DOI:
10.1039/C9FO00038K
(Paper)
Food Funct., 2020,
11, 871-882
Effect of high energy electron beam on proteolysis and antioxidant activity of rice proteins
Received
8th January 2019
, Accepted 29th December 2019
First published on 31st December 2019
Abstract
This research focused on the effects of electron beam irradiation (EBI) on the hydrolysis and antioxidant activity of rice proteins (RPs). The RPs were treated with 0, 5, 10, 20 and 30 kGy doses of EBI. The results showed that EBI pretreatment improved significantly (P < 0.05) the degree of hydrolysis, increasing the DH value by more than 15.09% at a dose of 30 kGy. In addition, radical scavenging results showed that EBI treatment had effects on antioxidant activity and could increase the DPPH and ABTS+ radical scavenging activity of rice protein hydrolysates (RPHs) by 32.06% and 79.11%, respectively (30 kGy). The CAA test also confirmed that EBI pretreatment could effectively improve the ability of RPHs to remove intracellular free radicals. Scanning electron microscopy indicated that EBI treatment destroyed microstructures and resulted in cracks and fragments of RPs. Circular dichroism analysis showed that EBI affected the secondary structure of RPs by destroying the α-helix structure. Changes in the UV visible spectra indicated unfolding of RPs by EBI. Amino acid and molecular weight distribution analysis revealed that EBI pretreatment could increase the ratio of antioxidant-related amino acids and produce smaller peptides. Therefore, EBI pretreatment is an efficient method to promote protein proteolysis due to its effect on the molecular conformation as well as on protein microstructure. Moreover, EBI treatment applied before enzymatic hydrolysis has the potential to prepare hydrolysates with high bioactivity.
1. Introduction
Rice proteins (RPs), as natural cereal proteins, are an excellent source of food proteins due to their unique nutritional value and hypoallergenic properties.1 However, as a byproduct produced during the starch extraction process,2 RPs often undergo denaturation due to high-temperature and high-pressure treatments, leading to low protein solubility that limits their applications in food formulations.3 Limited enzymatic hydrolysis is an efficient method to overcome this drawback and improve the functional properties and bioactivities of RPs. Moreover, natural antioxidants have attracted increasing attention, and a large number of studies have reported that protein hydrolysates of plant and animal origins exhibit significant antioxidant activity.4–7 Thus, RPs may potentially be used to prepare antioxidant peptides in the food industry. Previously, we demonstrated that hydrolysates of RPs obtained by various proteases could inhibit the oxidative damage induced by H2O2 in HepG-2 cells, and optimum use of alcalase could produce peptides with higher antioxidant activity.8 Although enzymatic modification of proteins via protease is a particularly attractive technique, it has many limitations, such as a low hydrolysis degree and protein-peptide yield.9
To improve the hydrolysis of rice protein, some physical methods have been applied, for instance, ultrasonic treatment,10 microwave treatment,11,12 ultra-high pressure13,14 and electron beam irradiation.9 Electron beam irradiation (EBI) is a process in which food is exposed to high-energy electrons produced by machine sources.15 Compared with other methods, EBI technology is very suitable for mass production with low cost and high efficiency.16 Moreover, as for the parameters, irradiation dose is the only technical parameter for EBI technology, and thus, it is easy to control. In addition, EBI is a cold process and does not produce radioactive waste. In terms of safety, a dose of 10 kGy is completely safe. However, a document issued by the WHO in 1999 stated that higher doses could be considered safe as long as the dose is technically available.17 As an emerging technology, EBI has been successfully applied to decontamination, assisted extraction, and disinfestation for improvement of food quality and safety.18–20
Studies on the application of EBI technology for food protein modification are gradually increasing. Electrons may alter various chemical and molecular bonds, resulting in either cross-linking or degradation of food proteins, thus influencing their functional properties and bioactivities.21–23 Wang et al. reported that the EBI treatment of pea proteins results in an increase in the emulsifying and foaming properties of their hydrolysates.24 The application of EBI has also been reported by Min et al. to change the foaming properties of egg white protein.25 A study by Sabato et al. noted that films based on Tilapia proteins were improved after irradiation with 100 kGy of EBI.26 Moreover, irradiation of cereal and crop seeds with doses of 15–60 kGy proved to be a viable alternative to increase crude protein digestibility.27–29 Several studies have also investigated the use of EBI to increase free-radical scavenging capacity of protein hydrolysates.24,30 However, whether EBI affects rice protein hydrolysates (RPHs) has not been reported. Therefore, the aim of this study was to investigate the effect of EBI treatment on the structure, hydrolysis and antioxidant activity of rice protein hydrolysates. The results of this study are expected to provide a theoretical basis to solve the problem of the low degree of hydrolysis of rice proteins.
2. Materials and methods
2.1 Materials
Rice dreg protein (82.9% protein, 7.9% water, 1.35% ash, 6.25% lipid, 1.60% sugar) was provided by Shanyuan Biotechnology Co. Ltd (Wuxi, China). Alcalase (2.4 L) was purchased from Novozymes (Beijing, China). DPPH; 2,2′-azino-bis(3-ethylbenzothiazoline-6-sulfonic acid) diammonium salt (ABTS); 6-hydroxy-2,5,7,8-tetramethylchroman-2-carboxylic acid (Trolox); 2′,7′-dichlorofluorescin diacetate (DCFH-DA) and 2,2′-azobis(2-amidinopropane) dihydrochloride (ABAP) were obtained from Sigma (St Louis, MO, USA). HepG-2 cells were purchased from the Institute of Biochemistry and Cell Biology, SIBS, CAS (Shanghai, China). Dulbecco's Modified Eagle's medium (DMEM), fetal bovine serum (FBS), Hanks’ Balanced Salt Solution (HBSS) and other cell culture materials were purchased from Gibco BRL, Life Technologies (USA). Cell Counting Kit-8 (CCK-8) was obtained from Beyotime Biotechnology Co. Ltd (Shanghai, China). All chemicals and reagents were of analytical grade or higher.
2.2 Electron beam treatment
Electron beam irradiation was conducted by the AIBANG EB-Tech Co., Ltd, Wuxi, China, using a high-energy linear accelerator. One hundred grams of the starting material was sealed in a sterile food-grade polyethylene plastic bag with a thickness of approximately 1.0 cm. Then, rice protein was irradiated (powder form) at varying doses (5 kGy, 10 kGy, 20 kGy, or 30 kGy, the irradiation depended on the exposure time) by electron beam irradiation at 5.0 MeV. All irradiations were performed at room temperature. After irradiation, the samples were stored at −20 °C until further use.
2.3 Enzymatic hydrolysis of RPs
Rice protein subjected to different EBI doses was stirred into distilled water (5% w/v), mixed for 30 min, and then enzymatic hydrolysis was carried out with 2.4 L of alcalase for 2 h at 55 °C, pH 8.5. After adding 1% protease (w/w), 1 M NaOH was applied to keep the pH slurry constant. Two hours later, the enzyme was inactivated using immersion in a boiling water bath for 10 min. The hydrolysate was then centrifuged at 10
000g for 20 min and the supernatant was freeze-dried and stored at −20 °C for further use. The degree of hydrolysis (DH) was calculated as follows:
DH = (V × C)/(MP × htot × α) × 100 |
where V and C are the volume (mL) and concentration (mol L−1) of NaOH, respectively. MP is the protein mass of hydrolysis (g); htot is the hydrolysis equivalent per gram of protein (mmol g−1) (for rice protein, htot is 7.4 mmol per g protein); and α is the calibration factor for pH-stat (for alcalase, 1/α is 1.01).
2.4 Protein solubility
The solubility of RPs under optimum hydrolysis conditions as described in 2.3 was determined. RP dispersions (5%, w/v) in distilled water were prepared and adjusted to a pH of 8.5 with 1 M NaOH. These were mixed for 30 min at 55 °C and centrifuged at 5000 rpm for 15 min. After appropriate dilution, the protein contents in the supernatants were measured by the Folin–phenol method.31 Bovine serum albumin was used as the standard.
2.5 Determination of free radical scavenging capacity
2.5.1 DPPH radical scavenging activity.
The DPPH scavenging activity was detected according to the previously reported method32 with some modifications. Firstly, 0.1 mM DPPH solution was prepared by dissolving 0.039 g DPPH in 1000 mL ethanol and 5 mg mL−1 rice protein hydrolysate sample was prepared by dissolving 0.05 g rice protein hydrolysate sample (with or without EBI irradiation) in 10 mL distilled water. Then 100 μL of the sample was mixed with 100 μL of DPPH and then incubated for 30 min at room temperature in the dark. A microplate reader (SH-1000, Hitachi, Japan) was used to measure the absorbance at 517 nm. The DPPH radical scavenging activity was calculated using the following equation:
DPPH scavenging effect (%) = [AC − (AS1 − AS2)]/AC × 100 |
where AC, AS1, AS2 are the absorbance of the control, sample, and sample without DPPH solution, respectively.
2.5.2 ABTS+ radical scavenging activity.
First, an ABTS+ working solution was prepared. 7 mmol L−1 ABTS+ stock solution and 2.45 mmol L−1 potassium permanganate solution were mixed and then stored at room temperature for 12–16 h in the dark. Before use, a solution was diluted using PBS (0.1 mmol L−1, pH 7.4) until the absorbance at A734 was in the range of 0.70 ± 0.02. 5 mg mL−1 rice protein hydrolysate sample was prepared by dissolving 0.05 g rice protein hydrolysate sample (with or without EBI irradiation) in 10 mL distilled water. After that, 4 mL of ABTS+ and 100 μL of the protein hydrolysates (or Trolox as an antioxidant standard) were mixed and reacted for 10 min in the dark. The absorbance at 734 nm was measured using a spectrophotometer (UV-1800, Shimadzu, Japan). Trolox equivalents (μmol g−1 RPHs), which were calculated by a standard curve prepared with Trolox, were used to express the ABTS+ radical scavenging activity.
2.6 Cellular antioxidant activity (CAA)
2.6.1 Cell culture.
Human HepG-2 cells were propagated in a DMEM nutrient mixture supplemented with 10% FBS, 100 units per mL penicillin, and 100 μg mL−1 streptomycin at 37 °C in an incubator with 5% CO2 and 95% air. All experiments were carried out at 12 h after the cells were seeded onto microplates.
2.6.2 Cell morphology.
HepG-2 cells were seeded onto 96-well plates at a density of 4 × 105 cells per mL and incubated at 37 °C in a CO2 incubator overnight. The cells were incubated with 100 μL of complete culture medium containing 0.2, 0.4, 0.6, 0.8, 1.0, 1.2 mg mL−1 of RPHs and cultured for 48 h for morphology analysis. Cell morphology was observed and photographed with an inverted biological microscope.
2.6.3 Cell cytotoxicity.
HepG-2 cells were seeded onto 96-well plates at a density of 4 × 105 cells per mL and incubated at 37 °C in a CO2 incubator overnight. The cells were incubated with 100 μL of complete culture medium containing 0.2, 0.4, 0.6, 0.8, 1.0, 1.2 mg mL−1 of RPHs. The cells were incubated for 48 h for cytotoxicity analysis. Cell growth was determined by adding 10 μL CCK-8 to each well and incubating for 4 h. After removal of the medium, 150 μL DMSO was added to each well and wells were shaken gently and carefully. The cell viability was expressed as the following equation:
Cell viability = Atreated/Acontrol × 100%. |
2.6.4. CAA.
HepG-2 cells were seeded onto 96-well plates at a density of 4 × 105 cells per mL and incubated at 37 °C in a CO2 incubator overnight. Then, the growth medium was removed, and the wells were washed with PBS. The cells were incubated with 100 μL of culture medium containing 25 μM DCFH-DA and a series of concentrations (0.2, 0.4, 0.6, 0.8, 1.0, 1.2 mg mL−1) of RPHs. After a 1 h incubation, the wells were washed with 100 μL of PBS. Then, 600 μM ABAP was applied to the cells in 100 μL of HBSS, and the absorbance was measured at 450 nm by a microplate reader (M5, Molecular Devices, USA) at 37 °C. Emission at 538 nm was measured with excitation at 485 nm every 5 min for 1 h. The control wells contained cells treated with DCFH-DA and an oxidant. The blank wells contained cells treated with dye and HBSS without the oxidant. The CAA value at each concentration of RPHs was calculated as follows:
where
is the integrated area under the sample fluorescence versus time curve.
is the integrated area under the control fluorescence versus time curve.
The median effective dose (EC50) was determined by the plot of log(fa/fu) versus log(dose), where fa is the CAA unit and fu is the 1 − CAA unit.
In the experiment, quercetin was used as a standard, and cellular antioxidant activities (CAA value) for RPHs were expressed as micromoles of quercetin equivalents (QE) per g of RPHs.
2.7 Scanning electron microscopy (SEM)
The samples were first coated with gold–palladium. Then, the microcosmic surface structure of sample granules was observed with a FEI Quanta™ 200 scanning electron microscope (FEI Co., Hillsboro, USA). The surface structure of the sample was observed at 5000× magnification.
2.8 Fourier transform infrared spectroscopy (FTIR) analysis
2 mg of rice protein was mixed with KBr, grounded, and then pressed into a pellet. A Nicolet iS10 FTIR spectrometer (ThermoFisher Scientific, Marietta, OH, USA) was used to record the FTIR spectroscopy. The wavenumber was ranged from 4000 cm−1 to 400 cm−1, at a 2 cm−1 resolution. Omnic V8.1 (ThermoFisher Scientific, USA) and PeakFit 4.12 (SeaSolve Software Inc., USA) were used to analyze the amide I band and determined the content of α-helix, β-sheet, turn and unordered structure in the protein mixtures.
2.9 Circular dichroism (CD) spectroscopy
Far-UV CD spectra of RPs were recorded using a MOS-450 spectrometer (BioLogic Science Instruments, Ltd, Claix, France). RPs were dissolved in 0.01 M PBS (pH 8.0) at a concentration of 0.5 mg mL−1. A 0.1 cm path length quartz cell and a 0.2 nm band were used for the measurement. The content of the protein secondary structure was calculated by CDSSTR.
2.10 UV visible spectra
Samples were prepared with 0.01 M phosphate buffered solution (PBS, pH 8.0) at a final concentration of 2.0 mg mL−1. The UV visible spectra of the sample solutions were recorded at 200–400 nm with a Varian Cary 100 UV-Vis spectrophotometer (Varian Inc., Palo Alto, USA) at 25 °C. For the blank, 0.01 M PBS (pH 8.0) was used.
2.11 Amino acid analysis
2.11.1 Free amino acid.
600 mg of RPHs were dissolved in trichloroacetic acid (5% w/v) and diluted with water to 25 mL. The sample was treated with ultrasound for 20 min, left stationary for 2 h and filtered. The sample was then centrifuged at 15
000 rpm for 30 min and analyzed by an automatic amino acid analyzer (L-8800, Hitachi, Japan).
2.11.2 Total amino acid.
200 mg of RPHs were filled in sealed, evacuated glass tubes and digested with 6 M HCl at 110 °C for 24 h. Then, the hydrolysates were evaporated under nitrogen at 60 °C. After cooling, the hydrolysates were diluted with water to 100 mL and filtered. The sample was then centrifuged at 15
000 rpm for 30 min. An automatic amino acid analyzer (L-8800, Hitachi, Japan) was used to analyze the amino acids.
2.12 Determination of molecular weight distribution
Gel permeation chromatography with an HPLC system (1260 Infinity, Agilent Technologies, USA) was applied to detect the molecular weight distribution of the RPHs. A TSkgel2000 SW XL column (7.8 mm i.d. × 300 mm; Tosoh, Tokyo, Japan) was used at a flow rate of 0.5 mL min−1. The mobile phase proportion was as follows: aceto-nitrile/water/trifluoroacetic acid was 45/55/0.1 (v/v). A molecular weight calibration curve was prepared using the following protein standards: cytochrome C (12.5 kDa), bacitracin (1450 Da), tetrapeptide GGYR (451 Da), and tripeptide GGG (189 Da) (Sigma St Louis, MO, USA).
2.13 Statistical analysis
Data were analyzed using IMB SPSS statistics 2.0 software. The differences between the mean values of the samples were determined using the least significant difference (LSD) test at a level of 0.05.
3. Results and discussion
3.1 Effect of EBI treatment on hydrolysis of RPs
DH is an index reflecting the degree of protein hydrolysis; an increased DH means an improved production efficiency of protein peptides. In this study, alcalase was selected to produce protein hydrolysates because it is a nonspecific endopeptidase that can generate extremely small peptides with high antioxidant activity.8,33 The protease was used under the optimum hydrolysis conditions as described by the manufacturer, with data listed in Fig. 1. As depicted, all irradiated samples presented significantly higher DH (P < 0.05) when compared with the native protein. Moreover, the DH increased with increasing irradiation doses. Irradiation of RPs at 30 kGy resulted in the highest hydrolytic efficiency, with DH increased from 21.80% to 25.09%. Similar results were observed with the hydrolysis of egg white protein and corn gluten meal.9,30 In addition, it could be observed that the initial reaction rate for the irradiated groups increased quite rapidly compared with the control group (0 kGy) (Fig. 1A). The reason might be that the high energy electron beams destroyed the microcosmic surface structure of RPs and produced many fragments (Fig. 5), which enlarged the contact area of protein substrates and enzymes and resulted in high hydrolysis efficiency. On the other hand, irradiation may induce unfolding of the protein and expose more peptidic bonds that are active sites for enzymes, thus resulting in rice proteins that are hydrolyzed more easily.34 However, the reaction rates remained steady after 60 min, and the DH differences between RPHs pretreated with EBI and the control group (treated with alcalase and without EBI) remained steady (Fig. 1A and B). The steady of reaction rates may be because most of the substrate was hydrolyzed by the enzyme with increasing reaction time. The results showed that EBI pretreatment of RPs could effectively promote hydrolysis efficiency and increase the yield of polypeptides within the same enzymatic hydrolysis time.
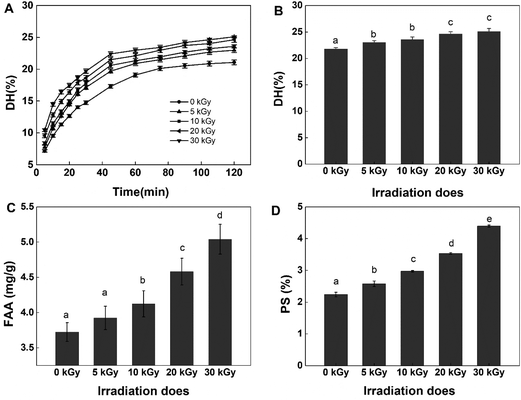 |
| Fig. 1 Effects of EBI pretreatment on the enzymatic hydrolysis of RPs. (A) The enzymatic hydrolysis curves for different irradiation doses; (B) effects of irradiation on the degree of hydrolysis at 120 min; (C) changes in free amino acids (FAAs) in the hydrolysates at 120 min; (D) effects of irradiation on the protein solubility (PS) of RPs. Data points represent the mean ± SD (n = 3). | |
In regards to DH, as expected, EBI pretreatment substantially increased the content of free amino acids (FAAs) in the hydrolysates (Fig. 1C). Generally, more FAAs will be released with an increase in the degree of enzymatic hydrolysis. As shown in Fig. 1, the FAA content increased slightly at low irradiation doses (<10 kGy) and then increased dramatically with the further increase of irradiation dose, reaching the maximum value at 30 kGy (increased 1.5-fold). This can be attributed to the ability of EBI to unfold the structure of proteins, exposing more amino-terminal residues that are more easily hydrolyzed into free amino acids. The results further confirmed that EBI pretreatment of RPs increased the degree of hydrolysis.
With regard to protein solubility (PS) at 55 °C and pH 8.5 (the optimum hydrolysis conditions for alcalase), the EBI pretreatment resulted in significantly (P < 0.05) higher PS values compared with the control group (Fig. 1D). At the same time, dose-dependent changes in PS were observed with the maximum levels occurring at 30 kGy (increasing by 51.82% vs. the control group). The results indicated that the high energy electron beam might have broken the cross-linkage and caused more protein molecules to dissolve in the solution. In this way, the amount of proteins in contact with enzymes in the early stage of the reaction increased, and thus, the reaction rate increased, which was consistent with the analysis results shown in Fig. 1A.
3.2 Effect of EBI treatment on free radical scavenging capacity of RPHs
To test the effect of EBI on the antioxidant activity of rice protein hydrolysates, DPPH and ABTS+ radical scavenging abilities were tested in this study. Both methods are widely applied to examine the antioxidant activities of food products because of their high sensitivity, wide detection range and reliable results.35,36 The DPPH and ABTS+ radical scavenging activity of RPHs irradiated at various doses are presented in Fig. 2. Pretreatment of RPs with EBI had a positive effect on the DPPH radical scavenging capacity of RPHs. Untreated RPHs showed a DPPH radical scavenging rate of 55.64 ± 0.57%. EBI treatment improved the DPPH radical scavenging capacity of RPHs in a dose-dependent manner, and the highest value was 73.48 ± 0.98%, increasing by 32.06% in comparison with the control (0 kGy). Similarly, EBI treatment improved the ABTS+ radical scavenging capacity of RPHs (Fig. 2B). In addition, the ABTS+ radical scavenging activity exhibited a dose-dependent rise in irradiated samples and reached a maximum value at 30 kGy (increased from 36.90 to 66.09 μmol g−1 RPHs). Thus, samples obtained from RPs pretreated with EBI possessed a much better DPPH and ABTS+ radical scavenging capacity than the untreated group. This is mainly because EBI-induced protein unfolding may also expose more amino acid residues and produce low molecular weight peptides that effectively eliminate free radicals and contribute to the increase in antioxidant activity. On the other hand, DPPH and ABTS+ are chemically synthesized free radicals, among which DPPH is an oil-soluble free radical and ABTS+ is a water-soluble free radical. These become stable products after accepting an electron or a hydrogen from an antioxidant. The results showed that RPHs had a strong ability to transfer electrons or hydrogen. Furthermore, after treatment with EBI and enzymatic hydrolysis, more amino acid residues with electron-sharing properties were exposed, which made electron transfer more convenient and RPHs showed better antioxidant properties.
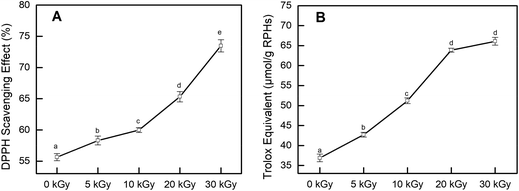 |
| Fig. 2 Effects of EBI pretreatment on the free radical scavenging capacity of RPHs. (A) DPPH radical scavenging activity; (B) ABTS+ radical reducing power. Data points represent the mean ± SD (n = 3). | |
Several studies have reported that irradiation has a positive effect on the antioxidant properties of many agricultural products. Choi et al. indicated in their study that the DPPH radical scavenging activities of the ethanol extracts from Hizikia fusiformis cooking drips increased with irradiation doses, which was supported by the observed increase in polyphenolic compounds content.37 Wang et al. found that electron beam irradiation enhances the antioxidant activity of wheat germ protein hydrolysates because of the low-molecular-weight peptides produced by irradiation.38 Fernandes et al. demonstrated that the antioxidant activity on irradiated mushroom samples was improved. They explained that the improvement may be related to the high amount of tocopherols detected in the irradiated samples.39 These findings are consistent with our experimental results indicating that irradiation effectively improved the antioxidant performance of the samples.
3.3 Effects of EBI treatment on cellular antioxidant activity (CAA) of RPHs
3.3.1 Cell morphology and cell viability.
CAA, as a widely used method for determining antioxidant capacity in recent years, is a more biologically relevant model because it takes place within the context of the cellular environment.40 Before the CAA test, effects of EBI treated RPHs and untreated RPHs on HepG-2 cells morphology were tested to ensure the accuracy of the experimental results. As can be seen in Fig. 3A, HepG-2 cells in the control group grew vigorously after 48 h of culture. The cell body was completely adhered to the wall and closely connected, and the color was even and bright. There was no significant difference in cell morphology between the control group and the experimental group pretreated with RPHs (1.2 mg mL−1), and the cell morphology remained intact. When the sample concentration was lower than 1.2 mg mL−1, cell morphology was not affected (data not shown). The results showed that RPHs before and after EBI treatment could not affect the morphology of HepG2 cells.
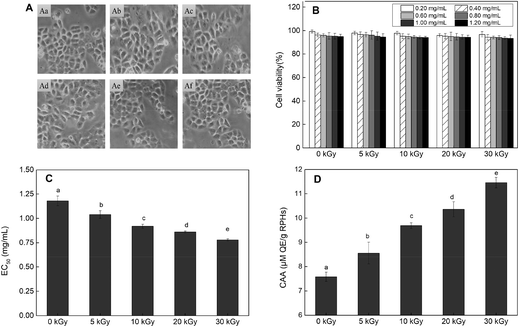 |
| Fig. 3 Effects of EBI treated RPHs and untreated RPHs on the cellular antioxidant capacity in of HepG-2 cells. (A) Cell morphology. A(a): Cells were cultured with growth medium only. A(b–f): Cells were co-cultured with RPHs irradiated at 0, 5, 10, 20 and 30 kGy; (B) cell viability. (C) EC50 values for the inhibition of peroxyl radical-induced DCFH oxidation by RPHs; (D) cellular antioxidant activity (CAA) of RPHs. Data points represent the mean ± SD (n = 3). | |
In addition, the CCK-8 assay was used to evaluate whether RPHs would cause cell death in the concentration range of 0.20–1.20 mg mL−1. Fig. 3B shows that the cell viability of all HepG-2 cells cultivated with EBI-pretreated RPHs was higher than 90%, suggesting that there was no cell toxicity at the concentrations used in this study.41 Thus, we selected a 0.20–1.20 mg mL−1 sample concentration for subsequent analysis.
3.3.2 Fluorescence in HepG-2 cells.
Fig. 4 shows the peroxyl radical-induced oxidation of DCFH to DCF in HepG-2 cells and the inhibition of oxidation by RPHs obtained from irradiated RPs over time. In general, antioxidants can inhibit the generation of DCF and decrease the fluorescence value in cells. As shown in Fig. 4B–F, the fluorescence signal intensity of all samples in the selected concentration range decreased with increasing concentration, indicating that RPHs can effectively inhibit the generation of oxidative free radicals in cells and that their antioxidant capacity is positively correlated with the concentration of RPHs. At the same time, it was found that the fluorescence of the irradiated groups decreased faster when the concentration increased from 0.60 mg mL−1 to 1.20 mg mL−1, compared with that of the control group. The results showed that pretreatment of RPs with EBI could enhance the cellular antioxidant activity of RPHs.
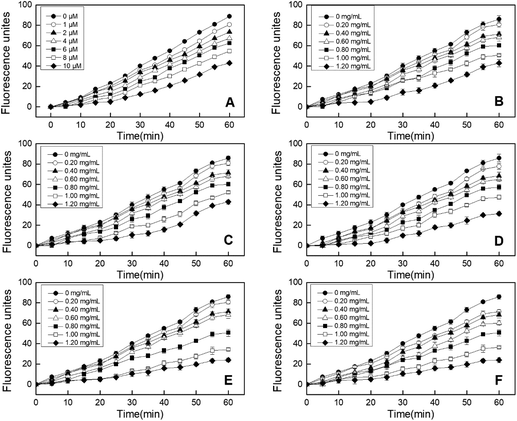 |
| Fig. 4 Peroxyl radical-induced oxidation of DCFH to DCF in HepG-2 cells and the inhibition of oxidation by quercetin (A) and RPHs irradiated at 0, 5, 10, 20 and 30 kGy (B–F) over time. Data points represent the mean ± SD (n = 3). | |
3.3.3 cellular antioxidant capacity.
Samples with low EC50 values showed higher antioxidant activity. To further evaluate the influence of EBI pretreatment on the antioxidant capacity of RPHs in HepG-2 cells, we calculated the EC50 value of RPHs under different EBI treatment conditions (0 kGy, 5 kGy, 10 kGy, 20 kGy, 30 kGy) according to Wolfe's method,40 and the results are shown in Fig. 3C. EBI treatment of RPs before enzymatic hydrolysis can significantly reduce the EC50 value of the RPHs. Moreover, the EC50 of RPHs treated with EBI declined with the increase in the radiation dose and dropped to the lowest value at 30 kGy (from 1.18 mg mL−1 to 0.77 mg mL−1). On the other hand, the EC50 values were converted to CAA values, expressed as micromoles of quercetin (QE) per gram of RPHs (Fig. 3D). The CAA values of irradiated RPHs presented marked increases when compared with the unmodified sample. As the irradiation dose increased, the CAA values increased up to a maximum value (11.46 ± 0.22 μM QE per g RPHs) at 30 kGy, which was an increase of 51.19%. The results demonstrated that RPHs pretreated with EBI could effectively inhibit the oxidation of DCFH in cells at a lower concentration and showed higher antioxidant activity in comparison with the non-irradiated sample. This may be because EBI treatment facilitates the enzymatic hydrolysis efficiency of RPs and increases the content of peptides with small molecular weight and FAAs in the RPHs (Fig. 1C). These small molecular weight compounds are more likely to enter the cell, clear the peroxyl radical generated by ABAP in the cell, inhibit the oxidation of DCFH to DCF, and thus enhanced the cellular antioxidant activity.
3.4 Effect of EBI treatment on structural properties
3.4.1 Microcosmic surface structure of RPs.
Scanning electron microscopic photographs of RPs before and after irradiation treatment are shown in Fig. 5. The microcosmic surface structure of RPs changed significantly after irradiation. As shown in Fig. 5A, the non-irradiated RPs appeared to be spherical structures with tight and rough surfaces. The compact structure was destroyed when RPs were subjected to electron beam irradiation. Moreover, the damaging effect was more significant with increasing radiation dose. Clumps of protein were broken down to form many small aggregates and fragments (Fig. 4B–E, b–f). The results indicated that EBI at higher doses destroyed the integrity of the sample, unfolded the closed structure, and increased the surface area of the protein. Because of the increased irregular convex shapes of the protein and fragments, the surface area of the substrate in contact with the enzyme increased, making it easier to hydrolyze by the protease. This, in turn, formed significantly smaller molecular peptides and released more essential amino acids, which played an important role in antioxidant activity.42 The results of microcosmic surface structural analysis provide evidence for the increased surface area of RPs modified by irradiation and the improved enzymatic hydrolysis.
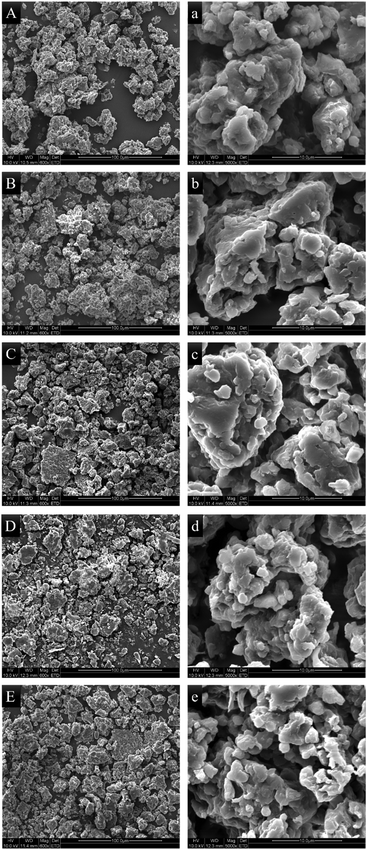 |
| Fig. 5 Scanning electron microscopic photographs of RPs irradiated at 0, 5, 10, 20 and 30 kGy, at 600× magnification (A–E) and 5000× magnification (a–e), respectively. | |
3.4.2 Secondary structure of RPs.
The effect of EBI on the secondary structure of RPs was determined by means of fourier transform infrared spectroscopy (FTIR) and far UV circular dichroism (CD) spectra. FTIR analysis of the amide I band reflect the secondary protein structure of solid powder. CD analysis reflect the secondary protein structure of soluble sample. Both results showed that the secondary structure of RPs was changed upon electron beam irradiation (Fig. 6A and B). The α-helix content sharply decreased as a result of electron beam irradiation, and the decrease was proportional to irradiation dose. In contrast to the α-helix content, the relative fractions of β-sheet, β-turn and random coils were slightly increased. This indicated that the covalent bonds in proteins were cleaved and the ordered structure of protein was disrupted by electron beam irradiation, hence decreasing the native protein structure and resulting in relatively loose β structure. Similar results were observed by many studies. Lv et al. reported that EBI treatment led to the conversion of the α-helix structure to the β-sheet structure in myofibrillar protein from Tegillarca granosa meat, but these effects were less dependent on the dose of irradiation.43 Malik et al. stated that gamma irradiation changed the conformation of sunflower protein isolates with a decreased α-helix structure and concomitant increased β-sheet, β-turn and random coil content.44 Moon et al. found that increasing the irradiation dose can further damage the ordered structure of proteins.45
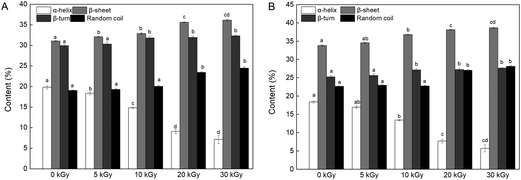 |
| Fig. 6 Effects of EBI pretreatment on the secondary structural content of RPs irradiated at 0, 5, 10, 20 and 30 kGy. (A) FTIR analysis; (B) CD analysis. Data points represent the mean ± SD (n = 3). | |
On the other hand, the changes in the content of the secondary structure might affect the enzymatic hydrolysis process. Proteins with a high content of β-sheets and random coils may be more accessible to the enzyme because of the loose protein molecules.46 In addition, reduction in α-helix content relates to a partial opening of this structure and exposed a number of buried enzyme sites of the protein, which promoted the process of enzymatic hydrolysis.43,47 Therefore, it can be speculated that the DH improvement may be connected with an unfolded α-helix structure and increased β-sheet and random coil content (Fig. 6).
3.4.3 UV-visible spectra and the second-derivative spectra of RPs.
Fig. 7 shows the UV-visible spectra and its second-derivative spectra of RPs. The characteristic absorption at 200 nm is mainly related to the existence of peptide bonds.48 The results showed that the absorbance intensity of RPs at 200 nm increased after treatment with EBI. At the same time, the absorption intensity increased significantly with increasing irradiation (Fig. 7A). These results may be due to the unfolding of protein molecules and subsequent exposure of chromophore (C
O and –COOH) in the peptide bond. Moreover, changes in the spatial structure of chromophore and co-chromophore (–OH and –NH2, respectively) in peptide molecules also affect the intensity of UV absorption peaks of peptide bonds. Therefore, it can be speculated that the spatial structure of the peptide bond changes after EBI treatment, and more peptide bonds are exposed to participate in the enzymatic hydrolysis reaction, thus accelerating the enzymatic hydrolysis process.
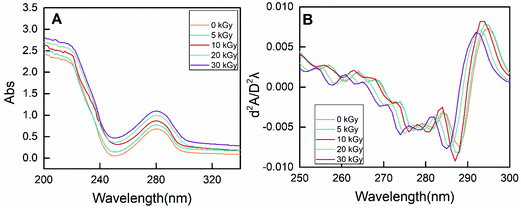 |
| Fig. 7 The zero-order (A) and second-derivative (B) UV spectra of RPs prepared by different irradiation doses. | |
On the other hand, the characteristic absorption at 250–300 nm is mainly due to the presence of phenylalanine, tyrosine and tryptophan.49 As shown in Fig. 7A, the absorbance intensity of RPs pretreated by EBI was strengthened, indicating exposure of the buried hydrophobic groups. Moreover, conformational changes of proteins can be evaluated by the peak displacement characteristics of each amino acid residue. Therefore, second-derivative UV spectra were used to separate the superimposed absorption peak, and the results are shown in Fig. 7B. Two positive peaks (285 nm and 295 nm) and two negative bands (282 nm and 288 nm) were observed for non-irradiated RPs. The absorption peak at 285 nm can be attributed to the combined action of tyrosine and tryptophan residues. EBI treatment caused the absorption peak to move towards the lower wavenumber (blue-shift), and the degree of blue-shift increased with increasing irradiation dose. The absorption peak moved from 285 nm to 281 at 30 kGy. In addition, the absorption at 295 nm is only due to the presence of tryptophan residues. A blue-shift of the peak at 295 nm occurred for rice proteins treated with EBI, and this change was does-dependent. It can be seen that the characteristic spectrum peaks in the second derivative UV spectrum of RPs all exhibited a certain blue-shift after EBI treatment. This indicated that the amino acid residues migrate to a more hydrophilic environment, resulting in conformational changes of proteins.50,51 Such conformational changes of RPs make enzyme contact easier, thus improving the efficiency of enzymatic hydrolysis.
3.4.4 Amino acid content of RPHs.
The amino acid content of the rice protein hydrolysates prepared with different irradiation doses are given in Table 1. There was no change in amino acid composition, indicating that EBI did not affect the nutritional composition of RPs and that it maintained the nutritional value of the original protein. Otherwise, the total amino acid (TAA) content of the rice proteins increased with an increase in irradiation. A similar result was reported by Olotu et al. They observed that the increased amino acid content at 10 kGy may be due to reductive deamination and decarboxylation.52 In contrast, methionine and cystine, which are sulfur-containing amino acids, were low in concentration but exhibited a dramatic decline upon irradiation. This is due to the extreme sensitivity of these two amino acids prone to oxidation when irradiated, allowing them to be oxidized when irradiated.53
Table 1 Amino acid percentage of RPHs irradiated at 0, 5, 10, 20 and 30 kGy
Amino acid |
Amino acid content (%) |
0 kGy |
5 kGy |
10 kGy |
20 kGy |
30 kGy |
Not detected. TAA, total amino acids, (g per 100 g); TEAA, total essential amino acids. His, thr, val, met, ile, leu, lys (%); THAA, total hydrophobic amino acids. ala, cys-s, val, met, phe, ile, leu (%); TAAA, total antioxidant related amino acid. asp, glu, arg, val, phe, leu, pro (%).
|
asp |
10.84 ± 0.17a |
10.92 ± 0.07a |
10.98 ± 0.19a |
11.09 ± 0.24a |
11.03 ± 0.10a |
glu |
18.93 ± 0.07a |
18.34 ± 0.06bc |
18.33 ± 0.38bc |
18.17 ± 0.05b |
18.57 ± 0.06c |
ser |
3.93 ± 0.11a |
3.75 ± 0.11ab |
3.5 ± 0.15c |
3.63 ± 0.07bc |
3.65 ± 0.11bc |
his |
2.53 ± 0.14a |
2.48 ± 0.12a |
2.42 ± 0.12a |
2.53 ± 0.11a |
2.56 ± 0.13a |
gly |
4.73 ± 0.09a |
4.8 ± 0.08a |
4.32 ± 0.08b |
4.04 ± 0.08c |
3.2 ± 0.11d |
thr |
3.31 ± 0.10ab |
3.27 ± 0.15a |
3.47 ± 0.08b |
3.46 ± 0.08ab |
3.43 ± 0.07ab |
arg |
9.33 ± 0.15a |
9.75 ± 0.08b |
9.62 ± 0.09b |
9.37 ± 0.11a |
9.41 ± 0.06a |
ala |
5.51 ± 0.16a |
5.94 ± 0.08b |
5.87 ± 0.08b |
6.23 ± 0.07c |
6.3 ± 0.09c |
tyr |
3.66 ± 0.09a |
3.26 ± 0.08b |
3.47 ± 0.13c |
3.64 ± 0.07ac |
3.56 ± 0.09ac |
cys-s |
0.55 ± 0.09a |
0.48 ± 0.09a |
0.39 ± 0.08ab |
0.23 ± 0.10bc |
0.14 ± 0.09c |
val |
6.98 ± 0.08a |
6.94 ± 0.14a |
6.92 ± 0.09a |
6.92 ± 0.09a |
6.79 ± 0.08a |
met |
2.08 ± 0.13a |
1.1 ± 0.13b |
0.98 ± 0.08b |
0.67 ± 0.08c |
0.64 ± 0.07c |
phe |
5.53 ± 0.08a |
5.65 ± 0.08a |
5.58 ± 0.13a |
5.67 ± 0.07a |
5.63 ± 0.07a |
ile |
4.84 ± 0.13a |
5.79 ± 0.09a |
5.75 ± 0.18a |
5.69 ± 0.08a |
5.76 ± 0.07a |
leu |
8.09 ± 0.11a |
8.41 ± 0.1b |
8.96 ± 0.08c |
9.29 ± 0.07d |
9.99 ± 0.06e |
lys |
3.67 ± 0.09a |
3.67 ± 0.09a |
3.79 ± 0.13ab |
3.94 ± 0.10b |
3.97 ± 0.09b |
pro |
4.56 ± 0.09a |
4.58 ± 0.11a |
4.8 ± 0.09b |
4.63 ± 0.08a |
4.61 ± 0.08a |
trpa |
— |
— |
— |
— |
— |
TAA |
76.11 ± 0.16a |
80.58 ± 0.10b |
85.41 ± 0.57c |
89.93 ± 0.15d |
93.54 ± 0.22e |
TEAA |
31.49 ± 0.72a |
31.65 ± 0.80a |
32.30 ± 0.68ab |
32.50 ± 0.59ab |
33.14 ± 0.54b |
THAA |
33.57 ± 0.72a |
34.3 ± 0.64ab |
34.45 ± 0.64ab |
34.7 ± 0.53 ab |
35.25 ± 0.50b |
TAAA |
64.26 ± 0.72a |
64.58 ± 0.80a |
65.20 ± 0.68ab |
65.15 ± 0.59ab |
66.19 ± 0.54b |
The essential amino acids (EAA), His, Thr, Val, Met, Ile, Leu and Lys, are required by the FAO/WHO for growing children or adults.54 The content of EAA in RPs was significantly increased by irradiation, with the highest proportion of 33.14 ± 0.54% at 30 kGy, compared to 31.49 ± 0.72% at 0 kGy (Table 1). This result agrees with the findings of Sattar et al., who reported increases in essential amino acids of soybean at a dose of 0.10 kGy.55 Therefore, it is reasonable to believe that irradiated RPHs with a high content of EAA may serve as highly nutritional fortifiers.
The hydrophobic amino acids (HAA) increased gradually by 5.01%, with increasing irradiation doses, when pretreated with EBI at 30 kGy compared to the unpretreated RPs (Table 1). HAA can improve the ability of peptide to capture free radicals and thus affect the antioxidant activity. When the N-terminal is a hydrophobic amino acid, such as leucine, the antioxidant activity of peptides is higher.56 In this study, the improvement in HAA concentration confirmed that EBI caused unfolding of RPHs and improved the antioxidant activity of RPHs.
It is known that amino acids play a critical role in the antioxidant properties of hydrolysates. As shown in Table 1, RPHs are rich in Asp, Glu, Arg, Phe, Pro, and Leu, which have been reported to show strong antioxidant activity.8 EBI pretreatment of RPs facilitated the release of total antioxidant-related amino acid (TAAA) content, in contrast to that of the non-irradiated sample. The higher content of antioxidant-related amino acids could be responsible to the improved scavenging ability of irradiated RPHs (Fig. 2 and 3).
3.4.5 Molecular weight (MW) distribution of RPHs.
Molecular weight distribution is another important factor affecting the antioxidant capacity of proteins and peptides, and to some extent, it can reflect the degree of protein hydrolysis. Peng et al. found that the antioxidant activity of whey protein hydrolysates depended on peptide molecular weight.57 Xie et al. reported that peptides from alfalfa leaf protein hydrolysates with MW of <1000 Da possessed stronger antioxidant activity than higher MW peptides.58 It can be presumed that smaller peptides with low molecular weights have better biological activity. In the present study, the molecular weight distribution profile of RPHs prepared with different irradiation doses was analyzed, and the results are displayed in Table 2. The molecular weight distributions of RPHs were concentrated in the <1000 Da range. Moreover, EBI significantly increased the content of low molecular weight peptides. For non-irradiate sample, the proportion of 1000–500 Da and <500 Da fractions were 29.01% and 48.07%, respectively. Electron beam irradiation at 30 kGy generated smaller peptides, with 16.88% and 70.99% of the peptides falling in the range of 1000–500 Da and <500 Da, respectively. In addition, the fraction of <180 Da peptides, which consisted mainly of free amino acids, became larger as irradiation doses increased. These results showed that EBI caused RPs to be easily broken by protease and produced small peptides or even free amino acids, thus enhancing the antioxidant activity of RPHs.
Table 2 Molecular weight distribution profiles of RPHs irradiated at 0, 5, 10, 20 and 30 kGy
|
Percentage of RPHs fractions (%) |
>3 kDa |
2–3 kDa |
1–2 kDa |
0.5–1 kDa |
180–500 Da |
<180 Da |
0 kGy |
7.74 ± 0.16a |
6.19 ± 0.24a |
8.99 ± 0.14a |
29.01 ± 0.35a |
44.06 ± 0.12a |
4.01 ± 0.14a |
5 kGy |
5.6 ± 0.16b |
5.87 ± 0.19a |
8.58 ± 0.04ab |
26.96 ± 0.13b |
47.57 ± 0.14b |
5.42 ± 0.04b |
10 kGy |
4.44 ± 0.11c |
5.01 ± 0.29b |
8.16 ± 0.15b |
21.83 ± 0.15c |
54.2 ± 0.17c |
6.36 ± 0.15c |
20 kGy |
2.41 ± 0.24c |
5.02 ± 0.51b |
7.48 ± 0.56c |
18.09 ± 0.55d |
59.83 ± 0.45d |
7.17 ± 0.12d |
30 kGy |
2.07 ± 0.76d |
3.68 ± 0.75bc |
6.38 ± 0.57 |
16.88 ± 0.24de |
62.26 ± 0.65e |
8.73 ± 0.14e |
4. Conclusion
In this study, electron beam irradiation was used as a pre-processing method to improve the efficiency of peptide production and the antioxidant activity of hydrolysates. The DH of RPs treated with EBI (30 kGy) and alcalase was increased from 21.80% to 25.09%. Moreover, RPHs pretreated with EBI possessed an enhanced DPPH and ABTS+ radical scavenging capacity and cellular antioxidant activity than the non-irradiated RPHs. The improved antioxidant activity of RPHs may be attributed to the fact that EBI made RPs more accessible to protease action and produced smaller peptides. On the other hand, both technologies (EBI and enzyme hydrolysis) have been applied at industrial level and it is feasible to perform these procedures sequentially. Therefore, EBI treatment before enzymatic hydrolysis has the potential to become a novel hydrolysis process for preparation of bioactive hydrolysates.
Conflicts of interest
There are no conflicts to declare.
Acknowledgements
Financial support for this research was provided by National Natural Science Foundation of China (No. 31471616), National Key R&D Program of China (2017YFD0401200), National Top Youth Talent for Grain Industry of China (LQ2016301) and National First-Class Discipline Program of Food Science and Technology (JUFSTR20180203).
References
- M. Shibasaki, S. Suzuki, H. Nemoto and T. Kuroume, Allergenicity and lymphocyte-stimulating property of rice protein, J. Allergy Clin. Immunol., 1979, 64, 259–265 CrossRef CAS.
- F. F. Shih, An update on the processing of high-protein rice products, Mol. Nutr. Food Res., 2003, 47, 420–424 Search PubMed.
- Q. Zhao, H. Xiong, C. Selomulya, X. D. Chen, H. Zhong, S. Wang, W. Sun and Q. Zhou, Enzymatic hydrolysis of rice dreg protein: Effects of enzyme type on the functional properties and antioxidant activities of recovered proteins, Food Chem., 2012, 134, 1360–1367 CrossRef CAS PubMed.
- J.-s. Wang, M.-m. Zhao, Q.-z. Zhao and Y.-m. Jiang, Antioxidant properties of papain hydrolysates of wheat gluten in different oxidation systems, Food Chem., 2007, 101, 1658–1663 CrossRef CAS.
- M. Memarpoor-Yazdi, H. Mahaki and H. Zare-Zardini, Antioxidant activity of protein hydrolysates and purified peptides from Zizyphus jujuba fruits, J. Funct. Foods, 2013, 5, 62–70 CrossRef CAS.
- H. Agrawal, R. Joshi and M. Gupta, Isolation and characterisation of enzymatic hydrolysed peptides with antioxidant activities from green tender sorghum, Lebensm.-Wiss. Technol., 2017, 84, 608–616 CrossRef CAS.
- J. G. D. S. Aguilar and H. H. Sato, Microbial proteases: Production and application in obtaining protein hydrolysates, Food Res. Int., 2018, 103, 253–262 CrossRef CAS PubMed.
- X. Zhang, L. Wang, R. Wang, X. Luo, Y. Li and Z. Chen, Protective effects of rice dreg protein hydrolysates against hydrogen peroxide-induced oxidative stress in HepG-2 cells, Food Funct., 2016, 7, 1429–1437 RSC.
- Y. Jin, R. Liang, J. Liu, S. Lin, Y. Yu and S. Cheng, Effect of structure changes on hydrolysis degree, moisture state, and thermal denaturation of egg white protein treated by electron beam irradiation, LWT–Food Sci. Technol., 2017, 77, 134–141 CrossRef CAS.
- H. Zhang, H. Ma, W. Liu, J. Pei, Z. Wang, H. Zhou and J. Yan, Ultrasound enhanced production and antioxidant activity of polysaccharides from mycelial fermentation of Phellinus igniarius, Carbohydr. Polym., 2014, 113, 380–387 CrossRef CAS PubMed.
- Z. Cheng, Y. Yang, Y. Liu, Z. Liu and H. Hu, Optimization of microwave-assisted enzymatic extraction of polysaccharides from the fruit of Schisandra chinensis Bail, Int. J. Biol. Macromol., 2015, 76, 161–168 CrossRef CAS PubMed.
- M. M. D. S. Moretti, D. A. B. Souza, B. Martins, C. D. C. C. Nunes, M. A. Villena, O. M. Perrone, R. d. Silva, M. Boscolo and E. Gomes, Pretreatment of sugarcane bagasse with microwaves irradiation and its effects on the structure and on enzymatic hydrolysis, Appl. Energy, 2014, 122, 189–195 CrossRef CAS.
- S. Jin, G. Zhang, P. Zhang, S. Fan and F. Li, High-pressure homogenization pretreatment of four different lignocellulosic biomass for enhancing enzymatic digestibility, Bioresour. Technol., 2015, 181, 270–274 CrossRef CAS PubMed.
- P. Garcia-Mora, E. Peñas, J. Frias, R. Gomez and C. Martinez-Villaluenga, High-pressure improves enzymatic proteolysis and the release of peptides with angiotensin I converting enzyme inhibitory and antioxidant activities from lentil proteins, Food Chem., 2015, 171, 224–232 CrossRef CAS PubMed.
- J. Farkas, Irradiation for better foods, Trends Food Sci. Technol., 2006, 17, 148–152 CrossRef CAS.
- P. Supriya, K. R. Sridhar, S. Nareshkumar and S. Ganesh, Impact of Electron Beam Irradiation on Fatty Acid Profile of Canavalia Seeds, Food Bioprocess Technol., 2012, 5, 1049–1060 CrossRef CAS.
-
WHO, High-dose irradiation: Wholesomeness of food irradiated with doses above 10 kGy, World Health Organization, Geneva (WHOTechnical Report Series 890), 1999.
- Â. Fernandes, J. C. Barreira, A. L. Antonio, A. Rafalski, M. B. Oliveira, A. Martins and I. C. Ferreira, How does electron beam irradiation dose affect the chemical and antioxidant profiles of wild dried Amanita mushrooms?, Food Chem., 2015, 182, 309–315 CrossRef PubMed.
- R. Bhat and K. R. Sridhar, Nutritional quality evaluation of electron beam-irradiated lotus (Nelumbo nucifera) seeds, Food Chem., 2008, 107, 174–184 CrossRef CAS.
- J. Farkas and C. Mohacsifarkas, History and future of food irradiation, Trends Food Sci. Technol., 2011, 22, 121–126 CrossRef CAS.
- Y. H. Kuan, R. Bhat, A. Patras and A. A. Karim, Radiation processing of food proteins – A review on the recent developments, Trends Food Sci. Technol., 2013, 30, 105–120 CrossRef CAS.
- M. H. Gaber, Effect of gamma-irradiation on the molecular properties of bovine serum albumin, J. Biosci. Bioeng., 2005, 100, 203–206 CrossRef CAS PubMed.
- R. Bhat, K. R. Sridhar and K. Tomitayokotani, Effect of ionizing radiation on antinutritional features of velvet bean seeds (Mucuna pruriens), Food Chem., 2007, 103, 860–866 CrossRef CAS.
- L. Wang, X. Zhang, F. Liu, Y. Ding, R. Wang, X. Luo, Y. Li and Z. Chen, Study of the functional properties and anti-oxidant activity of pea protein irradiated by electron beam, Innovative Food Sci. Emerging Technol., 2017, 41, 124–129 CrossRef CAS.
- B. Min, K. C. Nam, C. Jo and D. U. Ahn, Irradiation of shell egg on the physicochemical and functional properties of liquid egg white, Poult. Sci., 2012, 91, 2649 CrossRef CAS PubMed.
- S. F. Sabato, N. Nakamurakare and P. J. A. Sobral, Mechanical and thermal properties of irradiated films based on Tilapia (Oreochromis niloticus) proteins, Radiat. Phys. Chem., 2007, 76, 1862–1865 CrossRef CAS.
- A. Akbarian, M. Khorvash, G. R. Ghorbani, E. Ghasemi, M. Dehghan-Banadaky, P. Shawrang and M. H. Ghaffari, Effects of roasting and electron beam irradiating on protein characteristics, ruminal degradability and intestinal digestibility of soybean and the performance of dairy cows, Livest. Sci., 2014, 168, 45–52 CrossRef.
- H. J. Kim, J. I. Choi, D. J. Kim, J. H. Kim, B. S. Chun, D. H. Ahn, H. S. Yook, M. W. Byun, M. J. Kim, M. G. Shin and J. W. Lee, Effect of ionizing radiation on the physiological activities of ethanol extract from hizikia fusiformis cooking drips, Appl. Radiat. Isot., 2009, 67, 1509–1512 CrossRef CAS PubMed.
- M. Taghinejad-Roudbaneh, S. R. Ebrahimi, S. Azizi and P. Shawrang, Effects of electron beam irradiation on chemical composition, antinutritional factors, ruminal degradation and in vitro protein digestibility of canola meal, Radiat. Phys. Chem., 2010, 79, 1264–1269 CrossRef CAS.
- S. Y. Lin, K. Wang and J. B. Liu, Improving effect of enzyme hydrolysis of corn gluten meal by assistant of electron beam irradiation, Trans. Chin. Soc. Agric. Eng., 2014, 30, 300–308 CAS.
- M. Ledoux and F. Lamy, Determination of proteins and sulfobetaine with the folin-phenol reagent, Anal. Biochem., 1986, 157, 28–31 CrossRef CAS PubMed.
- K. Zhou, J. J. Yin and L. L. Yu, Phenolic acid, tocopherol and carotenoid compositions, and antioxidant functions of hard red winter wheat bran, J. Agric. Food Chem., 2005, 53, 3916–3922 CrossRef CAS PubMed.
- D. Dany, D. E. Otter, S. F. Gauthier and F. E. Allen, Enzyme-induced gelation of extensively hydrolyzed whey proteins by Alcalase: peptide identification and determination of enzyme specificity, J. Agric. Food Chem., 2003, 51, 6300–6308 CrossRef PubMed.
-
R. K. Murray, D. K. Granner, P. A. Mayes and V. W. Rodwell, Harper's Biochemistry, 26th ed, McGraw-Hill, New York, NY, USA, 2003 Search PubMed.
- A. Kumaran and R. J. Karunakaran, Antioxidant and free radical scavenging activity of an aqueous extract of Coleus aromaticus, Food Chem., 2006, 97, 109–114 CrossRef CAS.
- N. S. S. Kumar, R. A. Nazeer and R. Jaiganesh, Purification and biochemical characterization of antioxidant peptide
from horse mackerel (Magalaspis cordyla) viscera protein, Peptides, 2011, 32, 1496–1501 CrossRef PubMed.
- J.-i. Choi, H.-J. Kim, J.-H. Kim, B. S. Chun, D. H. Ahn, G. H. Kim and J.-W. Lee, Changes in colour and antioxidant activities of Hizikia fusiformis cooking drips by gamma irradiation, LWT–Food Sci. Technol., 2010, 43, 1074–1078 CrossRef CAS.
- L. Wang, T. Li, D. L. Sun, M. Q. Tang, Z. Sun, L. F. Chen, X. H. Luo, Y. F. Li, R. Wang, Y. N. Li, J. Li and Z. X. Chen, Effect of electron beam irradiation on the functional properties and antioxidant activity of wheat germ protein hydrolysate, Innovative Food Sci. Emerging Technol., 2018 DOI:10.1016/j.ifset.2018.09.003.
- Â. Fernandes, J. C. M. Barreira, A. L. Antonio, M. B. P. P. Oliveira, A. Martins and I. C. F. R. Ferreira, Feasibility of electron-beam irradiation to preserve wild dried mushrooms: Effects on chemical composition and antioxidant activity, Innovative Food Sci. Emerging Technol., 2014, 22, 158–166 CrossRef.
- K. L. Wolfe and L. R. Hai, Cellular antioxidant activity (CAA) assay for assessing antioxidants, foods, and dietary supplements, J. Agric. Food Chem., 2007, 55, 8896–8907 CrossRef CAS PubMed.
- D. L. Felice, S. Jie and H. L. Rui, A modified methylene blue assay for accurate cell counting, J. Funct. Foods, 2009, 1, 109–118 CrossRef.
- H.-C. Wu, H.-M. Chen and C.-Y. Shiau, Free amino acids and peptides as related to antioxidant properties in protein hydrolysates of mackerel (Scomber austriasicus), Food Res. Int., 2003, 36, 949–957 CrossRef CAS.
- M. C. Lv, K. L. Mei, H. Zhang, D. Xu and W. Yang, Effects of electron beam irradiation on the biochemical properties and structure of myofibrillar protein from Tegillarca granosa meat, Food Chem., 2018, 254, 64–69 CrossRef CAS PubMed.
- A. M. Malik, H. K. Sharma and C. S. Saini, Effect of gamma irradiation on structural, molecular, thermal and rheological properties of sunflower protein-isolate, Food Hydrocolloids, 2017, 72, 312–322 CrossRef.
- S. Moon and K. B. Song, Effect of γ-irradiation on the molecular properties of ovalbumin and ovomucoid and protection by ascorbic acid, Food Chem., 2001, 74, 479–483 CrossRef CAS.
- J. Jian, H. Ma, W. Kai, E. G. A. Yagoub, J. Owusu, W. Qu, R. He, C. Zhou and X. Ye, Effects of multi-frequency power ultrasound on the enzymolysis and structural characteristics of corn gluten meal, Ultrason. Sonochem., 2015, 24, 55–64 CrossRef PubMed.
-
A. Fersht, Structure and Mechanism in Protein Science: A Guide to Enzyme Catalysis and Protein Folding, Macmillan, 1999 Search PubMed.
- R. Nie, Y. Liu and Z. Liu, The calcium-binding activity of fish scale protein hydrolysates, J. Agric. Chem. Environ., 2014, 03, 11–15 Search PubMed.
- C. Zhou, H. Ma, Q. Ding, L. Lin, X. Yu, L. Luo, C. Dai and E. G. A. Yagoub, Ultrasonic pretreatment of corn gluten meal proteins and neutrase: Effect on protein conformation and preparation of ACE (angiotensin converting enzyme) inhibitory peptides, Food Bioprod. Process., 2013, 91, 665–671 CrossRef CAS.
- R. Lange and C. Balny, UV-visible derivative spectroscopy under high pressure, Biochim. Biophys. Acta, 2002, 1595, 80–93 CrossRef CAS.
- D. C. F. Lopes, F. M. Delvivo and M. P. C. Silvestre, Use of activated carbon for removing phenylalanine from reconstituted skim milk powder hydrolysates, LWT–Food Sci. Technol., 2005, 38, 447–453 CrossRef CAS.
- I. O. Olotu, V. Enujiugha and A. O. Obadina, The Effect of γ-Irradiation and Cooking on the Amino Acid Profile of African Oil Bean Seed (Pentaclethra macrophylla Benth), J. Food Process. Preserv., 2014, 38, 2020–2026 CrossRef CAS.
-
P. S. Elias and A. J. Cohen, Radiation chemistry of proteins, in Radiation Chemistry of Major Food Components, Elsevier Scientific, Amsterdam, The Netherlands, 2nd edn, 1977, pp. 33–34 Search PubMed.
- T. Wang, R. Wang, L. Wang, H. Zhang and Z. X. Chen, Solubilization by freeze-milling of water-in-soluble subunits in rice proteins, Food Funct., 2015, 6, 428–430 Search PubMed.
- A. Sattar, Neelofar and M. A. Akhtar, Irradiation and germination effects on phytate, protein and amino acids of soybean, Plant Foods Hum. Nutr., 1990, 40, 185–194 CrossRef CAS PubMed.
-
Z. Guichun, The research of UHP treatment on rice residue protein hydrolysate antioxidant activity, Central South University of Forestry and Technology, 2016 Search PubMed.
- X. Peng, Y. L. Xiong and B. Kong, Antioxidant activity of peptide fractions from whey protein hydrolysates as measured by electron spin resonance, Food Chem., 2009, 113, 196–201 CrossRef CAS.
- Z. Xie, J. Huang, X. Xu and Z. Jin, Antioxidant activity of peptides isolated from alfalfa leaf protein hydrolysate, Food Chem., 2008, 111, 370–376 CrossRef CAS PubMed.
|
This journal is © The Royal Society of Chemistry 2020 |
Click here to see how this site uses Cookies. View our privacy policy here.