Inactivation of E. coli, Enterococcus spp., somatic coliphage, and Cryptosporidium parvum in wastewater by peracetic acid (PAA), sodium hypochlorite, and combined PAA-ultraviolet disinfection†
Received
20th September 2019
, Accepted 15th November 2019
First published on 15th November 2019
Abstract
Wastewater disinfection is important to protect human and ecosystem health. Peracetic acid (PAA) could be used as a disinfectant for wastewater and has the advantage of being less toxic to aquatic species and forming fewer known disinfection byproducts than traditionally used chlorine. Assessment of the relative performance of PAA and chlorine at different exposures and seasonal temperatures is still needed to promote utility adoption especially in cold environments and for non-traditional applications such as water reuse. Additionally, the combination of PAA and UV disinfection has the potential to augment inactivation especially against more resistant microorganisms. In this study, the disinfection efficiency of PAA and chlorine was compared for four microorganisms: two fecal indicator bacteria (E. coli and Enterococcus spp.), a virus (somatic coliphage), and a protozoan (Cryptosporidium parvum). Experiments were conducted in authentic secondary wastewater samples to compare PAA and NaOCl disinfection at two C·t values (20 mg min l−1 and 60 mg min l−1) and two temperatures (room temperature and 4 °C), investigate combined PAA plus UV treatment, and evaluate PAA and PAA plus UV disinfection after 10 min and 24 h. PAA and NaOCl disinfection efficiency was comparable for E. coli, Enterococcus spp. and somatic coliphage at a C·t of 60 mg min l−1 (2 mg l−1, 30 min) but not at 20 mg min l−1 (2 mg l−1, 10 min). Neither PAA nor NaOCl disinfection reduced concentrations of Cryptosporidium parvum oocysts spiked into secondary wastewater. At a winter-relevant temperature (4 °C), NaOCl was more effective against E. coli and Enterococcus spp. than PAA regardless of exposure, while both PAA and NaOCl achieved comparable somatic coliphage inactivation. The combined use of PAA and UV treatment appear to have achieved higher log reductions than the use of PAA only for E. coli and somatic coliphage, and of UV only for E. coli. Log reductions after PAA and PAA plus UV treatments continued to increase up to 24 h after exposure which has implications for distribution systems for reclaimed water used for irrigation purposes. The results presented here support the use of PAA as an alternative wastewater disinfectant to chlorine and as a complimentary disinfectant to UV.
Water impact
The ideal wastewater disinfectant must be effective against numerous microorganisms under varying wastewater conditions. Traditionally used chlorine (NaOCl) can be harmful in the environment. Peracetic acid (PAA), an alternative, achieves comparable bacterial and coliphage inactivation under certain conditions. UV can enhance PAA disinfection. Increased inactivation and no bacterial regrowth observed 24 h after PAA disinfection has implications for wastewater reuse applications.
|
1 Introduction
Chemical disinfection is often employed as the final treatment of wastewater to minimize the release of pathogenic microorganisms into the environment. When chemical disinfection is employed, chlorine (added as chlorine gas (Cl2) or sodium hypochlorite solution (NaOCl)) is the most common wastewater disinfection method due to its high efficiency, ease of implementation, and low capital and operational costs.1 However, chlorine exhibits toxicity to aquatic life. Residual chlorine as low as 100–300 μg l−1 has been shown to be toxic to aquatic species.2 In addition, research has demonstrated that chlorine leads to the formation of mutagenic and carcinogenic halogenated disinfection byproducts (DBPs).3–5 Due to these negative effects, alternative disinfection methods are being evaluated.
Peracetic acid (PAA) is an alternative disinfectant that is less toxic to aquatic species and forms much less halogenated disinfection byproducts than chlorine-based disinfectants.3–7 Tightening regulations on residual chlorine concentrations have provided incentives for PAA adoption in some countries such as South Africa, Italy, and the U.S.8–10 Previous research has been conducted in several water types and with several different bacterial targets. In synthetic water, E. coli log reductions by 3 mg l−1 PAA for 10 min (C·t = 30 mg min l−1) and 18 mg l−1 of NaOCl for 10 min (C·t = 180 mg min l−1) were 2.81 and 0.28, respectively.11 In filtered secondary effluent, a PAA C·t of 540 mg min l−1 (15 mg l−1, 36 min) achieved 4 log reductions of E. coli, and total and fecal coliforms while a lower NaOCl C·t of 135 mg min l−1 (7.5 mg l−1, 18 min) achieved higher bacterial log reductions of 4.5–5.5.12 In laboratory experiments with arctic wastewater pretreated with coagulation, 6 and 12 mg l−1 of PAA for 60 min (C·t = 360 and 720 mg min l−1) achieved 2.8 and 3.1 log reductions of heterotrophic bacteria.13 PAA disinfection efficiency has also been investigated at the pilot scale. Pilot scale studies demonstrated 2–3 log reductions of fecal coliforms and E. coli with PAA C·t values of 30–40 mg min l−1,14–17 while Enterococcus spp. inactivation tended to be 1–2 logs lower than total coliforms or E. coli at comparable exposures.18–20 More recently, a pilot study demonstrated different microorganisms were affected differently by increases in PAA concentration versus increases in contact time.21 At a full scale wastewater treatment facility, however, bacterial concentrations before and after PAA exposures of 30–60 mg min l−1 (1–2 mg l−1, 30 min), were not significantly different for fecal coliforms, E. coli, and Enterococcus spp.22
For both PAA and chlorine disinfection, viruses tend to require higher exposures than bacteria to reach comparable levels of inactivation. In secondary effluent, PAA was not able to achieve more than 1 log reduction of MS2 coliphage at exposures of 800 mg min l−1, but was capable of achieving 1–2 log reductions of murine norovirus (MNV) with 20 mg min l−1.23 Using molecular-based techniques, the same research group further demonstrated that PAA (500 mg min l−1) achieved negligible log reductions (<0.5) of human noroviruses group II and MS2 coliphage in secondary effluent, but 1 log reduction of human noroviruses group I was achieved by just 100 mg min l−1 of PAA.24 Protozoa are even less susceptible to PAA and chlorine than viruses or bacteria. In secondary effluent, 120 mg min l−1 of PAA and NaOCl achieved 0.60 and 0.50 log reductions, respectively, of autochthonous Cryptosporidium parvum.25 Protozoa are less studied and should be considered in experiments comparing PAA and NaOCl inactivation of fecal indicator bacteria, viruses, and protozoa in authentic wastewater matrices.
The combined use of a chemical disinfectant such as PAA with UV irradiation has the potential to augment disinfection through multiple mechanisms such as direct nucleic acid damage and the formation of additional free radicals resulting in oxidative stress.26 Previous research showed the potential positive effects of the combined use of PAA and UV in synthetic and authentic wastewater.11,27,28 Sequential application of PAA (5–18 mg min l−1) followed by UV (10.0–162 mJ cm−2) achieved 2–4 bacterial log reductions higher than PAA alone.11,26 Similarly, the combined treatment of PAA and UV can achieve greater coliphage inactivation than the solitary use of either disinfectant. In wastewater, 30 mg min l−1 of PAA (3 mg l−1, 10 min) followed by UV (109–187 mJ cm−2) achieved greater than 2.5 log reductions of coliphages on contrast to the 0.4–0.6 log reductions by PAA alone.27 In authentic secondary effluent, PAA and UV (1.5 mg l−1, 30 min, 20 mJ cm−2) achieved at least twice the log reductions of MS2 coliphage and murine norovirus than by PAA alone, with murine norovirus exhibiting greater susceptibility to disinfection.29 The order in which PAA and UV are applied may influence the disinfection effectiveness. One study found that the simultaneous application of PAA and UV achieved greater inactivation of total coliforms and fecal coliforms than the sequential application of UV followed by PAA.30 The potential synergistic effect of PAA and UV on protozoa is not known.
Previous research suggests that the wastewater matrix can have significant impacts on the efficacy of different disinfectants since sources and treatment stages of wastewater may contain different constituents that affect the disinfectant's efficiency.18,29,31,32 For example, in primary, secondary, and tertiary effluent, 65 mg min l−1 of PAA (5 mg l−1, 13 min), achieved 0.7, 2.7, and 3.0 log reductions of total coliforms, respectively.18 In comparison to chlorine, PAA disinfection is less susceptible to increases in suspended particle sizes.33 Chlorine's disinfection efficiency is known to drop with decreasing temperature.34 The PAA disinfection performance at cold temperatures is yet to be studied. Future research should include samples from multiple treatment utilities to account for variability in wastewater characteristics and their effect on disinfection performance of PAA.
Research using authentic wastewater with autochthonous microorganisms is needed to evaluate PAA's efficiency across a range of disinfection conditions. Previous studies show that PAA inactivation varies by microorganisms and the physical–chemical wastewater characteristics. Few studies have investigated the effectiveness of PAA against protozoa despite their importance as etiological agents. The present study compares disinfection using PAA, NaOCl, and PAA combined with UV in secondary wastewater collected from four utilities in Western New York State. The research objectives are: (1) to compare the disinfection efficiency of PAA and NaOCl at different C·t values and seasonal temperatures (simulated by room temperature and 4 °C refrigeration) in authentic secondary treated wastewater, and (2) to investigate if combined PAA and UV treatment achieves higher microbial inactivation than PAA only and UV only disinfection at a contact time relevant to wastewater treatment facilities (10 min) as well as a longer contact time (24 h), with potential relevance for wastewater storage and reuse for irrigation purposes. Disinfection experiments used E. coli, Enterococcus spp., and somatic coliphage found in authentic wastewater and Cryptosporidium parvum oocysts spiked into authentic wastewater. Results from this research enhance our understanding of PAA efficacy on a variety of microorganisms at different temperatures, exposures, and disinfection scenarios. This information is needed to inform the use of PAA as an alternative disinfectant for wastewater and water reuse applications.
2 Materials and methods
2.1 Wastewater sample collection
Secondary effluent samples were collected from four (A, B, C, D) wastewater treatment plants (WWTP) in Western New York. Samples were collected in HDPE bottles pre-sterilized in 10% hydrochloric acid. All samples were collected between 09:00–10:00 am and stored on ice during transportation back to the laboratory at University at Buffalo. Disinfection experiments at room temperature were conducted within 2–6 h of collection. Table 1 summarizes the size and treatment processes for each treatment plant. All samples were collected between August 3, 2016 and March 14, 2018. All treatment plants practice chlorine disinfection with WWTP A and D disinfecting only during summers (May 15th – October 15th). Disinfection experiments were conducted with grab samples from at least two of the facilities for each treatment scenario and microorganism. The date and WWTP for the grab sample for each set of experiments is shown in Table SI 1.†
Table 1 Sampling dates at each utility along with the size of utilities and their respective treatment processes prior to disinfection
WWTP |
Size (MGD) |
Treatment process |
Samples from this treatment plant were collected from the clarifier post aeration for nitrification.
|
A |
36 |
Screening, grit removal, primary settling, secondary treatment (aeration for BOD removal, settling, aeration for nitrification, settling)a |
B |
16 |
Screening, secondary aeration, secondary clarification, rapid sand filtration |
C |
5 |
Screening, grit removal, primary settling, secondary aeration, secondary clarification with addition of alum to remove phosphorus |
D |
3 |
Screening, grit removal, secondary aeration, rapid sand filtration |
2.2 Wastewater quality analysis
Total suspended solids (TSS), pH, oxidant demand (OD), turbidity, and UV absorbance at 254 nm (UV254) were monitored for each sample. TSS was measured according to Method 2540.35 The pH of samples was measured using a Mettler Toledo pH meter (SevenCompact pH/Ion S220, Switzerland). In this study, OD was defined as the difference between the residual oxidant concentrations at 0.5 min and at 5 min. PAA and NaOCl residual concentrations were quantified based on the colorimetric Method 4500 G35 using N,N-diethyl-p-phenylenediamine total chlorine reagent (2105669, HACH, CO, USA). NaOCl and PAA were allowed a reaction time of 3 min and 1 min, respectively, during the colorimetric reaction.36 Turbidity was measured using a HACH 2100Q portable turbidimeter (2100Q01, HACH, CO, USA). UV254 was measured using a Cary 60 instrument (Agilent Technologies, CA, USA). The UV absorbance by a sample was used as a proxy for the total organic carbon (TOC) in the sample.37
2.3 Disinfection experiments
2.3.1 Peracetic acid (PAA) and sodium hypochlorite (NaOCl) disinfection.
PAA and NaOCl disinfection experiments were conducted at room temperature for all microorganisms and at 4 °C for E. coli, Enterococcus spp., and somatic coliphage. PAA (SIGMA Aldrich, MO, USA) and NaOCl (ACROS Organics, Belgium) were used as purchased. The concentrations of PAA and NaOCl in the respective commercial stock solutions were determined by Iodometric Method 4500.35 The PAA and NaOCl concentrations in the commercial stock solutions were 38 ± 0.05% and 5 ± 0.22%, respectively. The PAA stock solution also contained 6% H2O2.
The experimental design is shown in Fig. 1. All bacteria and phage inactivation experiments were conducted in triplicate and relied on autochthonous microorganisms to ensure field-relevant results. We decided not to spike lab cultivated bacteria and viruses since previous research has shown that laboratory strains of fecal indicator bacteria (FIB) are more susceptible to treatments such as sunlight exposure than environmental strains are.38Cryptosporidium parvum oocysts were not found in high enough concentrations in wastewater for detection and hence were spiked into wastewater samples at an initial concentration of 100 oocysts per ml. Cryptosporidium parvum inactivation experiments were conducted using samples from WWTP A and B and technical duplicates were sampled from each microcosm for enumeration.
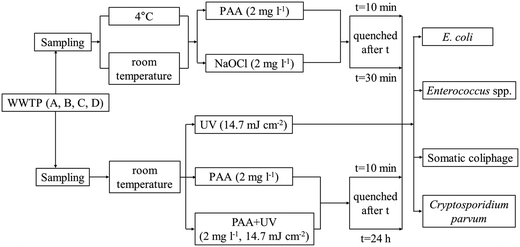 |
| Fig. 1 Overview of experimental design. Cryptosporidium parvum oocysts were spiked into wastewater samples. The 2 mg l−1 concentrations added of PAA and NaOCl correspond to molar concentrations of 26 and 28 μM, respectively. | |
In the experiments comparing PAA and chlorine disinfection, 300 ml of secondary treated wastewater was placed in 600 ml autoclaved glass beakers (diameter 9 cm, No. 14005, Kimax Kimble) and kept in the dark. The disinfectant (PAA or NaOCl) was added to the beaker at a concentration of 2 mg l−1 (the corresponding molar concentrations of PAA and NaOCl are 26 and 28 μM, respectively), and two contact times, 10 and 30 min, were evaluated. The shorter contact time (10 min) was tested because the most significant microbial reductions by PAA were shown to occur within the first 15 min of contact,28 and it is relevant for the lower range of contact times in typical wastewater treatment plants; while the longer contact time (30 min) represents the upper range of possible contact times.
After the 10 or 30 min contact time, PAA or chlorine residual was quenched immediately with sodium thiosulfate pentahydrate (2085-0010, ACROS) at a 0.44 mM (i.e., 10.7- and 10.5-fold molar excess to the applied PAA and NaOCl concentration, respectively). At the applied concentration, we did not observe any effects of sodium thiosulfate pentahydrate itself on bacterial concentrations in wastewater. Microorganisms were enumerated immediately after quenching, and compared with the microbial concentrations before disinfection to calculate log (base 10) reductions according to eqn (1):
| 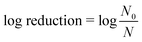 | (1) |
where
N
0 = initial microbial concentration, CFU/100 ml or PFU/100 ml
N = microbial concentration after disinfection, CFU/100 ml or PFU/100 ml
All disinfection experiments comparing PAA and NaOCl at room temperature were done in their own grab samples from at least 2 different utilities (WWTP B, C, and D for bacterial inactivation, all WWTP for somatic coliphage inactivation, and WWTP A and B for Cryptosporidium parvum inactivation). For experiments conducted at 4 °C, samples were stored in a 4 °C refrigerator for at least 12 h before enumeration of initial microbial concentrations and during disinfection treatments.
2.3.2 Ultraviolet (UV) and combined PAA + UV disinfection.
All experiments involving UV were conducted at room temperature. UV irradiation was conducted in bench-scale semi-collimated beam reactor. An autoclaved crystallization dish (diameter 10 cm, depth 5 cm, No. 3140, Pyrex) containing 200 ml of secondary effluent sample (depth 2.7 cm) was placed directly under the UV lamp and stirred continuously by a magnetic stir bar to ensure mixing. The fluence rate of the UV reactor was 0.307 mW cm−2 as determined by iodide-iodate chemical actinometry.39,40 The incident UV fluence used in all disinfection experiments was 14.7 mJ cm−2, which required 48 s irradiation time. This UV fluence is on the low end of typical wastewater disinfection exposure, and was selected for relevance for the treatment plants in this region. Microbial concentration was enumerated immediately after UV irradiation.
For the combined PAA and UV experiments (hereafter PAA + UV), samples were exposed to PAA at a concentration of 2 mg l−1 for 10 min prior to UV irradiation. PAA residual was quenched immediately after UV irradiation by sodium thiosulfate pentahydrate as described above, and the microbial concentration was enumerated. These PAA + UV experiments with a 10 min PAA contact time were conducted alongside UV only experiments (Table SI 1†). Experiments were also conducted to investigate the effect of a longer PAA contact time (microbial enumeration at 24 h) and the potential for microbial regrowth after treatment with PAA, UV or PAA + UV. Experiments with PAA only, UV only and PAA + UV were conducted, sodium thiosulfate pentahydrate was added at 24 h followed by immediate enumeration. The specific conditions tested in this set of experiments were (1) UV only (14.7 mJ cm−2) (hereafter UV24), (2) PAA (2 mg l−1) quenched 24 h after the addition of PAA (hereafter PAA24), and (3) PAA (2 mg l−1, 10 min) followed by UV (14.7 mJ cm−2) quenched 24 h after the addition of PAA (hereafter PAA24 + UV).
To determine the effect of the sequential order of PAA and UV exposure on microbial inactivation, experiments were conducted using WWTP A secondary effluent to compare log reductions of E. coli and Enterococcus spp. between (1) PAA (2 mg l−1, 10 min) followed by UV (14.7 mJ cm−2) and (2) UV (14.7 mJ cm−2) followed by PAA (2 mg l−1, 10 min) (hereafter UV + PAA). PAA residual was quenched after PAA or UV treatment, which ever was applied last, followed by the enumeration of microbial concentrations.
2.4 Microbial enumeration
Microorganisms were enumerated before and after the disinfection treatment for each microcosm experiment. Method 1603 and Method 1600 were used to provide direct counts of E. coli and Enterococcus spp. bacteria, respectively.41,42 Briefly, diluted and undiluted samples were filtered through 0.45 μm mixed cellulose ester filters (Millipore, CA, USA) and placed on mTEC selective media to enumerate E. coli and on mEI to enumerate Enterococcus spp. mTEC plates were incubated for 2 h at 35.5 ± 0.5 °C and then for 22 h at 44.5 ± 0.5 °C. mEI plates were incubated for 24 h at 41.0 ± 0.5 °C. When possible, final bacterial concentrations were based on plates with colony counts between 10–200 colony forming units (CFU). The detection limit for bacterial counts was 2–25 CFU/100 ml, depending on the volume of water filtered.
Somatic coliphage was enumerated according to Method 1602, single agar layer procedure.43 Briefly, 2.5 ml host culture of E. coli CN-13 (ATCC# 700609), grown to stationary phase (7 hours at 37 °C), were added to 25 ml of sampled wastewater followed by the addition of double strength (2×) tryptic soy broth (Difco, MD, USA). The 50 ml mixture was distributed into three plastic petri dishes (100 × 15 mm, Fisherbrand) and incubated for 16–24 h at 37.0 ± 0.5 °C. Plaques represented by zones of bacterial host lawn clearings in the three dishes were counted as somatic coliphage plaque forming units (PFU) and the concentration for a sample was the sum of PFUs on the three plates where each plate had plaque counts between 1–200 plaque forming units. The detection limit for somatic coliphage counts was 4 PFU/100 ml.
Cryptosporidium parvum oocysts were spiked into the secondary wastewater from 2 utilities (WWTP A and B). Oocysts were attained at a stock concentration of 2.5 × 105 oocysts per ml (P102C, Waterborne Inc.). Wastewater samples were spiked with 10
000 oocysts per 100 ml. Cryptosporidium parvum oocysts were enumerated in treated samples according to EPA Method 1623.1 (ref. 44) without the initial filtration step since experiments were spiked with a high enough concentration. Method 1623.1 uses immunomagnetic separation (IMS) and microscopy to enumerate oocysts. Briefly, duplicate aliquots (5 and 25 ml for samples from WWTP A, and 5 and 10 ml or 5 and 15 ml for samples from WWTP B) of disinfected wastewater samples were collected and centrifuged at 1500 × g for 30 min to pellet the oocysts. IMS was carried out using the Dynabeads Cryptosporidium Kit (73011, IDEXX). The separated oocysts were stained using the Crypta-a-Glo Fluorescein Comprehensive Kit (A400FLK, Waterborne), after which the fluorescein isothiocyanate-conjugated antibodies combined with the oocysts would emit green fluorescence under UV light. The oocysts were then counted at 400X magnification using a Zeiss microscope (ID# M208571, Carl Zeiss MicroImaging). To confirm Cryptosporidium parvum oocysts by microscopy in the wastewater matrix, all samples were compared to a positive slide made with Cryptosporidium parvum oocysts spiked into deionized water. Cryptosporidium parvum was characterized as oocysts with an intact circular shape, green in color and without any atypical structures such as pores, appendages, or spikes (Fig. SI 1 and SI 2†). Cryptosporidium parvum concentrations were reported as the number of oocysts per milliliter of sample (oocysts per ml). When possible, final oocyst concentrations were based on well slides with counts between 20–100 oocysts. The recovery of oocysts in the spiked secondary wastewater was 16.5 ± 5.9%, which is consistent with a previous study in wastewater.25 The detection limit for Cryptosporidium parvum counts was 10 oocysts per 100 ml. The concentration Cryptosporidium parvum oocysts in 25 ml of the wastewater sample before spiking was tested once for each WWTP and was always below the detection limit.
2.5 Data analysis
The disinfection efficacy was assessed based on log reductions. For figures and tables, the average log reduction and the associated error are shown. The propagated error associated with the average log reduction was calculated according to methods described previously.45 Statistical analyses were performed in R.46 ANOVAs followed by Tukey's Honest Significance Difference (HSD) were used to test whether the log reductions for a given secondary effluent sample were the same between (1) PAA and NaOCl at different C·t values (accounting for WWTP and temperature), (2) PAA and NaOCl at different temperatures (accounting for C·t and WWTP), (3) UV, PAA + UV, PAA24, UV24 and PAA24 + UV. Homogeneity of variance and normality were visually verified. A paired t-test was used to test whether there was a difference between log reductions when PAA treatment was followed by UV versus UV treatment followed by PAA (PAA + UV and UV + PAA). Statistical significance was set at an alpha value of 0.05 for all tests. WWTPs were sampled multiple times throughout the study, however, each sample collection at a WWTP was treated as an individual grab sample and used for one set of experiments for a given treatment (Table SI 1†).
The detection limit for bacteria, somatic coliphage and Cryptosporidium parvum oocysts was 2–25 CFU/100 ml, 4 PFU/100 ml and 10 oocysts per 100 ml, respectively. When the concentration in all 3 microcosms of a given treatment was below the detection limit, one-half the detection limit was substituted in for the 3 microcosms. If the concentration in at least one microcosm of a given treatment was above the detection limit, no substitutions were made for the microcosms below the detection limit (Text SI 1†). The propagated error was calculated only when all 3 microcosms were available.
All PAA and UV treatment experiments were analyzed to determine if UV enhanced PAA disinfection. Previous research defined that there was synergy between PAA and UV disinfection if the log reductions by the combined PAA and UV treatment were greater than the sum of the of the individual log reductions by UV only and PAA only.11,27,29 In this paper, however, we report a positive effect of combined PAA and UV treatment if the log reductions by the combined PAA + UV treatment are greater than those by UV only and PAA only.
3 Results
3.1 Comparing PAA and NaOCl disinfection
The initial concentrations in the secondary effluents were on the order of 103–105 CFU/100 ml for E. coli, 102–104 CFU/100 ml for Enterococcus spp., and 102–104 PFU/100 ml for somatic coliphage. For both PAA and NaOCl disinfection, E. coli was the most susceptible indicator organism, followed by Enterococcus spp. and then somatic coliphage (Table SI 2†). Cryptosporidium parvum oocysts exhibited minimal susceptibility to NaOCl and PAA disinfection (Table 2).
Table 2
Cryptosporidium parvum oocyst concentrations (oocysts per 100 ml) before (Ci) and after (Cf) disinfection treatments of samples from WWTP A and B. A semicolon separates the technical duplicates results. NA denotes unreadable well slides under microscope. Treatment time refers to the time between the applied treatment and enumeration (for the shorter times, treatment time is likely equivalent to exposure time. However, for longer times, the disinfectant may have decayed, and the treatment time is not equivalent to exposure time)
Treatment time |
Disinfection treatment |
WWTP A (oocysts per 100 ml) |
WWTP B (oocysts per 100 ml) |
C
i
|
C
f
|
C
i
|
C
f
|
10 min |
NaOCl |
1120; 1488 |
460; 1176 |
1720 |
1680; 1160 |
|
PAA |
1120; 1489 |
1240; 1008 |
1720 |
1710; NA |
|
UV |
1300; 1564 |
1000; 572 |
2440 |
1640; 1150 |
|
PAA + UV |
1300; 1564 |
1560; 1084 |
2440 |
1080; 930 |
30 min |
NaOCl |
1120; 1489 |
260; 812 |
1720 |
1960; 1480 |
|
PAA |
1120; 1489 |
2000; 1028 |
1720 |
1980; NA |
24 h |
PAA |
1300; 1564 |
860; 824 |
2440 |
1420; 1950 |
|
UV |
1300; 1564 |
600; 220 |
2440 |
2220; 2670 |
|
PAA + UV |
1300; 1564 |
540; 284 |
2440 |
2800; 1350 |
3.1.1 PAA and NaOCl disinfection at two C·t values (2 mg l−1 for 10 or 30 min).
Fig. 2 shows a comparison of PAA and NaOCl inactivation of E. coli, Enterococcus spp., and somatic coliphage at room temperature and at two different C·t values (specifically two different contact times). At 20 mg min l−1C·t (2 mg l−1, 10 min), NaOCl and PAA achieved comparable inactivation of E. coli and somatic coliphage, but NaOCl achieved significantly higher inactivation of Enterococcus spp. than PAA (ANOVA, p = 2 × 10−3). At 60 mg min l−1C·t (2 mg l−1, 30 min), PAA and NaOCl disinfection was comparable for all three microorganisms. Increasing contact time resulted in a significantly higher log reduction by both PAA and NaOCl for Enterococcus spp. (ANOVA, p < 0.05) but not for E. coli and somatic coliphage (ANOVA, p > 0.05). Table 2 shows in a few cases in which NaOCl and PAA inactivated Cryptosporidium parvum oocysts, specifically NaOCl treatment for a contact time of 30 minutes reduced oocyst concentrations for WWTP A and PAA treatment resulted in reduced oocyst concentrations when enumerated at 24 h.
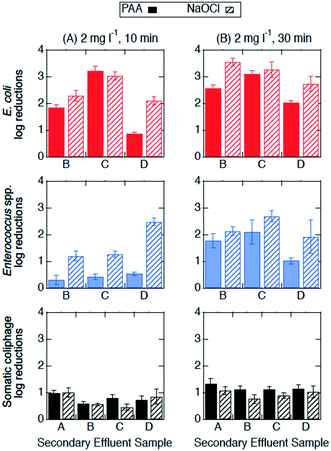 |
| Fig. 2 Log reductions of E. coli (red, top row), Enterococcus spp. (blue, middle row), and somatic coliphage (black, bottom row) at room temperature by PAA (solid bars) and NaOCl (bars with diagonal stripes) at (A) 2 mg l−1, 10 min (n = 3) and (B) 2 mg l−1, 30 min (n = 3) in secondary effluent samples from different utilities (x-axis). Error bars represent the propagated error of triplicate experiments. All log reductions are shown in Table SI 2.† | |
3.1.2 Effect of temperature on disinfectant performance.
Overall, log reductions at 4 °C were similar to or lower than at room temperature for both PAA and NaOCl disinfection (Fig. 3). Similar to results from the experiments at room temperature, NaOCl and PAA disinfection at 4 °C resulted in comparable log reductions for somatic coliphage regardless of the C·t value; and NaOCl achieved significantly greater inactivation of E. coli and Enterococcus spp. than PAA at both the C·t values tested at 4 °C (ANOVA p < 0.05). Only PAA C·t influenced disinfection performance at 4 °C for the inactivation of bacteria. PAA disinfection achieved significantly greater log reductions of both E. coli and Enterococcus spp. at the longer contact time tested (30 minutes) (ANOVA, p < 0.05), while the longer contact time did not increase log reductions by NaOCl disinfection. These trends are different from those observed at room temperature in two ways. First, increases in contact time enhanced PAA disinfection of only Enterococcus spp. at room temperature, and both Enterococcus spp. and E. coli at 4 °C. Second, while increases in contact time enhanced the NaOCl disinfection of Enterococcus spp. at room temperature, no significant difference in NaOCl disinfection of Enterococcus spp. at 4 °C was observed for either contact time.
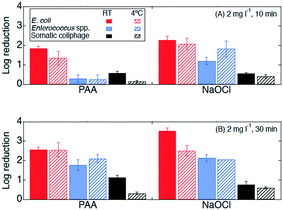 |
| Fig. 3 Log reductions of E. coli (red, first bar), Enterococcus spp. (blue, third bar), and somatic coliphage (black, fifth bar) at room temperature (RT, in solid bars) and 4 °C (in bars with diagonal stripes) by PAA and NaOCl with exposure of (A) 20 mg min l−1C·t (2 mg l−1, 10 min) and (B) 60 mg min l−1C·t (2 mg l−1, 30 min) in the secondary effluent samples from WWTP B. Error bars represent the propagated error of triplicate experiments. For experiments with only one or two quantifiable log reductions, the single log reduction or average of two is plotted with no error bars. All log reductions are shown in Table SI 2.† | |
3.2 Combined PAA and UV disinfection
3.2.1 PAA, UV, and PAA + UV treatment.
The log reductions achieved by UV (14.7 mJ cm−2), PAA (2 mg l−1, 10 min), and PAA + UV (PAA 2 mg l−1, 10 min prior to 14.7 mJ cm−2 UV) treatment for E coli, Enterococcus spp., and somatic coliphage are shown in Fig. 4. The log reductions previously achieved by PAA alone were 0.87–3.2, 0.30–0.53, and 0.6–1.0 for E. coli, Enterococcus spp., and somatic coliphage, respectively (Fig. 2(A) and Table SI 2†). In comparison to UV only, PAA + UV achieved significantly higher log reductions for E. coli (ANOVA, p = 1 × 10−26) but not for Enterococcus spp. and somatic coliphage. E. coli, Enterococcus spp. and somatic coliphage log reductions by PAA only were measured in a secondary effluent sample collected on a different day than the sample used for UV only and PAA + UV experiments. Since the secondary effluent samples were collected on different days and potentially had different compositions, we considered the samples to be different and therefore no statistical analyses were conducted to compare PAA only with either UV only or PAA + UV. However, UV only appeared to achieve lower log reductions than PAA of E. coli (1.4–1.5), higher log reductions of Enterococcus spp. (1.1–1.2) and of somatic coliphage (1.1–2.4). PAA + UV as a combined treatment appeared to achieve higher log reductions than PAA only for the inactivation of E. coli (3.1–3.6), Enterococcus spp. (1.3–1.6), and somatic coliphage (1.3–2.7). Minimal inactivation of Cryptosporidium parvum oocysts was observed for PAA only, UV only and PAA + UV treatments (Table 2).
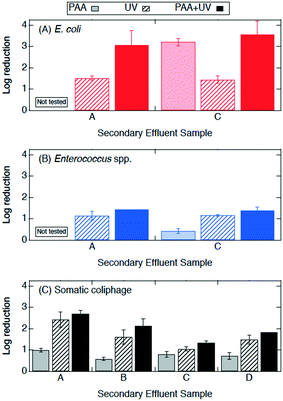 |
| Fig. 4 Log reductions of (A) E. coli, (B) Enterococcus spp., and (C) somatic coliphage by secondary effluent sample (utility) after treatment with PAA (2 mg l−1 for 10 min) in light bars, UV (14.7 mJ cm−2) in bars with diagonal stripes, and PAA + UV (PAA 2 mg l−1 for 10 min prior to 14.7 mJ cm−2 UV) in solid bars. E. coli, Enterococcus spp., and somatic coliphage log reductions by PAA are plotted here for a visual comparison only as they were from different secondary effluent grab samples than those used during the UV only and PAA + UV treatments. Error bars represent the propagated error of triplicate experiments. For experiments with only one or two quantifiable log reductions, the single log reduction or average of two is plotted with no error bars. All log reductions are shown in Table SI 3.† | |
Experiments were conducted to determine if bacterial inactivation differed when PAA (2 mg l−1, 10 min) was applied before UV (PAA + UV) or after UV (UV + PAA). No significant difference was observed between these two treatments for the inactivation of E. coli and of Enterococcus spp. (paired t-test, p > 0.05) (Fig. SI 3†). These results suggest that the sequential order of PAA and UV did not affect bacterial inactivation.
3.2.2 PAA, UV and combined PAA plus UV treatment with microbial enumeration at 24 h.
Experiments were conducted to investigate the effect of PAA, UV and PAA + UV treatment on longer time scales relevant for water reuse applications. Twenty-four hours after treatment with PAA, UV, or PAA + UV, samples were quenched with sodium thiosulfate (to quench any remaining PAA) and microorganisms were immediately enumerated. All log reductions are shown in Fig. 5 and Table SI 3.† Compared to PAA24, PAA24 + UV log reductions were significantly higher for somatic coliphage (ANOVA, p = 0.02), but not for E. coli and Enterococcus spp. Compared to UV24, PAA24 + UV achieved significantly higher log reductions for E. coli, Enterococcus spp., and somatic coliphage (ANOVA, p < 0.05). Comparing the effects of PAA24 and UV24, PAA24 achieved significantly higher log reductions for E. coli and Enterococcus spp. (ANOVA, p < 0.05), but not for somatic coliphage. Overall, both PAA plus UV (PAA24 + UV, 2 mg l−1; 14.7 mJ cm−2) and PAA only (PAA24, 2 mg l−1) achieved higher bacterial log reductions than UV only (UV24, 14.7 mJ cm−2), while only the combined treatment achieved higher somatic coliphage log reductions than UV only. Minimal inactivation of Cryptosporidium parvum oocysts was observed for all three treatments (Table 2).
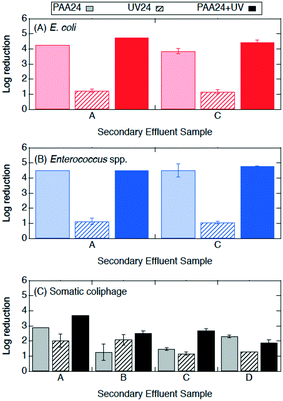 |
| Fig. 5 Log reductions of (A) E. coli, (B) Enterococcus spp., and (C) somatic coliphage by secondary effluent sample (utility) after treatment with PAA24 (2 mg l−1 initial concentration) in light bars, UV24 (14.7 mJ cm−2) in bars with diagonal stripes, and PAA24 + UV (2 mg l−1 for 10 min prior to 14.7 mJ cm−2 UV) in solid bars. PAA was quenched and microorganisms were enumerated at 24 h. Error bars represent the propagated error of triplicate experiments. For experiments with only one or two quantifiable log reductions, the single log reduction or average of two is plotted with no error bars. All log reductions are shown in Table SI 3.† | |
Log reductions 10 min and 24 h after PAA, UV, and PAA + UV treatments were compared to determine the effect of the longer contact time. The log reductions of E. coli, Enterococcus spp. and somatic coliphage by PAA appear to be higher by a factor of at least 1.2, 8.5, and 1.3, respectively, when they were enumerated at 24 h than when they were enumerated at 10 min. Log reductions for PAA plus UV were significantly higher when enumerated at 24 h than after 10 min for Enterococcus spp. (ANOVA, p = 1 × 10−26), but comparable for both E. coli and somatic coliphage. No significant differences in bacterial and coliphage log reductions were observed for UV treatment when enumerated after 10 min and at 24 h. These results suggest that extended contact times may enhance PAA disinfection of bacteria, particularly Enterococcus spp., and somatic coliphage. For the PAA24, UV24 and PAA24 + UV experiments, no bacterial regrowth was observed when bacteria were enumerated after 24 h.
3.3 Wastewater characteristics
Several physical–chemical characteristics of the secondary effluents were measured to investigate how these characteristics influence inactivation (Table 3). Most of the physical–chemical characteristics showed little variability between secondary effluents from different utilities. Two variables, total suspended solids (TSS) and turbidity, varied between secondary effluents and sample collection date. However, no trends were observed between log reductions and either TSS or turbidity, and no statistical tests were run given the limited sample size.
Table 3 Wastewater characteristics measured for each utility on each sampling date. The following parameters were measured: total suspended solids (TSS) (mg l−1), pH, oxidant demand for NaOCl (NaOCl OD) (mg l−1), oxidant demand for PAA (PAA OD) (mg l−1), absorbance at 254 nm, turbidity (NTU). The average and standard deviation for a particular parameter is included if it was measured at least three times for a given utility. An asterisk (*) indicates that the wastewater parameter was only measured once or twice for the given utility. A dash (−) indicates that the parameter was not measured
WWTP |
TSS (mg l−1; n = 5–9) |
pH (n = 5–11) |
PAA OD (mg l−1; n = 2–6) |
NaOCl OD (mg l−1; n = 1–5) |
UV254 (n = 5–11) |
Turbidity (NTU; n = 5–6) |
A |
20.00 ± 12.01 |
6.69 ± 0.14 |
0.07 ± 0.02 |
0.24 + 0.22 |
0.17 ± 0.04 |
6.06 ± 5.42 |
B |
16.34 ± 14.04 |
6.88 ± 0.22 |
0.11 ± 0.04 |
0.11 + 0.08 |
0.19 ± 0.09 |
6.31 ± 1.06 |
C |
23.79 ± 20.49 |
7.11 ± 0.29 |
0.10 ± 0.03 |
0.1*, 0.08* |
0.23 ± 0.11 |
— |
D |
18.75 ± 13.69 |
7.17 ± 0.64 |
0.060*, 0.41* |
0.40* |
0.13 ± 0.07 |
— |
4 Discussion
4.1 Comparing PAA and NaOCl disinfection
The effectiveness of PAA and NaOCl as wastewater disinfectants is dependent on the microorganism being targeted and the applied exposure. Overall, our results for both PAA and NaOCl show the susceptibility of microorganisms from greatest to least being E. coli > Enterococcus spp. > somatic coliphage > Cryptosporidium parvum, and increasing C·t, specifically contact time, results in more inactivation. PAA disinfection was comparable to NaOCl in all cases except for the inactivation of Enterococcus spp. at the lower exposure of 20 mg min l−1 (2 mg l−1, 10 min). Our results are consistent with previous research. Other studies showed that PAA and NaOCl have similar log reductions against fecal and total coliforms, while coliphages are more resistive to disinfectants and are more effectively inactivated by NaOCl than by PAA.9,33,47,48 Veschetti et al.48 found that PAA and NaOCl disinfection resulted in comparable inactivation of fecal coliforms and E. coli, but PAA required 7 times the initial concentration of NaOCl to achieve a 1 log reduction of bacteriophages. In our study, somatic coliphage log reductions by NaOCl varied from log reductions by PAA by less than a factor of 1.2 at an exposure of 20 mg min l−1 and a factor of 0.9 at an exposure of 60 mg min l−1. Similar to another study investigating inactivation of Cryptosporidium parvum in wastewater, we observed a reduction in oocysts after a contact time of 30 min (C·t = 60 mg min l−1) of NaOCl.25 Unlike Briancesco et al.25 however, we did not observe reductions in oocyst concentrations after a PAA contact time of 30 min (C·t = 60 mg min l−1).
At a winter-relevant temperature (4 °C), PAA and NaOCl disinfection were not comparable at disinfecting bacteria, with NaOCl achieving higher log reductions than PAA for E. coli and Enterococcus spp. Previous studies have shown that PAA and NaOCl were less effective at colder temperatures.34,49 For PAA disinfection, a study investigating the effect of temperature found that the inactivation of Enterococcus spp. dropped by 1 log for every 10 °C temperature drop.49 Understanding the effect of temperature is particularly important in regions with large temperature changes between seasons and large volumes of water due to winter storms and snow melt. Interestingly, we observed that increasing contact time (10 to 30 min) had different effects on the performance of the two disinfectants at room temperature from that at 4 °C. At room temperature, the increase in contact time enhanced Enterococcus spp. inactivation by both PAA and NaOCl, while the increase in contact time at 4 °C enhanced Enterococcus spp. inactivation by PAA only. In addition, the increase in contact time at 4 °C enhanced PAA (but not NaOCl) disinfection of E. coli while no significant differences in E. coli inactivation were observed between 10 and 30 min at room temperature for either disinfectant. Reasons for these differences could include decreases in predation due to colder temperatures, changes in bacterial growth rates, and bacteria entering a viable but nonculturable (VBNC) state.34,50–52 Another possible explanation for differences observed between the disinfectants could be changes in disinfectant decay kinetics due to temperature. While the results presented in this paper support the use of PAA as an alternative disinfectant, utilities considering switching from NaOCl to PAA need to consider their target microorganism, the feasibility of the required exposure for the disinfectant-microorganism combination, and seasonal temperatures.
Neither exposure nor temperature produced significant changes in somatic coliphage inactivation by either disinfectant. For the inactivation of somatic coliphage, PAA and NaOCl were comparable, achieving a maximum of 1.3 and 1.1 log reductions, respectively, for a contact time of 30 min. Previous studies using synthetic water achieved 1.0–1.3 log reductions of MS2 coliphage by PAA (3 mg l−1, 10 min, C·t = 30 mg min l−1) and NaOCl (12 mg l−1, 10 min, C·t = 120 mg min l−1), and only 0.4 log reductions in secondary effluent treated with PAA 3 mg l−1 for 13 min (C·t = 39 mg min l−1).11,18 Another study showed that PAA (1.5 mg l−1, 30 min, C·t = 45 mg min l−1) achieved negligible MS2 coliphage log reductions (<0.5) but over 1.5 log reductions of murine norovirus in secondary effluent.29 MS2 coliphage resistance has also been demonstrated at extreme PAA and monochloramine exposures (800 mg min l−1) in secondary effluent, however, 40 mg min l−1 achieved over 2 log reductions of murine norovirus.23 These results suggest that the effectiveness of PAA as a disinfectant for viruses is variable and could be virus specific. Given these results, utilities should be cautious choosing a PAA exposure based on the results presented herein unless somatic coliphage is used for regulatory purposes. Viruses in authentic secondary effluent may also be attached to particles that protect them from disinfectants which could contribute to lower inactivation of viruses seen in this study compared to studies with spiked viruses in synthetic waters.53
Cryptosporidium parvum was the least susceptible microorganism to both PAA and NaOCl in the present study. Minimal inactivation of Cryptosporidium parvum oocysts was observed after NaOCl treatment (2 mg l−1 for 30 min) and PAA treatment and enumeration at 24 h. Previous research has shown that Cryptosporidium parvum is difficult to remove by traditional treatments such as chlorine disinfection or rapid sand filtration.54 A study found that 60–120 mg min l−1 of PAA removed 71–75% or approximately 0.57 log reduction of Cryptosporidium oocysts in secondary effluent, which is more than we observed in our study.25 To achieve a 1–3 log reduction of Cryptosporidium, the required chlorine C·t value is estimated to be in the range of 900–12
000 mg min l−1.55,56 To the best of our knowledge, the exposure of PAA needed to inactivate Cryptosporidium parvum oocysts by 1 log reduction in authentic secondary treated wastewater is not known. Given these previous studies and our results, neither the use of PAA nor NaOCl at concentrations relevant to wastewater treatment are effective against Cryptosporidium parvum oocysts.
4.2 Combined PAA and UV disinfection
We found the order of PAA and UV addition in the combined PAA and UV treatments did not affect the inactivation of E. coli and Enterococcus spp. at the concentration and contact time tested (PAA: 2 mg l−1,10 min; UV: 14.7 mJ cm−2). A previous study observed the same level of E. coli inactivation when PAA (contact time of 3–12 s) was applied before UV (165–192 mJ cm−2) and when UV was followed by PAA (contact time of 30 min).30 However, in the same study, the application of PAA (contact time of 3–12 s) followed by UV achieved greater inactivation for total and fecal coliforms.30 There are three main differences between their study and ours. First, our PAA contact time preceding UV application is at least 50 times higher than their contact time of 3–12 s. Second, our UV exposure is at least 10 times smaller than theirs. Lastly, we used secondary treated effluent and they used tertiary treated effluent (sand filtered effluent). While we both observed similar patterns for E. coli, it remains unclear if PAA's contact time (seconds vs. minutes) would have an effect on the sequential order of PAA and UV in advanced oxidative processes that rely on high UV exposures to treat wastewater. The effect that the sequential order of PAA and UV treatment has on viruses and protozoa was not investigated in this study and warrants future research, particularly for viruses.
The combined treatment of PAA (20 mg min l−1) followed by UV (14.7 mJ cm−2) achieved log reductions of 3.1–3.6 and 1.4 for E. coli and Enterococcus spp., respectively. Our results fall within the range reported by previous studies. Caretti et al.30 achieved log reductions of 4.3 for E. coli in authentic wastewater with 0.4 mg min l−1 PAA followed by UV (165 mJ cm−2). Another study observed log reductions of 1.1 and 1.6 for E. coli and Enterococcus spp., respectively, in authentic wastewater with 80 mg min l−1 PAA exposure followed by UV (6.5 mJ cm−2).57 In synthetic water, PAA and UV (20 mg min l−1, 14 mJ cm−2) achieved 4.7 log reductions of E. coli11 which is higher than what we observed in wastewater but could be due to characteristic of the water used for the experiments.58 In addition, our results show that UV can augment the PAA disinfection of E. coli, but no synergy was observed between PAA and UV. Similarly, another study observed no synergy when inactivating E. coli in secondary effluent using the same PAA exposure (2 mg l−1, 10 min, C·t = 20 mg min l−1) but with a much higher UV exposure (187 mJ cm−2).27 The authors observed a synergy however when the PAA concentration increased from 2 to 3 mg l−1 (holding the contact time and UV exposure constant).27 Taken together, these results suggest that 1) UV treatment can augment PAA disinfection but wastewater constituents might affect the extent of this augmentation and 2) extremely high UV exposures are required for synergy to occur. More research is warranted given supplementing PAA with UV disinfection could lower the PAA concentration or contact time needed to achieve inactivation targets which might be advantageous for some utilities.
In addition to enhancing bacterial inactivation, combined PAA plus UV treatment (and UV only) achieved greater somatic coliphage inactivation than PAA only. The combined treatment of PAA (20 mg min l−1) plus UV (14.7 mJ cm−2) achieved a maximum log reduction of 2.7 and UV only achieved a maximum log reduction of 2.4, while log reductions by PAA only were less than 1. Similarly, another study using secondary effluent achieved comparable log reduction of MS2 coliphage and reported no synergy between PAA (1.5 mg l−1, 30 min, C·t = 45 mg min l−1) and UV (20 mJ cm−2).29 Using a comparable UV exposure (20 mJ cm−2), the authors demonstrated that synergy existed between PAA and UV in secondary effluent only when the PAA concentration was 10 mg l−1 (for either 30 or 120 min) or when the contact time was 120 min (for either 1.5 or 10 mg l−1).29 Another study also achieved synergy for the inactivation of coliphages in secondary effluent but at PAA exposures of 30–40 mg min l−1 (3–4 mg l−1, 10 min) and at much higher UV exposures (125–187 mJ cm−2).27 In comparison to our results, de Souza et al.,27 achieved comparable coliphage log reductions of 2.5 and 0.59 by PAA + UV (3 mg l−1, 10 min, 125 mJ cm−2) and PAA (3 mg l−1, 10 min, C·t = 30 mg min l−1), respectively. Rajala-Mustonen et al.28 demonstrated that by using a combined PAA and UV treatment, the total disinfection time to inactivate spiked coliphages in treated wastewater could be reduced from 1 h to 12.5 min. These results collectively suggest that the use of PAA and UV as a combined treatment would be beneficial for the inactivation of viruses. It is not surprising that the addition of UV augments inactivation of viruses since the susceptibility of viruses in wastewater to UV treatment has been shown previously.28,29,59 However, previous research suggests that PAA has a different effect on different viruses.23,24,28,29 It remains unclear whether or not the combined effect of PAA and UV is virus specific. Our study specifically contributes to the literature on somatic coliphage in wastewater and suggests that adding UV treatment after PAA is beneficial for somatic coliphage inactivation. Future research on PAA and UV as a combined treatment should consider multiple types of viruses.
Previous studies have shown protozoa such as Cryptosporidium parvum are not easily removed by traditional treatment methods (e.g., chlorine, sand filters, activated sludge) but are sensitive to UV.54,58,60–62 UV exposures less than 20 mJ cm−2 were sufficient to achieve 1–3 log reductions of Cryptosporidium parvum oocysts in filtered surface water or synthetic waters.60–62 In authentic wastewater however, we did not observe any significant effects against Cryptosporidium parvum oocysts by either UV or PAA. We achieved negligible log reductions (<0.5) in authentic wastewater using 20–60 mg min l−1 PAA or 14.7 mJ cm−2 UV. To the best of our knowledge, the combined use of PAA and UV on Cryptosporidium parvum has not been previously examined. We could not conclude from our results whether Cryptosporidium parvum oocysts were more susceptible to either PAA or UV or whether or not the combined treatment enhanced its disinfection. Future work should aim to determine an effective exposure of PAA only and PAA plus UV to inactivate Cryptosporidium parvum oocysts in authentic wastewater.
4.3 Combined PAA and UV disinfection for water reuse
The disinfection efficiency of PAA and the combined use of PAA plus UV continued for 24 h. At 24 h, both PAA only and PAA plus UV treatments achieved significantly higher inactivation of E. coli and Enterococcus spp. than UV only, but were not significantly different from one another. These results suggest that the cellular damage caused by PAA continues to inactivate bacterial cells as contact time increases. No increase in E. coli and Enterococcus spp. concentrations was observed at 24 h. These results suggest that E. coli and Enterococcus spp. were not able to sufficiently repair any damage within 24 h despite the potential for cellular repair mechanisms63 and for regrowth due to increased organic content from the PAA (specifically acetic acid).5,6,18,64 This is consistent with a previous study by Rossi et al.,10 who showed E. coli log reductions immediately after PAA disinfection with 2–15 mg l−1 and with up to 30 min contact time were comparable to those attained after 5 h. Another study found that fecal coliforms and E. coli exhibited no regrowth after treatment with 2 mg l−1 PAA and a contact time of 29 h (negligible PAA residual was measured after 11 h) while total heterotrophic bacteria exhibited variable results.65 The inactivation or lack of regrowth observed at 24 h after PAA plus UV treatment and PAA treatment could be advantageous for wastewater or water reuse applications with longer hold times post disinfection.
4.4 Effects of wastewater source
Wastewater characteristics can affect the inactivation performance of disinfectants. Previous studies have also shown the importance of wastewater characteristics such as turbidity, total suspended solid, particle size, and biochemical oxygen demand can reduce the disinfection efficiency of PAA.11,28,33,49,66,67 Turbidity and UV254 (a measure of total organic carbon) have been associated with decreases in inactivation due to suspended solids reducing UV penetration.12,68 Our study used secondary effluent from different utilities to incorporate variability in wastewater characteristics into our study design. We did not observe a clear trend between wastewater characteristics and log reductions, but this could be due to a limited number of samples with different wastewater characteristics.
5 Conclusion
The inactivation of E. coli, Enterococcus spp., somatic coliphage, and Cryptosporidium parvum oocysts by NaOCl, PAA, and PAA plus UV was studied using authentic secondary wastewater from different utilities. PAA and NaOCl disinfection can be comparable but is dependent on contact time. At 2 mg l−1 for 30 min (C·t = 60 mg min l−1), NaOCl and PAA disinfection were comparable for the inactivation of E. coli, Enterococcus spp., and somatic coliphage at room temperature. However, at 2 mg l−1 for 10 min (C·t = 20 mg min l−1), only Enterococcus spp. inactivation was higher for NaOCl than for PAA. At a winter-relevant temperature (4 °C), NaOCl achieved greater inactivation of E. coli and Enterococcus spp. than PAA at both 20 and 60 mg min l−1. Somatic coliphage inactivation was comparable between PAA and NaOCl at both exposures and temperatures. Increases in contact time from 10 to 30 min had no effect on somatic coliphage. Cryptosporidium parvum oocysts were not effectively disinfected by either NaOCl or PAA at either C·t. Results suggest that the use of UV may augment PAA disinfection. The combined use of PAA (20 mg min l−1) plus UV (14.7 mJ cm−2) achieved the same or higher log reductions than PAA only or UV only for E. coli, Enterococcus spp. and somatic coliphage. PAA has the potential to be a useful disinfectant for water treatment applications with long contact times. Log reductions of E. coli, Enterococcus spp. and somatic coliphage by PAA were higher when enumerated at 24 h than at 10 min after PAA treatment while only the log reduction of Enterococcus spp. by PAA plus UV were higher when enumerated at 24 h than at 10 min. No bacterial regrowth was observed 24 h post disinfection.
Conflicts of interest
There are no conflicts to declare.
Acknowledgements
This work was supported by the funding provided by the NYS Pollution Prevention Institute through a grant from the New York State Department of Environmental Conservation (GC-2015-01-19). Any opinions, findings, conclusions or recommendations expressed are those of the authors and do not necessarily reflect the views of the New York State Department of Environmental Conservation. We thank Joseph Fiegl, Deputy Commissioner of the County of Erie for facilitating a network of support that made this research possible. We also thank Robert Klosko and the participating wastewater treatments plants for assisting and granting access to wastewater samples, and Jiale Xu for assisting in laboratory procedures.
References
-
U. S. EPA, EPA Wastewater Technology Fact Sheet: Chlorine Disinfection, EPA 832-F-99-062, 1999 Search PubMed.
- A. J. Stewart, W. R. Hill, K. D. Ham, S. W. Christensen and J. J. Beauchamp, Chlorine dynamics and ambient toxicity in receiving streams, Ecol. Appl., 1996, 6, 458–471 CrossRef.
- R. Crebelli, L. Conti, S. Monarca, D. Feretti, I. Zerbini, C. Zani, E. Veschetti, D. Cutilli and M. Ottaviani, Genotoxicity of the disinfection by-products resulting from peracetic acid- or hypochlorite-disinfected sewage wastewater, Water Res., 2005, 39, 1105–1113 CrossRef CAS PubMed.
- S. Monarca, D. Feretti, C. Collivignarelli, L. Guzzella, I. Zerbini, G. Bertanza and R. Pedrazzani, The influence of different disinfectants on mutagenicity and toxicity of urban wastewater, Water Res., 2000, 34, 4261–4269 CrossRef CAS.
- S. Monarca, S. D. Richardson, D. Feretti, M. Grottolo, A. D. Thruston Jr., C. Zani, G. Navazio, P. Ragazzo and I. Zerbini, Mutagenicity and disinfection by-products in surface drinking water disinfected with peracetic acid, Environ. Toxicol. Chem., 2002, 21, 309–318 CrossRef CAS PubMed.
- A. Dell'Erba, D. Falsanisi, L. Liberti, M. Notarnicola and D. Santoro, Disinfection by-products formation during wastewater disinfection with peracetic acid, Desalination, 2007, 215, 177–186 CrossRef.
- L. Domínguez Henao, A. Turolla and M. Antonelli, Disinfection by-products formation and ecotoxicological effects of effluents treated with peracetic acid: A review, Chemosphere, 2018, 213, 25–40 CrossRef PubMed.
-
U. S. EPA, Stage 1 Disinfectants and Disinfection Byproducts Rule: A Quick Reference Guide, EPA 816-F-01-010, 2001, pp. 1–2 Search PubMed.
- S. D. Freese, D. J. Nozaic, I. Bailey and D. L. Trollip, Alternative disinfectants for wastewater effluents: Viable or prohibitively expensive?, Water SA, 2002, 28, 23–32 Search PubMed.
- S. Rossi, M. Antonelli, V. Mezzanotte and C. Nurizzo, Peracetic acid disinfection: a feasible alternative to wastewater chlorination, Water Environ. Res., 2007, 79, 341–350 CrossRef CAS PubMed.
- J. Koivunen and H. Heinonen-Tanski, Inactivation of enteric microorganisms with chemical disinfectants, UV irradiation and combined chemical/UV treatments, Water Res., 2005, 39, 1519–1526 CrossRef CAS PubMed.
- V. Mezzanotte, M. Antonelli, S. Citterio and C. Nurizzo, Wastewater Disinfection Alternatives: Chlorine, Ozone, Peracetic Acid, and UV Light, Water Environ. Res., 2007, 79, 2373–2379 CrossRef CAS PubMed.
- R. K. Chhetri, E. Klupsch, H. R. Andersen and P. E. Jensen, Treatment of Arctic wastewater by chemical coagulation, UV and peracetic acid disinfection, Environ. Sci. Pollut. Res., 2018, 25, 32851–32859 CrossRef CAS PubMed.
-
K.-K. Au and B. T. Dunkley, Peracetic Acid to Replace Gaseous Chlorine for Wastewater Disinfection : From Bench Scale Testing to Pilot Demonstration, WEFTEC, 2016, pp. 319–330 Search PubMed.
- S. Stampi, G. D. Luca, M. Onorato, E. Ambrogiani and F. Zanetti, Peracetic acid as an alternative wastewater disinfectant to chlorine dioxide, J. Appl. Microbiol., 2002, 93, 725–731 CrossRef CAS PubMed.
-
E. Stec-Uddin, A. Atwater, K. Cowan, V. Anderson, B. McQuarrie, B. James and A. DaSilva, Evaluation of Peracetic Acid as an Alternative to Chloramine for Effluent Disinfection at the Robert W . Hite Treatment Facility, WEFTEC, 2017 Search PubMed.
-
M. Whalley, J. R. Bicudo, S. Wilson, G. Kumordzi, N. Fleming, D. Scott and B. D. Angelis, Pilot Demonstration of Peracetic Acid Disinfection – Do You Have the Time ?, WEFTEC, 2015, pp. 6196–6211 Search PubMed.
- J. Koivunen and H. Heinonen-Tanski, Peracetic acid (PAA) disinfection of primary, secondary and tertiary treated municipal wastewaters, Water Res., 2005, 39, 4445–4453 CrossRef CAS PubMed.
- T. Luukkonen, T. Heyninck, J. Rämö and U. Lassi, Comparison of organic peracids in wastewater treatment: Disinfection, oxidation and corrosion, Water Res., 2015, 85, 275–285 CrossRef CAS PubMed.
- F. Zanetti, G. De Luca, R. Sacchetti and S. Stampi, Disinfection efficiency of peracetic acid (PAA): Inactivation of coliphages and bacterial indicators in a municipal wastewater plant, Environ. Technol., 2007, 28, 1265–1271 CrossRef CAS PubMed.
- A. H. Hassaballah, J. Nyitrai, C. H. Hart, N. Dai and L. M. Sassoubre, A pilot-scale study of peracetic acid and ultraviolet light for wastewater disinfection, Environ. Sci.: Water Res. Technol., 2019, 5, 1453–1463 RSC.
- S. Bonetta, C. Pignata, E. Lorenzi, M. De Ceglia, L. Meucci, S. Bonetta, G. Gilli and E. Carraro, Peracetic Acid (PAA) disinfection: Inactivation of microbial indicators and pathogenic bacteria in a municipal wastewater plant, Water, 2017, 9, 1–10 CrossRef.
- N. Dunkin, S. Weng, K. J. Schwab, J. McQuarrie, K. Bell and J. G. Jacangelo, Comparative Inactivation of Murine Norovirus and MS2 Bacteriophage by Peracetic Acid and Monochloramine in Municipal Secondary Wastewater Effluent, Environ. Sci. Technol., 2017, 51, 2972–2981 CrossRef CAS PubMed.
- N. Dunkin, S. Weng, C. G. Coulter, J. G. Jacangelo and K. J. Schwab, Reduction of Human Norovirus GI, GII, and Surrogates by Peracetic Acid and Monochloramine in Municipal Secondary Wastewater Effluent, Environ. Sci. Technol., 2017, 51, 11918–11927 CrossRef CAS PubMed.
- R. Briancesco, E. Veschetti, M. Ottaviani and L. Bonadonna, Peracetic acid and sodium hypochlorite effectiveness in reducing resistant stages of microorganisms, Cent. Eur. J. Public Health, 2005, 13, 159–162 CAS.
- P. Sun, T. Zhang, B. Mejia-Tickner, R. Zhang, M. Cai and C.-H. Huang, Rapid disinfection by peracetic acid combined with UV irradiation, Environ. Sci. Technol. Lett., 2018, 5, 400–404 CrossRef CAS.
- J. B. de Souza, F. Queiroz Valdez, R. F. Jeranoski, C. M. De Sousa Vidal and G. S. Cavallini, Water and Wastewater Disinfection with Peracetic Acid and UV Radiation and Using Advanced Oxidative Process PAA/UV, Int. J. Photoenergy, 2015, 2015, 1–7 CrossRef.
- R. L. Rajala-Mustonen, P. S. Toivola and H. Heinonen-Tanski, Effects of peracetic acid and UV irradiation on the inactivation of coliphages in wastewater, Water Sci. Technol., 1997, 35, 237–241 CrossRef CAS.
- S. C. Weng, N. Dunkin, K. J. Schwab, J. McQuarrie, K. Bell and J. G. Jacangelo, Infectivity reduction efficacy of UV irradiation and peracetic acid-UV combined treatment on MS2 bacteriophage and murine norovirus in secondary wastewater effluent, J. Environ. Manage., 2018, 221, 1–9 CrossRef CAS PubMed.
- C. Caretti and C. Lubello, Wastewater disinfection with PAA and UV combined treatment: A pilot plant study, Water Res., 2003, 37, 2365–2371 CrossRef CAS PubMed.
- H. Charles, Water quality and disinfection kinetics, J. - Am. Water Works Assoc., 1996, 88, 95–103 Search PubMed.
- L. Domínguez Henao, R. Delli Compagni, A. Turolla and M. Antonelli, Influence of inorganic and organic compounds on the decay of peracetic acid in wastewater disinfection, Chem. Eng. J., 2018, 337, 133–142 CrossRef.
- M. McFadden, J. Loconsole, A. J. Schockling, R. Nerenberg and J. P. Pavissich, Comparing peracetic acid and hypochlorite for disinfection of combined sewer overflows: Effects of suspended-solids and pH, Sci. Total Environ., 2017, 599–600, 533–539 CrossRef CAS PubMed.
- C. T. Butterfield, E. Wattie, S. Megregian and C. W. Chambers, Influence of pH and Temperature on the Survival of Coliforms and Enteric Pathogens When Exposed to Free Chlorine, Public Health Rep., 1943, 58, 1837–1866 CrossRef CAS.
-
APHA, Standard Methods for the Examination of Water and Wastewater, American Public Health Association, 1998 Search PubMed.
-
HACH, Determination of Peracetic Acid (PAA) and Hydrogen Peroxide (H2O2) in Water, Application Note, 2014 Search PubMed.
- R. A. Dobbs, R. H. Wise and R. B. Dean, The use of Ultra-Violet absorbance for monitoring the total organic carbon content of water and wastewater, Water Res., 1972, 6, 1173–1180 CrossRef CAS.
- A. I. Silverman and K. L. Nelson, Modeling the endogenous sunlight inactivation rates of laboratory strain and Wastewater E. coli and enterococci using biological weighting functions, Environ. Sci. Technol., 2016, 50, 12292–12301 CrossRef CAS PubMed.
- R. O. Rahn, Potassium Iodide as a Chemical Actinometer for 254 nm Radiation : Use of Iodate as an Electron Scavenger, Photochem. Photobiol., 1997, 66, 450–455 CrossRef CAS.
- R. O. Rahn, M. I. Stefan, J. R. Bolton, E. Goren, P.-S. Shaw and K. R. Lykke, Quantum yield of the iodide-iodate chemical actinometer: dependence on wavelength and concentrations, Photochem. Photobiol., 2003, 78, 146–152 CrossRef CAS PubMed.
-
U. S. EPA, Method 1603: Escherichia coli (E. coli) in Water by Membrane Filtration Using Modified membrane-Thermotolerant Escherichia coli Agar (Modified mTEC), EPA-821-R-06-011, 2006, p. 42 Search PubMed.
-
U. S. EPA, Method 1600: Enterococci in Water by Membrane Filtration Using membrane-Enterococcus Indoxyl- B -D-Glucoside Agar (mEI), EPA-821-R-06-009, 2006, p. 42 Search PubMed.
-
U. S. EPA, Method 1602: Male-specific (F+) and Somatic Coliphage in Water by Single Agar Layer (SAL) Procedure April 2001, EPA Document 821-R-01-029, EPA-821-R-01-029, 2001, p. 38 Search PubMed.
-
U. S. EPA, Method 1623.1: Cryptosporidium and Giardia in Water by Filtration/IMS/FA, United States Protection Agency, 2012, p. 83 Search PubMed.
-
D. R. Helsel and R. M. Hirsch, Statistical Methods in Water Resources, USGS, 1994, vol. 36, p. 323 Search PubMed.
- R, R: A language and environment for statistical computing. R Foundation for Statistical Computing, Vienna, Austria, URL https://www.R-project.org/, R Core Team, Vienna, Austria, 2016.
- S. D. Freese and D. J. Nozaic, Chlorine: Is it really so bad and what are the alternatives?, Water SA, 2004, 30, 566–572 CAS.
- E. Veschetti, D. Cutilli, L. Bonadonna, R. Briancesco, C. Martini, G. Cecchini, P. Anastasi and M. Ottaviani, Pilot-plant comparative study of peracetic acid and sodium hypochlorite wastewater disinfection, Water Res., 2003, 37, 78–94 CrossRef CAS PubMed.
- S. Stampi, G. D. Luca and F. Zanetti, Evaluation of the efficiency of peracetic acid in the disinfection of sewage effluents, J. Appl. Microbiol., 2001, 91, 833–838 CrossRef CAS PubMed.
- R. A. Blaustein, Y. Pachepsky, R. L. Hill, D. R. Shelton and G. Whelan, Escherichia coli survival in waters: Temperature dependence, Water Res., 2013, 47, 569–578 CrossRef CAS PubMed.
- J. McCambridge and T. A. McMeekin, Relative effects of bacterial and protozoan predators on survival of Escherichia coli in estuarine water samples, Appl. Environ. Microbiol., 1980, 40, 907–911 CAS.
- B. Thammavongs, D. Corroler, J. M. Panoff, Y. Auffray and P. Boutibonnes, Physiological response of Enterococcus faecalis JH2-2 to cold shock: Growth at low temperatures and freezing/thawing, Lett. Appl. Microbiol., 1996, 23, 398–402 CrossRef CAS PubMed.
- Y. Ye, R. M. Ellenberg, K. E. Graham and K. R. Wigginton, Survivability, Partitioning, and Recovery of Enveloped Viruses in Untreated Municipal Wastewater, Environ. Sci. Technol., 2016, 50, 5077–5085 CrossRef CAS PubMed.
- A. M. Nasser, Removal of Cryptosporidium by wastewater treatment processes: a review, J. Water Health, 2016, 14, 1–13 CrossRef PubMed.
- T. Hirata, A. Shimura, S. Morita, M. Suzuki, N. Motoyama, H. Hoshikawa, T. Moniwa and M. Kaneko, The effect of temperature on the efficacy of ozonation fro inactivating cryptosporidium parvum oocysts, Water Sci. Technol., 2018, 43, 163–166 CrossRef.
- J. L. Rennecker, A. M. Driedger, S. A. Rubin and B. J. Mariňas, Synergy in sequential inactivation of Cryptosporidium parvum with ozone/free chlorine and ozone/monochloramine, Water Res., 2000, 34, 4121–4130 CrossRef CAS.
- F. Formisano, A. Fiorentino, L. Rizzo, M. Carotenuto, L. Pucci, M. Giugni and G. Lofrano, Inactivation of Escherichia coli and Enterococci in urban wastewater by sunlight/PAA and sunlight/H2O2processes, Process Saf. Environ. Prot., 2016, 104, 178–184 CrossRef CAS.
- W. A. M. Hijnen, E. F. Beerendonk and G. J. Medema, Inactivation credit of UV radiation for viruses, bacteria and protozoan (oo)cysts in water: A review, Water Res., 2006, 40, 3–22 CrossRef CAS PubMed.
- A. H. Havelaar, W. M. Pot-Hogeboom, W. Koot and R. Pot, F-specific bacteriophages as indicators of the disinfection efficiency of secondary effluent with ultraviolet radiation, Ozone: Sci. Eng., 1987, 9, 353–367 CrossRef CAS.
- J. L. Clancy, Z. Bukhari, T. M. Hargy, J. R. Bolton, B. W. Dussert and M. M. Marshall, Using UV to inactivate Cryptosporidium, J. - Am. Water Works Assoc., 2000, 92, 97–104 CrossRef CAS.
- S. A. Craik, D. Weldon, G. R. Finch, J. R. Bolton and M. Belosevic, Inactivation of cryptosporidium parvum oocysts using medium- and low-pressure ultraviolet radiation, Water Res., 2001, 35, 1387–1398 CrossRef CAS PubMed.
- S. Morita, A. Namikoshi, T. Hirata, K. Oguma, H. Katayama and S. Ohgaki, Efficacy of UV Irradiation in Inactivating Cryptosporidium parvum Oocysts, Appl. Environ. Microbiol., 2002, 68, 5387–5393 CrossRef CAS PubMed.
- K. Kollu and B. Örmeci, Regrowth Potential of Bacteria after Ultraviolet Disinfection in the Absence of Light and Dark Repair, J. Environ. Eng., 2015, 141, 04014069 CrossRef CAS.
- M. Kitis, Disinfection of wastewater with peracetic acid: A review, Environ. Int., 2004, 30, 47–55 CrossRef CAS PubMed.
- M. Antonelli, S. Rossi, V. Mezzanotte and C. Nurizzo, Secondary effluent disinfection: PAA long term efficiency, Environ. Sci. Technol., 2006, 40, 4771–4775 CrossRef CAS PubMed.
- D. Falsanisi, R. Gehr, L. Liberti and M. Notarnicola, Effect of suspended particles on disinfection of a physicochemical municipal wastewater with peracetic acid, Water Qual. Res. J. Can., 2008, 43, 47–54 CrossRef CAS.
-
R. Gehr, D. Cochrane and M. French, Peracetic Acid (PAA) as a disinfectant for municipal wastewaters: Encouraging performance results from physicochemical as well as biological effluents, Proceedings Disinfection 2002 Conference, Water Environment Federation, St. Petersburg, Florida, 2002, pp. 17–20 Search PubMed.
- L. Liberti and M. Notarnicola, Advanced Treatment and Disinfection for Municipal Wastewater Reuse in Agriculture, Water Sci. Technol., 1999, 40, 235–245 CrossRef CAS.
Footnote |
† Electronic supplementary information (ESI) available. See DOI: 10.1039/c9ew00837c |
|
This journal is © The Royal Society of Chemistry 2020 |
Click here to see how this site uses Cookies. View our privacy policy here.