sorption model rather than another clearly depend, among other factors, on the quality of the experimental data and the concentration range over which the sorption isotherms were determined. A comprehensive understanding of the models applied to fit the sorption isotherms is necessary to avoid not only misapplication but also inaccurate conclusions.16,28 Isotherm data fit using the Polanyi–Manes model resulted in the best goodness of fit for sorption to carbon black and well explained sorption to styrene butadiene rubber, TCR-S and TCR-R. This result shows a heterogeneous distribution of the sorption site energies of the investigated sorbents and that no saturation-limited sorbate monolayers had formed on the sorbent surfaces.
The heterogeneous distribution of the sorption site energies of the sorbent in turn explained the non-linearity of sorption. For carbon black, the BET data were consistent with the presence of micropores (<2 nm) and therefore pore-surface adsorption (pore-filling) as a potential sorption process (Fig. S1†). Polanyi–Manes theory-based sorption models have been shown to adequately describe non-linear sorption to other porous carbonaceous sorbents.24,29 The results from the fitting of the experimental sorption data to the non-linear sorption models (Table 3) showed that the Polanyi–Manes model best explained the sorption of the probe sorbates to carbon black, whereas the goodness of fit for the sorption of the different sorbates to styrene butadiene rubber, TCR-S, and TCR-R varied. In another study using sorption models to investigate the non-linear sorption of non-polar organic sorbates to microplastics, the Polanyi–Manes model also generally resulted in the best fit.20 The comparison of correlation curves in which the sorbed volume was plotted against the ratio of the sorption potential to the sorbate's molar volume showed significant differences among the sorbates. Similarly, in the present study correlation curves plotted for carbon black, styrene butadiene rubber, TCR-S, and TCR-R (Fig. 2) showed significant differences between the curves for all six probe sorbates. According to the Polanyi–Manes theory, the correlation curves for the sorption of various sorbates are assumed to form a single curve.30 The variations in the correlation curves for styrene butadiene rubber, TCR-S, and TCR-R suggested that the Polanyi–Manes model does not adequately describe non-linear sorption to these materials, which is in accordance with the non-porous character of these sorbents as demonstrated by the BET data (data not shown). For carbon black however, the variations in the correlation curves could be ascribed to the morphological differences between carbon black and activated carbon, for which the Polanyi–Manes model was originally used to describe sorption.31 While the sorption model fit showed that the Polanyi–Manes model was able to statistically well fit the experimental sorption data of tire materials and microplastics, according to the correlation curves it is unable to explain the sorption processes involved.
Table 3 Goodness of fit parameters obtained from fitting the experimental sorption isotherms of probe sorbates to tire materials (TCR-S and TCR-R) and their main components (carbon black, CB, and styrene butadiene rubber, SBR) to the Freundlich, Dual-Mode Langmuir, and Polanyi–Manes modela
|
Freundlich |
Dual-mode Langmuir |
Polanyi–Manes |
R
2
|
RMSE |
AIC |
R
2
|
RMSE |
AIC |
R
2
|
RMSE |
AIC |
R
2: regression coefficient; RMSE: root mean square error; AIC: Akaike's information criterion.
|
CB
|
nHex |
0.951 |
0.314 |
−29.48 |
0.971 |
0.115 |
−25.99 |
0.976 |
0.184 |
−42.40 |
cHex |
0.956 |
0.257 |
−40.73 |
0.992 |
0.164 |
−64.48 |
0.994 |
0.102 |
−68.33 |
Benz |
0.964 |
0.206 |
−41.35 |
0.983 |
0.149 |
−48.35 |
0.989 |
0.121 |
−54.10 |
cBenz |
0.985 |
0.118 |
−82.94 |
0.943 |
0.233 |
−54.10 |
0.989 |
0.103 |
−86.65 |
DNPE |
0.959 |
0.296 |
−33.69 |
0.951 |
0.226 |
−44.86 |
0.965 |
0.191 |
−86.65 |
DMH |
0.967 |
0.208 |
−34.53 |
0.986 |
0.143 |
−41.08 |
0.987 |
0.137 |
−42.16 |
![[thin space (1/6-em)]](https://www.rsc.org/images/entities/char_2009.gif) |
TCR-S
|
nHex |
0.962 |
0.234 |
−46.71 |
0.963 |
0.239 |
−44.15 |
0.963 |
0.239 |
−44.14 |
cHex |
0.959 |
0.260 |
−40.28 |
0.969 |
0.235 |
−41.62 |
0.983 |
0.173 |
−51.51 |
Benz |
0.921 |
0.377 |
−30.45 |
0.930 |
0.369 |
−29.31 |
0.939 |
0.345 |
−31.62 |
cBenz |
0.982 |
0.171 |
−82.33 |
0.976 |
0.180 |
−78.45 |
0.989 |
0.140 |
−90.35 |
DNPE |
0.965 |
0.248 |
−27.36 |
0.960 |
0.282 |
−21.95 |
0.965 |
0.261 |
−23.64 |
DMH |
0.956 |
0.247 |
−42.00 |
0.969 |
0.217 |
−44.16 |
0.972 |
0.208 |
−45.61 |
![[thin space (1/6-em)]](https://www.rsc.org/images/entities/char_2009.gif) |
TCR-R
|
nHex |
0.918 |
0.371 |
−22.77 |
0.973 |
0.228 |
−33.21 |
0.978 |
0.206 |
−35.81 |
cHex |
0.914 |
0.366 |
−31.72 |
0.983 |
0.170 |
−55.69 |
0.987 |
0.283 |
−38.61 |
Benz |
0.989 |
0.326 |
−72.48 |
0.991 |
0.254 |
−74.36 |
0.990 |
0.290 |
−71.84 |
cBenz |
0.956 |
0.278 |
−56.35 |
0.964 |
0.330 |
−46.89 |
0.971 |
0.308 |
−50.17 |
DNPE |
0.953 |
0.270 |
−39.09 |
0.931 |
0.337 |
−30.12 |
0.953 |
0.279 |
−36.17 |
DMH |
0.957 |
0.271 |
−28.17 |
0.985 |
0.169 |
−37.18 |
0.993 |
0.112 |
−46.98 |
![[thin space (1/6-em)]](https://www.rsc.org/images/entities/char_2009.gif) |
SBR
|
nHex |
0.969 |
0.226 |
−41.82 |
0.974 |
0.215 |
−41.29 |
0.979 |
0.193 |
−44.53 |
cHex |
0.956 |
0.269 |
−31.13 |
0.971 |
0.220 |
−34.13 |
0.975 |
0.211 |
−35.15 |
Benz |
0.962 |
0.257 |
−57.24 |
0.965 |
0.251 |
−56.76 |
0.964 |
0.254 |
−56.18 |
cBenz |
0.991 |
0.119 |
−87.03 |
0.995 |
0.097 |
−93.87 |
0.996 |
0.085 |
−99.17 |
DNPE |
0.981 |
0.150 |
−50.27 |
0.984 |
0.143 |
−49.47 |
0.981 |
0.156 |
−46.97 |
DMH |
0.923 |
0.394 |
−28.97 |
0.969 |
0.227 |
−45.90 |
0.951 |
0.283 |
−38.37 |
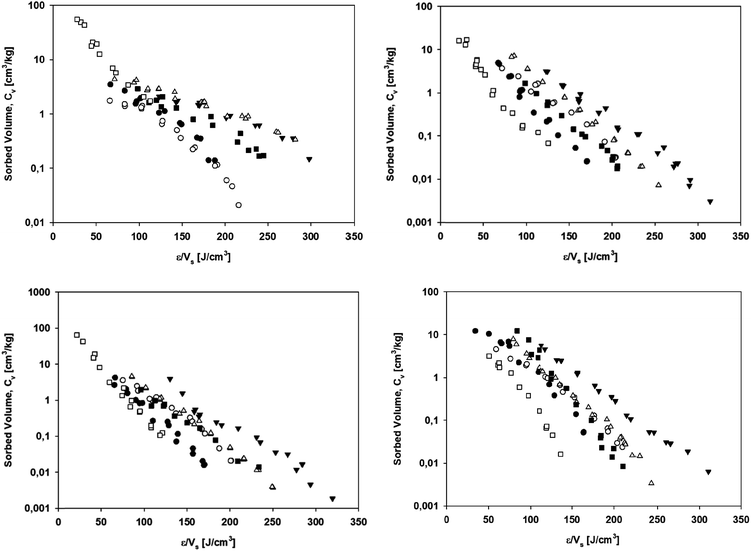 |
| Fig. 2 Correlation curves for the sorption of probe sorbates to carbon black (upper left), styrene butadiene rubber (upper right), TCR-S (lower left), and TCR-R (lower right). | |
3.2 Sorption to tire materials is dominated by absorption into the rubber fraction
To compare the sorption properties of the investigated sorbents, the distribution coefficients (log
KD) were calculated from the isotherm data at 10−3 of the sorbate solubility (Table S7†). Sorption was strongest to carbon black, which can be well explained by its particle size, which in aqueous suspension (2.65 ± 0.54 μm) was much smaller than that of the other sorbents. For carbon black, log
KD values ranged between 3.25 for benzene and 5.03 for n-hexane (Table S7†). The sorption of benzene to carbon black was the weakest among the investigated sorbates; among all sorbates benzene had the lowest hydrophobicity. Other molecular interactions including π–π electron-donor–acceptor interactions, which in combination with hydrophobic interactions were shown to result in the strong sorption of aromatic sorbates to carbonaceous sorbents and polystyrene microplastics.32 For styrene butadiene rubber and the two tire materials, the log
KD values (Table S7†) were one to approximately two orders of magnitude smaller than those of carbon black (with the exception of the smaller differences obtained with cyclohexane). This can be explained by the larger particle sizes of aqueous dispersions of these sorbents (styrene butadiene rubber: 204.77 ± 10.47 μm; TCR-S: 260.08 ± 10.54 μm; TCR-R: 211.36 ± 44.54 μm) than of carbon black. The presence of the carbon filler material in the tire materials did not result in an overall stronger sorption than observed for rubber. In fact, there was no clear trend between sorption strength and the particle size of the rubber-containing sorbents. The styrene butadiene rubber dispersion had the smallest particle size among the tested sorbents but sorption stronger than that of the tire sorbents was achieved only for the non-polar sorbates. For di-n-propyl ether and 2,6-dimethylheptan-2-ol, TCR-S exhibited a stronger sorption than either styrene butadiene rubber and TCR-R. Factors other than sorbent particle size and surface area therefore contribute to the differences in sorption by different tire materials and influence the interactions between these sorbents and organic sorbates. The aromatic phenyl groups of styrene butadiene rubber and SBR-containing tire materials would also allow for the occurrence of strong π–π electron-donor–acceptor interactions but in the tire materials these cannot be distinguished from the electron-donor–acceptor interactions of carbon black. A comparison of the sorption properties of tire materials to microplastics showed that the log
KD values for styrene butadiene rubber, TCR-S, and TCR-R were similar or higher than those obtained for polyamide, polyethylene, polystyrene, and polyvinyl chloride particles of similar size range.20
One important aspect regarding the interactions of organic compounds with environmentally relevant sorbents is the sorption mode (i.e. ad- or absorption). Which of these two processes dominates has implications for the linearity of sorption and the capacity of the sorbent. The categorization of polymers based on their glass transition temperature showed the highly linear sorption of rubber-like polymers such as polyethylene, based on Freundlich exponents (n) of ∼1. Accordingly, for these polymers partitioning into the bulk materials is the dominant sorption mode.20,32,33 By contrast, adsorption onto the polymer surface in combination with a heterogeneous distribution of sorption site energies would explain the non-linear sorption (n < 0.85) exhibited by glass-like polymers such as polystyrene and polyamide.20,32–35 The linear isotherms describing the interactions of toluene and xylene with styrene butadiene rubber have been attributed to the dominance of absorption and the dependence of sorption on water–polymer partitioning, while the nearly linear sorption of the two substances to tire crumb rubber was postulated to result from a combination of absorption into styrene butadiene rubber and adsorption onto carbon black.10 A link between the dominant sorption mode and sorption linearity inferred from the fitting of experimental sorption data to the Freundlich model alone cannot always unambiguously be established, as shown for sorption to aged and unaged microplastics.19,20 This is further illustrated by the broad variation in the obtained sorption linearities for styrene butadiene rubber, TCR-R, and TCR-S (Tables S1–S6†).
An additional approach to differentiate between adsorption and absorption is to compare the equilibrium distribution coefficients (log
KD) of n-alkanes and cyclic alkanes (Kn/Kc).36 In brief, absorption from air into a bulk phase is stronger for cycloalkanes than for their linear homologues, due to the smaller cavity formation energy necessary.37 Conversely, adsorption is stronger for n-alkanes because, unlike their cyclic homologues, all of the carbon atoms can interact with the surface of the sorbent material.38,39Kn/Kc ratios have been used to discriminate between sorption modes for a large variety of sorbent materials, including bulk liquid, organic polymers, and graphitized/activated carbons.36 In this study, to examine the dominant sorption modes of tire materials and their major components, the single point distribution coefficients of n- and cyclohexane were calculated by interpolation at a sorbate air-phase concentration of 10 μg L−1.40
The Kn/Kc ratios for the sorption of C6-alkanes to styrene butadiene rubber, TCR-R, TCR-S, and carbon black were 0.28, 0.77, 0.38, and 4.48, respectively. For sorption to styrene butadiene rubber, the Kn/Kc ratio clearly showed the dominance of absorption and was similar to the ratio reported for polyethylene (Kn/Kc = 0.33)20 and organic rubber polymers such as polyisoprene (0.46) and polybutadiene (0.47).41 For sorption to carbon black the Kn/Kc ratio was consistent with adsorption as the dominant sorption mode and was in line with the ratio of 2.20–4.52 reported for graphitized carbon black.42,43
For microplastics, the glass transition temperature of the polymer influences the dominant sorption mode. Sorption to glass-like polymers below their glass transition temperature is dominated by adsorption, while in the case of rubber-like polymers above their glass transition temperature absorption is dominant.19,20,44,45 The Kn/Kc ratios of the two composite tire materials showed that sorption is mainly driven by absorption but they were higher than the ratios of clearly absorbing materials. This result was in general agreement with the linearity of the sorption isotherms, which were more linear for styrene butadiene rubber, TCR-R, and TCR-S than for carbon black. The data also showed that for absorbing polymers steric effects can be neglected.40 Instead, for absorption into the polymer matrix, diffusion of the probe sorbates into the polymer needs to be considered. Depending on the sorbent–sorbate combination, the polymer's free volume and the molecular volume of the probe sorbate influence sorbate diffusion within the polymer. The effect of both the length of the probe sorbate molecule (i.e., the alkane chain) and the molar volume on diffusion within the investigated sorbents can be considered as minimal in the case of a C6 alkane pair, given the relatively short chain length of n-hexane and the small molar volume of cyclohexane. Since the Kn/Kc ratio can also be used to interpret the dominant sorption mode of mixtures, it provides a powerful tool to investigate the sorption of composite tire materials. However, a more detailed analysis of the contributions of the two sorption modes to the overall sorption to the tire materials would require more precise information on the main components and their properties.
3.3 Hydrophobicity plays an important but not an exclusive role in sorption to tire materials
The distribution of the sorbates (log
KD) was calculated for three different concentrations depending on the sorbate's solubility (10−2 to 10−4Sw) to account for the non-linearity of sorption and the different chemical activities of the sorbates at a given concentration. These values were then correlated using a Pearson correlation with selected physico-chemical properties of the sorbates (i.e. molar volume, the octanol–water partitioning constant, log
Kow, and the hexadecane–water partitioning constant, log
Khw) to investigate the influence of those properties on sorption to the sorbents. The air–water partitioning constant (log
Kaw) and the hexadecane–air partitioning constant (L) were used to calculate log
Khw.20 The correlations between sorption to all sorbents and the molar volumes of the sorbates were low (p > 0.05). This supports the results from the sorption model fit using the Polanyi–Manes model, in which sorption to all sorbents was not fully explained by the correlation curves. The correlations between the log
KD values and the proxies for sorbate hydrophobicity were higher (p < 0.05). For carbon black these correlations have to be treated with caution since, as pointed out above, sorption to carbon black is clearly an adsorption processes, while log
Kow and log
Khw refer to an absorption process. Regression of the distribution coefficients (here 10−3Sw) against log
Khw hydrophobicity parameters yielded (eqn (1)–(4)): | log KD,CB = 0.49 log Khw + 2.03 (R2 = 0.602, p < 0.05, n = 6) | (1) |
| log KD,SBR = 0.70 log Khw + 0.84 (R2 = 0.968, p < 0.001, n = 6) | (2) |
| log KD,TCR-R = 0.67 log Khw + 0.99 (R2 = 0.878, p < 0.01, n = 6) | (3) |
| log KD,TCR-S = 0.58 log Khw + 1.13 (R2 = 0.778, p < 0.05, n = 6) | (4) |
The results from the log
KD − log
Khw correlations for styrene butadiene rubber (eqn (2)) are similar to those obtained for sorption to polyethylene.20 Based on the molecular interactions involved in the phase transfer process, a strong correlation for sorption to styrene butadiene rubber was not expected because the molecular structures of polyethylene and hexadecane are far more similar than those of styrene butadiene rubber and hexadecane and therefore so are the respective molecular interactions. For polyethylene and hexadecane, only non-specific van-der-Waals interactions characterize the interactions with the sorbates, whereas due to the aromatic character of styrene butadiene rubber additional electron donor–acceptor interactions have to be considered. Both styrene butadiene rubber and polyethylene are adsorbing organic compounds, while sorption to tire materials, similar to other microplastics such as polystyrene and polyamide, is dominated by absorption but with a relevant contribution of adsorption. This may explain the decreasing quality of log
Khw as a proxy for sorption to these sorbents. The correlations showed that hydrophobicity plays an important role in sorption to rubber-containing sorbents and that, especially for sorption to styrene butadiene rubber, log
Khw is a very good proxy. However, sorption to the tire materials cannot be explained solely by the hydrophobicity of the sorbate.
4 Conclusion
This study examined the interactions of six organic probe sorbates with tire materials and their major components, styrene butadiene rubber and carbon black were investigated. Experimental sorption isotherms were determined over several orders of magnitude and fit to non-linear sorption models, which provided insights into the processes involved in sorption. The results showed that the commonly applied sorption models used to describe non-linear sorption processes could not fully explain sorption to the tire materials, as the experimental sorption data were well-fit using the Polanyi–Manes models but the correlation curves showed discrepancies among the different probe sorbates. The use of alkanes as probe sorbates revealed that sorption to tire materials was clearly dominated by absorption into the rubber fractions, with a smaller role played by adsorption onto the carbon black component. The sorption data were correlated with hydrophobicity, which was approximated using the octanol–water and the hexadecane–water partitioning constants. The correlations between the hexadecane–water partitioning constants and the distribution data of styrene butadiene rubber were the strongest, whereas in the case of the tire materials the correlations were weaker, and weakest for the carbon black. These results demonstrated both the importance of hydrophobicity in sorption to tire materials but also the need to consider additional interactions. Further investigations into the contributions of individual molecular interactions to the overall sorption to microplastics are required, using sorption data for a much broader set of probe compounds and covering a wide variety of chemical classes.
Conflicts of interest
There are no conflicts of interest to declare.
Acknowledgements
The authors thank Manuel Schönhofer, from the University of Vienna, for his assistance with the laboratory work.
References
- N. B. Hartmann, T. Hüffer, R. C. Thompson, M. Hassellöv, A. Verschoor, A. E. Daugaard, S. Rist, T. Karlsson, N. Brennholt and M. Cole,
et al., Are We Speaking the Same Language? Recommendations for a Definition and Categorization Framework for Plastic Debris, Environ. Sci. Technol., 2019, 53(3), 1039–1047 CrossRef CAS.
-
International Organization for Standardization, Plastics - Vocabulary. ISO 472:2013, 2013.
-
C. Lassen, S. F. Hansen, K. Magnusson, N. B. Hartmann, P. Rehne Jensen, T. G. Nielsen and A. Brinch, Microplastics - Occurrence, Effects and Sources of Releases to the Environment in Denmark, Copenhagen, 2015 Search PubMed.
- C. M. Rochman, C. Brookson, J. Bikker, N. Djuric, A. Earn, K. Bucci, S. Athey, A. Huntington, H. McIlwraith and K. Munno,
et al., Rethinking Microplastics as a Diverse Contaminant Suite, Environ. Toxicol. Chem., 2019, 38(4), 703–711 CrossRef CAS.
- Y. R. Lin and H. S. Teng, Mesoporous Carbons from Waste Tire Char and Their Application in Wastewater Discoloration, Microporous Mesoporous Mater., 2002, 54(1–2), 167–174 CrossRef CAS.
- S. Wagner, T. Hüffer, P. Klöckner, M. Wehrhahn, T. Hofmann and T. Reemtsma, Tire Wear Particles in the Aquatic Environment – A Review on Generation, Analysis, Occurrence, Fate and Effects, Water Res., 2018, 139, 83–100 CrossRef CAS PubMed.
- B. Bocca, G. Forte, F. Petrucci, S. Costantini and P. Izzo, Metals Contained and Leached from Rubber Granulates Used in Synthetic Turf Areas, Sci. Total Environ., 2009, 407(7), 2183–2190 CrossRef CAS.
- A. B. Khudhair, M. Musa, M. S. M. Jaafar and T. Hadibarata, Cresol Red Dye Removal Using Recycled Waste Tire Rubber, Int. J. Engine Res., 2015, 16, 57–63 Search PubMed.
- L. Alamo-Nole, O. Perales-Perez and F. R. Roman, Use of Recycled Tires Crumb Rubber to Remove Organic Contaminants from Aqueous and Gaseous Phases, Desalin. Water Treat., 2012, 49(1–3), 296–306 CrossRef CAS.
- L. A. Alamo-Nole, O. Perales-Perez and F. R. Roman-Velazquez, Sorption Study of Toluene and Xylene in Aqueous Solutions by Recycled Tires Crumb Rubber, J. Hazard. Mater., 2011, 185(1), 107–111 CrossRef CAS PubMed.
- J. Lopez-Morales, O. Perales-Perez and F. Roman-Velazquez, Sorption of Triclosan onto Tyre Crumb Rubber, Adsorpt. Sci. Technol., 2012, 30(10), 831–845 CrossRef CAS.
- R. Acosta, V. Fierro, A. M. de Yuso, D. Nabarlatz and A. Celzard, Tetracycline Adsorption onto Activated Carbons Produced by KOH Activation of Tyre Pyrolysis Char, Chemosphere, 2016, 149, 168–176 CrossRef CAS PubMed.
- A. S. Gunasekara, J. A. Donovan and B. S. Xing, Ground Discarded Tires Remove Naphthalene, Toluene, and Mercury from Water, Chemosphere, 2000, 41(8), 1155–1160 CrossRef CAS.
- I. Acosta-Coley and J. Olivero-Verbel, Microplastic Resin Pellets on an Urban Tropical Beach in Colombia, Environ. Monit. Assess., 2015, 187(7), 435–448 CrossRef.
- F. Lian, F. Huang, W. Chen, B. Xing and L. Zhu, Sorption of Apolar and Polar Organic Contaminants by Waste Tire Rubber and Its Chars in Single- and Bi-Solute Systems, Environ. Pollut., 2011, 159(4), 850–857 CrossRef CAS.
- T. Hüffer, S. Wagner, T. Reemtsma and T. Hofmann, Sorption of Organic Substances to Tire Wear Materials: Similarities and Differences with Other Types of Microplastic, TrAC, Trends Anal. Chem., 2019, 113, 392–401 CrossRef.
-
C. Halsall and E. J. Bouwer, Environmental Organic Chemistry, John Wiley & Sons, Hoboken, U.S., 3rd edn, 1997, vol. 25 Search PubMed.
- S. Brunauer, P. H. Emmett and E. Teller, Adsorption of Gases in Multimolecular
Layers, J. Am. Chem. Soc., 1938, 60(2), 309–319 CrossRef CAS.
- T. Hüffer, A.-K. Weniger and T. Hofmann, Sorption of Organic Compounds by Aged Polystyrene Microplastic Particles, Environ. Pollut., 2018, 236, 218–225 CrossRef PubMed.
- T. Hüffer and T. Hofmann, Sorption of Non-Polar Organic Compounds by Micro-Sized Plastic Particles in Aqueous Solution, Environ. Pollut., 2016, 214, 194–201 CrossRef.
- S. Endo, P. Grathwohl, S. B. Haderlein and T. C. Schmidt, Compound-Specific Factors Influencing Sorption Nonlinearity in Natural Organic Matter, Environ. Sci. Technol., 2008, 42(16), 5897–5903 CrossRef CAS PubMed.
- T. Hüffer, S. Endo, F. Metzelder, S. Schroth and T. C. Schmidt, Prediction of Sorption of Aromatic and Aliphatic Organic Compounds by Carbon Nanotubes Using Poly-Parameter Linear Free-Energy Relationships, Water Res., 2014, 59, 295–303 CrossRef.
- T. Hüffer, X. L. Osorio, M. A. Jochmann, B. Schilling and T. C. Schmidt, Multi-Walled Carbon Nanotubes as Sorptive Material for Solventless in-Tube Microextraction (ITEX2) – A Factorial Design Study, Anal. Bioanal. Chem., 2013, 405(26), 8387–8395 CrossRef PubMed.
- T. Hüffer, M. Kah, T. Hofmann and T. C. Schmidt, How Redox Conditions and Irradiation Affect Sorption of PAHs by Dispersed Fullerenes (NC60), Environ. Sci. Technol., 2013, 47(13), 6935–6942 CrossRef PubMed.
-
H. Motulsky and A. Christopoulos, Fitting Models to Biological Data Using Linear and Nonlinear Regression. A Practical Guide to Curve Fitting, GraphPad Software Inc., San Diego, CA, U.S.A., 2003 Search PubMed.
- M. Kah, X. Zhang, M. T. O. Jonker and T. Hofmann, Measuring and Modeling Adsorption of PAHs to Carbon Nanotubes over a Six Order of Magnitude Wide Concentration Range, Environ. Sci. Technol., 2011, 45(14), 6011–6017 CrossRef CAS.
- C. M. Long, M. A. Nascarella and P. A. Valberg, Carbon Black vs. Black Carbon and Other Airborne Materials Containing Elemental Carbon: Physical and Chemical Distinctions, Environ. Pollut., 2013, 181, 271–286 CrossRef CAS PubMed.
- H. N. Tran, S.-J. You, A. Hosseini-Bandegharaei and H.-P. Chao, Mistakes and Inconsistencies Regarding Adsorption of Contaminants from Aqueous Solutions: A Critical Review, Water Res., 2017, 120, 88–116 CrossRef CAS PubMed.
- K. Yang and B. Xing, Adsorption of Organic Compounds by Carbon Nanomaterials in Aqueous Phase: Polanyi Theory and Its Application, Chem. Rev., 2010, 110(10), 5989–6008 CrossRef CAS PubMed.
-
M. Manes, Activated Carbon Adsorption Fundaments, John Wiley & Sons, New York, 1998 Search PubMed.
- K. Yang, L. Zhu and B. Xing, Adsorption of Polycyclic Aromatic Hydrocarbons by Carbon Nanomaterials, Environ. Sci. Technol., 2006, 40(6), 1855–1861 CrossRef CAS PubMed.
- I. Velzeboer, C. J. A. F. Kwadijk and A. A. Koelmans, Strong Sorption of PCBs to Nanoplastics, Microplastics, Carbon Nanotubes, and Fullerenes, Environ. Sci. Technol., 2014, 48(9), 4869–4876 CrossRef CAS PubMed.
- X. Guo, X. Wang, X. Zhou, X. Kong, S. Tao and B. Xing, Sorption of Four Hydrophobic Organic Compounds by Three Chemically Distinct Polymers: Role of Chemical and Physical Composition, Environ. Sci. Technol., 2012, 46(13), 7252–7259 CrossRef CAS.
- E. L. Teuten, S. J. Rowland, T. S. Galloway and R. C. Thompson, Potential for Plastics to Transport Hydrophobic Contaminants, Environ. Sci. Technol., 2007, 41(22), 7759–7764 CrossRef CAS.
- J. M. Saquing, C. D. Saquing, D. R. U. Knappe and M. A. Barlaz, Impact of Plastics on Fate and Transport of Organic Contaminants in Landfills, Environ. Sci. Technol., 2010, 44(16), 6396–6402 CrossRef CAS.
- S. Endo, P. Grathwohl and T. C. Schmidt, Absorption or Adsorption? Insights from Molecular Probes n-Alkanes and Cycloalkanes into Modes of Sorption by Environmental Solid Matrices, Environ. Sci. Technol., 2008, 42(11), 3989–3995 CrossRef CAS.
- K.-U. Goss, The Air/Surface Adsorption Equilibrium of Organic Compounds Under Ambient Conditions, Crit. Rev. Environ. Sci. Technol., 2004, 34(4), 339–389 CrossRef CAS.
- A. E. Onjia, S. K. Milonjic and L. V. Rajakovic, Inverse Gas Chromatography of Chromia. Part II. Finite Surface Coverage, J. Serb. Chem. Soc., 2002, 67(3), 165–178 CrossRef CAS.
- I. D. Smiciklas, S. K. Milonjic and S. Zec, An Inverse Gas Chromatographic Study of the Adsorption of Alkanes on Hydroxyapatite, J. Mater. Sci., 2000, 35(11), 2825–2828 CrossRef CAS.
- S. Endo, P. Grathwohl, S. B. Haderlein and T. C. Schmidt, Characterization of Sorbent Properties of Soil Organic Matter and Carbonaceous Geosorbents Using N-Alkanes and Cycloalkanes as Molecular Probes, Environ. Sci. Technol., 2009, 43(2), 393–400 CrossRef CAS PubMed.
- I. Hadj Romdhane and R. P. Danner, Solvent Volatilities from Polymer Solutions by Gas-Liquid Chromatography, J. Chem. Eng. Data, 2002, 36(1), 15–20 CrossRef.
- P. A. Elkington and G. Curthoys, Heats of Adsorption on Carbon Black Surfaces, J. Phys. Chem., 2002, 73(7), 2321–2326 CrossRef.
- E. V. Kalaschnikova, A. V. Kiselev, R. S. Petrova and K. D. Shcherbakova, Die Untersuchung von Adsorptionsgleichgewichten Auf Graphitiertem Thermischen Rußmittels Gas-Chromatographie, Chromatographia, 1971, 4(11), 495–500 CrossRef.
- J. J. Pignatello, Y. Lu, E. J. LeBoeuf, W. Huang, J. Song and B. Xing, Nonlinear and Competitive Sorption of Apolar Compounds in Black Carbon-Free Natural Organic Materials, J. Environ. Qual., 2006, 35(4), 1049–1059 CrossRef CAS PubMed.
- J. J. Pignatello, Soil Organic Matter as a Nanoporous Sorbent of Organic Pollutants, Adv. Colloid Interface Sci., 1998, 76–77, 445–467 CrossRef CAS.
Footnote |
† Electronic supplementary information (ESI) available. See DOI: 10.1039/c9em00423h |
|
This journal is © The Royal Society of Chemistry 2020 |
Click here to see how this site uses Cookies. View our privacy policy here.