DOI:
10.1039/C9GC03393A
(Paper)
Green Chem., 2020,
22, 180-186
A tannin-derived zirconium-containing porous hybrid for efficient Meerwein–Ponndorf–Verley reduction under mild conditions†
Received
29th September 2019
, Accepted 20th November 2019
First published on 22nd November 2019
Abstract
Both the use of renewable natural sources to prepare catalytic materials and the Meerwein–Ponndorf–Verley (MPV) reduction for carbonyl compounds are very attractive topics in catalysis. In this study, tannins were simply assembled with zirconium in water for the scalable preparation of a heterogeneous zirconium–tannin hybrid catalyst (Zr-tannin). Various characterizations demonstrated the formation of robust porous inorganic–organic frameworks and strong Lewis acid–base sites in Zr-tannin. The cooperative effect of these acid–base sites and the abundant porosity endowed Zr-tannin with a remarkable catalytic performance for the MPV reduction of a broad range of carbonyl compounds to alcohols with 2-propanol under mild conditions. Moreover, Zr-tannin exhibited good recyclability for at least five reaction cycles. This novel strategy using tannins as the raw materials to construct heterogeneous catalytic materials may have a huge potential for green chemical synthesis due to low cost, nontoxicity, and sustainability.
Introduction
The Meerwein–Ponndorf–Verley (MPV) reaction is an ideal reduction technique for carbonyl compounds because of using abundant and safe secondary alcohols as H-donor instead of dangerous gaseous hydrogen.1–3 Typically, the selective hydrogenation of α,β-unsaturated aldehydes is of great importance in the production of unsaturated alcohols, which have been widely used in flavouring, perfume, and pharmaceutical industries.4,5 During the past decade, diverse catalysts based on metal oxides, metal alloys, hydroxides, metal–organic frameworks (MOF), and zeolites have been employed for MPV reductions.6–12 Among these catalysts, zirconium (Zr)-containing catalysts have been proven to be very effective for the reduction of carbonyl compounds.13–17 However, most of them often suffer from expensive raw materials, complex preparation processes, harsh reaction conditions (high reaction temperatures or long reaction times), and poor selectivity and stability. Therefore, the development of cost-effective, sustainable, and more efficient Zr-based catalysts for MPV reduction is desirable and imperative.
The use of renewable natural sources to synthesize functional materials has been recognized as a crucial research area.18–20 The assembly of natural compounds, such as polyphenols, lignins, porphyrins, phytic acid, and furan derivatives with various metal ions is able to generate active solid catalysts for heterogeneous catalysis.21–24 Through this method, several metal-based organic hybrids like Zr-LS, Zr-PhyA, and FDCA-Hf have been developed and used as solid catalysts for MPV reduction.25–32 The results indicate that via acid–base cooperative catalysis between metal ions and organic compounds, high activity and selectivity can be achieved. Inspired by these useful examples, also taking into account that the diversity of natural compounds provides many possibilities for improving the catalytic performance,25,28,29,33 our attention was focused on the construction of efficient Zr-containing solid catalysts from natural resources for MPV reduction.
Tannins obtained from the seeds and barks of plants are abundant biomass fractions in nature, which have been widely used in medicine, food, organic synthesis, and polymeric materials.34–37 Especially, due to the presence of abundant phenolic hydroxy (Ar–OH) groups, tannins can easily coordinate with metal ions to form insoluble M–tannin hybrids.21,36,38–40 This unique feature makes tannins an ideal adsorbent in the field of water treatment.35,41 Despite the versatile applications of tannins in many functional materials and environmental areas, quite surprisingly, their use as a building block for metal–organic hybrid catalyst synthesis has been seldom explored. Herein, we prepared a Zr-tannin porous catalyst simply by the chelation of Zr4+ and tannins. The Zr-tannin was explored to be a highly active and stable solid catalyst for the MPV reduction of various carbonyl compounds to alcohols with 2-propanol (2-PrOH) as the hydrogen source. Extensive characterizations were performed to investigate the relationship between the catalytic active sites and its catalytic performance. The results demonstrated that the porous organic–inorganic networks and acid–base synergistic sites in Zr-tannin may be responsible for the excellent catalytic performance.
Experimental section
Reagents and analyses
All chemicals were of analytical grade and used as received. Thermogravimetric (TG) analysis was performed using a STA409 instrument at a heating rate of 10 °C min−1 in nitrogen flow from 30 to 800 °C. Fourier infrared (FT-IR) spectra were recorded on a Nicolet 360 FT-IR instrument (KBr. discs) in the 4000–400 cm−1 region. Diffuse reflectance UV–vis spectra of solid samples were obtained on an UV-2550 spectrophotometer using barium sulfate as background. The nitrogen adsorption/desorption isotherms and pore size distribution curves were measured at the temperature of liquid nitrogen (77 K) using a BELSORP-MINI analyzer. The element distribution was characterized by Hitachi S-4800 field emission scanning electron microscope coupled with an energy-dispersive X-ray spectrometer. The Zr content in Zr-tannin was measured using a Jarrell-Ash 1100 ICP-AES spectrometer. X-ray photoelectron spectroscopy (XPS) was conducted on a PHI 5000 Versa Probe X-ray photoelectron spectrometer equipped with Al Kα radiation (1486.6 eV). X-ray diffraction (XRD) patterns were collected on a Bruker D8 Advance powder diffractometer using Ni-filtered Cu/Kα radiation. Water repellency was tested by the contact angle of the substrate droplet using a contact angle meter of OCA 40. The acidity and basicity of the samples were determined through temperature-programmed desorption of NH3-TPD and CO2-TPD by a Micromeritics Bel Cata II equipment. The sample was firstly degassed under flowing He at 150 °C for 2 h. After the sample was cooled to ambient temperature, 10 vol% CO2 or NH3 in Ar (50 mL min−1) was adsorbed onto the solid sample at 50 °C for 60 min, followed by purging with He, and then the mass signals were recorded by performing CO2 or NH3 desorption from 50 °C to 250 °C. The Lewis and Brønsted acid sites of Zr-tannin were measured by pyridine adsorption FT-IR fitted with a Bruker VERTEX V70v system in the wavenumber range of 1400–1700 cm−1. The oven-dried sample (25 mg) was pressed into a 13 mm self-supported wafer and activated in the IR cell at 150 °C for 3 h. After it was cooled to room temperature, the sample was contacted with pyridine vapor under vacuum for 1 h, which was followed by the evacuation of excess pyridine. Then, the cell was kept in a vacuum oven at 150 °C/250 °C for 1 h to remove the physisorbed pyridine. Based on the following equations, the concentration of Brønsted (CB (μmmol g−1)) and Lewis acid (CL (μmmol g−1)) sites was calculated.16,42
CB (μmol g−1) = (1.88 × IAB × R2)/W |
CL (μmol g−1) = (1.42 × IAL × R2)/W |
where IAB and IAL stand for the absorbance peak area at 1450 cm−1 for Brønsted acid sites and peak area at 1540 cm−1 for Lewis acid sites, respectively; R stands for the radius of the self-supported disk; W stands for the sample weight.
Preparation of catalysts
In a typical procedure for Zr-tannin preparation, tannins (1.0 g, 0.6 mmol) were dissolved in 30 mL deionized water in a 100 mL round-bottomed flask. The aqueous solution was heated to 80 °C in an oil bath, and then an aqueous solution of ZrCl4 (1.5 g ZrCl4 in 20 mL deionized water) was added dropwise into it. The milky white mixture was stirred at 80 °C for 12 h. The thus obtained solid was filtered and washed with water several times, and subsequently dried in a vacuum at 70 °C overnight to give the final catalyst (Zr-tannin) with a weight of about 0.85 g.
Catalytic tests
The MPV reduction of various carbonyl compounds using 2-PrOH as hydrogen-donor was carried out in a 35 mL pressure tube. In a typical reaction procedure, furfural (1 mmol), 2-PrOH (10 mL), and the catalyst (0.1 g) were added into the reactor and tightly sealed, and it was followed by heating to 80 °C. After magnetically stirring for 3 h, the solid catalyst was removed by centrifugation and the liquid was quantitatively analyzed by gas chromatography. The recovered catalyst could be directly reused for the next cycle under identical reaction conditions. In the leaching experiment, the catalyst was filtered out form the reaction system after reacting for 60 min. Then, the reaction was allowed to proceed for another 120 min without the catalyst. The Zr leached into the reaction solution was determined by ICP-AES, and the recovered catalyst was analyzed by FT-IR.
Results and discussion
The fabrication process for Zr-tannin only involves a facile and nontoxic one-step assembly synthesis in water (Scheme 1). Tannins dissolved in water and coordinated rapidly with Zr4+ to form insoluble white precipitates (Zr-tannin) (Fig. S1†), which were then filtered out, dried, and extensively characterized by SEM, FT-IR, XRD, N2 adsorption–desorption analysis, ICP-AES, and TGA. The SEM image of Zr-tannin presents an amorphous connected nanoparticle structure (Fig. 1A), and the EDS mapping images demonstrate the homogeneous dispersion of Zr, C, and O elements throughout the Zr-tannin hybrid (Fig. 1B). The XRD pattern of ZrCl4 exhibits clear diffraction peaks, but all these peaks have disappeared for Zr-tannin (Fig. 1C), suggesting the irregular connectivity between Zr4+ and tannins and the amorphous structure of Zr-tannin, which is unlike ZrO2 with high crystallinity (Fig. S2†). The Zr content in Zr-tannin was determined to be 22.3 wt% based on the ICP-AES result, indicating a high load capacity of tannins for metal ions. The TG profile indicated that Zr-tannin was stable up to 250 °C (Fig. S3†). In addition, it was observed to be insoluble in nonpolar and most polar solvents. These properties enable Zr-tannin to be a solid catalyst in liquid-phase reactions.
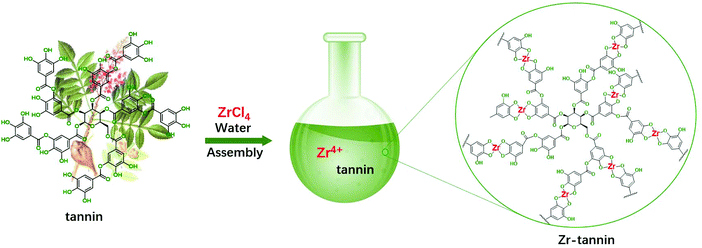 |
| Scheme 1 The preparation process of Zr-tannin using tannin as the building block and its plausible structure. | |
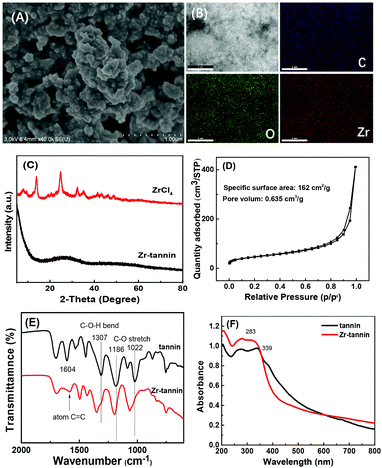 |
| Fig. 1 (A) SEM image and (B) EDS mapping images of Zr-tannin; (C) XRD patterns of ZrCl4 and Zr-tannin; (D) N2 adsorption/desorption isotherms of Zr-tannin; (E) FT-IR spectra and (F) UV–vis spectra of tannin and Zr-tannin. | |
The building block tannin is a nonporous material. In contrast, the N2 adsorption/desorption isotherms of Zr-tannin displayed a IV-type isotherm with an obvious H3-hysteresis loop (Fig. 1D), indicating that Zr-tannin is a mesoporous material. The specific surface area and pore volume were measured to be 162 m2 g−1 and 0.635 cm3 g−1, respectively. This result suggests that the assembly process between tannins and Zr4+ can promote the formation of porous organic–inorganic networks.
The FT-IR spectrum of tannins displayed the typical vibration peaks for –Ar–OH bending (1307 cm−1), –C–O– stretching (1186 cm−1, 1022 cm−1), and aromatic C
C stretching (1604 cm−1). Notably, for Zr-tannin, the vibrations at 1307 cm−1, 1186 cm−1, and 1022 cm−1 shifted to higher wave-numbers of 1353 cm−1, 1197 cm−1, 1060 cm−1, respectively, and the intensity of the band for aromatic C
C was remarkably decreased as compared with that of tannins (Fig. 1E). The observations indicated the possible coordination between Zr4+ and –Ar–OH that caused the formation of –Ar–O2–Zr–O2–Ar– frameworks. UV–vis spectroscopy was further performed to confirm the coordination between Zr4+ and tannins (Fig. 1F). Tannins exhibited absorption between 250 and 450 nm with a maximum at 350 nm. In the case of Zr-tannin, these absorption bands were blue shifted with the maximum absorption peak at 280 nm.
Catalytic performance
For the initial catalytic tests, we selected the MPV reduction of furfural (FF) to furfuryl alcohol (FA) with 2-PrOH as the probe reaction to study the catalytic performance of Zr-tannin and control catalysts at 80 °C (Fig. 2A). The reaction did not proceed at all without a catalyst or with tannins as the catalyst. When Zr-tannin was used as the catalyst, a solid–liquid heterogeneous catalysis was observed and a high conversion of 96.2% with 95.0% selectivity was achieved. Even with a lower reaction temperature of 70 °C or less catalyst amount of 80 mg, considerable activity (conversion >70%) was obtained (Fig. S4†). In contrast, ZrCl4 and ZrOCl2 induced a homogeneous system but caused low conversions of about ∼5%–6% with ∼23%–25% selectivity. ZrO2 and Zr(C5H7O4)4 presented slightly higher conversions (∼25%–35%) but caused very low selectivity (∼1%–3%). MOFs are a class of popular catalytic materials that have been demonstrated to be highly active in many organic transformations.43–45 Accordingly, two common Zr-based MOFs (UiO-66 and UiO-66-(OH)2 were prepared by assembling benzene dicarboxylic acid and 2,5-dihydroxyterephthalic acid46 and used as control catalysts for the reduction of FF. As can be seen, UiO-66 and UiO-66-(OH)2 gave 9.2% conversion with 34.1% selectivity and 23.2% conversion with 8.3% selectivity, respectively, which were much lower than that of Zr-tannin, indicating the superior performance of Zr-tannin hybrid.
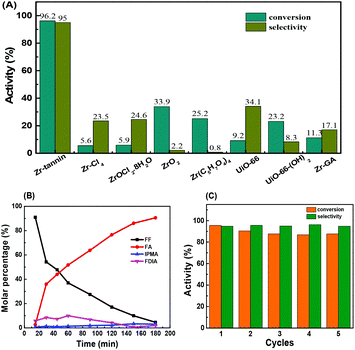 |
| Fig. 2 (A) The catalytic performance of various catalysts for the MPV reduction of FF to FA with 2-PrOH; (B) the product distribution during the MPV reduction over Zr-tannin; (C) the reusability of Zr-tannin for the MPV reduction of FF. Reaction conditions: Furfural 1 mmol, 2-PrOH 10 mL, Zr-tannin 100 mg, 80 °C, 3 h. | |
The time course of FF reduction over Zr-tannin was recorded at 80 °C (Fig. 2B). The product distribution demonstrates that FF was almost completely transformed into FA in 3 h. During this process, furfural dissopropyl acetal (FDIA) was observed probably due to the acetalization of FF with 2-PrOH. Notably, FDIA only existed for a short reaction time and converted to FF with the increase in the reaction time. When the reaction time was prolonged to 3 h, 2-(isopropoxymethyl)-furan (IPMA) as a by-product was formed and the FA selectivity decreased slightly. This phenomenon is consistent with the previously reported product distribution in the MPV reduction of FF.28,47
As for the durability tests, Zr-tannin exhibited good recyclability for the reduction of FF in a five-run test, only a slight decrease in conversion was observed in the first two cycles (Fig. 2C). The recovered Zr-tannin illustrated a similar FT-IR spectrum to that of the fresh one (Fig. S5†). Moreover, the concentration of Zr was lower than 2 ppm in the reaction solution as detected by ICP-AES, suggesting the negligible leaching of active sites. The results of the thermal filtration experiment demonstrate that the reaction was completely stopped after the removal of the catalyst from the reaction mixture at 60 min reaction time (Fig. S6†), further confirming the heterogeneous nature and high durability of Zr-tannin for MPV reduction.
We compared Zr-tannin catalyst for the MPV reduction of FF with other previously reported catalysts in literatures (Table S1†). The catalytic activity and selectivity of Zr-tannin are much higher than those of FDCA-Hf, Mn-NCA-700, Fe-L1/C-800, and NiO(P)-300. Zr-Based catalysts (such as Zr-PW, PhP-Zr, Zr-GA, Zr-PN, Zr-RSL, Zr-PhyA, Zr-LS, and Zr-HAs) can catalyze MPV reductions under milder conditions (80–120 °C). Compared with them, not only is our Zr-tannin comparable in activity, selectivity, and reusability, but also the preparation of the present catalyst is much simpler.
Delighted by the excellent performance of Zr-tannin for the MPV reduction of FF, we further investigated the catalytic activity of Zr-tannin for other carbonyl compounds (Table 1). As can be seen, Zr-tannin exhibited good activity and selectivity toward biomass-based carbonyl compounds, such as 5-methylfurfural and cinnamaldehyde, showing more than 90% conversions with higher than 95% selectivity to the corresponding alcohols (entries 2 and 3). Under proper rising reaction temperature (80–120 °C), other commercial carbonyl compounds, including benzaldehyde, salicylaldehyde, 4-hydroxybenzaldehyde, cyclohexanone, crotonaldehyde, and propanal were also successfully converted into the corresponding alcohols with both high conversions and selectivity (entries 4–9). In the case of acetophenone and ethyl levulinate, due to their higher electron density or steric hindrance, a higher reaction temperature of 150 °C and relatively longer reaction times (8–14 h) were needed to obtain satisfactory activity (entries 10 and 11). The above results indicate that the Zr-tannin hybrid was a highly versatile catalyst for the MPV reduction of a wide scope of substrates.
Table 1 Results of the Zr-tannin-catalyzed MPV reduction of various carbonyl compounds to alcohols
Entry |
Substrates |
Products |
Time (h) |
T (°C) |
Con. (%) |
Sel. (%) |
Reaction conditions: 1 mmol substrate in 10 mL 2-PrOH with 100 mg catalyst Zr-tannin. |
1 |
|
|
3 |
80 |
96 |
95 |
2 |
|
|
5 |
100 |
95 |
94 |
3 |
|
|
4 |
80 |
95 |
97 |
4 |
|
|
2 |
80 |
96 |
98 |
5 |
|
|
5 |
100 |
94 |
86 |
6 |
|
|
3 |
100 |
87 |
97 |
7 |
|
|
4 |
90 |
99 |
99 |
8 |
|
|
5 |
120 |
98 |
93 |
9 |
|
|
1.5 |
80 |
98 |
98 |
10 |
|
|
14 |
150 |
93 |
96 |
11 |
|
|
8 |
150 |
98 |
95 |
Understanding the catalytic performance
According to previous literatures, the acidic and basic sites are crucial for the MPV reaction.27,32,48,49 To gain more insight into the correlation between the acid/base properties and activity of Zr-tannin, we performed extensive characterizations, including NH3-TPD, CO2-TPD, and pyridine-adsorbed FT-IR to investigate the formation of strong acid–base catalytic sites in Zr-tannin. The acid/base properties of Zr-tannin and ZrO2 were first compared by NH3-TPD and CO2-TPD methods (Fig. 3A and B). No NH3/CO2 adsorption was observed for ZrO2, while a large amount of NH3/CO2 desorption and a longer desorption time suggested that Zr-tannin possessed high contents of both acidic sites and basic sites. This could be ascribed to the chelation between –Ar–OH groups and Zr4+ causing the formation of –Ar–O2–Zr–O2–Ar– frameworks that bare both acidic (Zr4+) and basic (–Ar–O2–) sites.22,28,50 Based on the quantitative analysis of the peak area, the amount of total acidic sites was calculated to be 0.114 mmol g−1, and the amount of basic sites was calculated to be 0.322 mmol g−1.
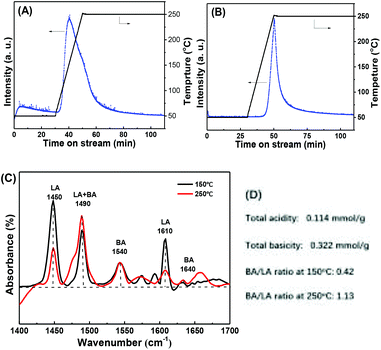 |
| Fig. 3 (A) NH3-TPD and (B) CO2-TPD spectra for ZrO2 and Zr-tannin; (C) pyridine-adsorbed FT-IR spectra of Zr-tannin; (D) the concentration of Brønsted and Lewis acid sites. | |
Pyridine-adsorbed FT-IR spectra were further obtained at two desorption temperatures of 150 °C and 250 °C to have a deep insight into the Lewis and Brønsted acidic sites of Zr-tannin (Fig. 3C). A series of bands occurred for Zr-tannin at both 150 °C and 250 °C detecting temperatures. These bands can be assigned to the pyridine interacting with the Lewis acid sites (LA) (1450 and 1610 cm−1), Brønsted acid sites (BA) (1540 and 1640 cm−1), or both of these two acid sites (1490 cm−1).25,26 Based on the equations in Experimental section, the Brønsted/Lewis acid ratio was calculated to be 0.42 and 1.13 at 150 °C and 250 °C, respectively (Fig. 3D). The LA sites are suggested to arise from Zr4+, and the BA sites are suggested to arise from the rest of the Ar–OH groups that don't have coordination with Zr in tannin. Acid sites are well known active sites for enhancing the adsorption and activation of substrates in MPV reduction.28,51 Thus, the moderate acidity in Zr-tannin should make the MPV reduction more favourable.
In the Zr 3d XPS spectra of Zr-tannin and ZrO2 (Fig. 4A), ZrO2 showed binding energies at 182.1 eV for Zr 3d5/2 and 184.4 eV for Zr 3d3/2. For Zr-tannin, these peaks shifted slightly to a higher level, the intensity of which decreased significantly in comparison with that of ZrO2. This observation can be attributed to a higher positive charge on Zr in Zr-tannin, manifesting a stronger Lewis acidity of Zr-tannin.25–29 Meanwhile, the higher O 1s binding energy of Zr-tannin as compared with that of ZrO2 assigned to a more negative charge on the oxygen atoms in Zr-tannin manifested a stronger basic strength of Zr-tannin (Fig. 4B).
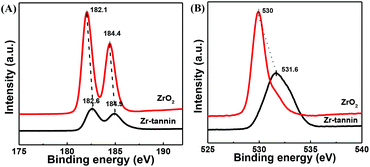 |
| Fig. 4 (A) Zr 3d XPS spectra; (B) O 1s XPS spectra of ZrO2 and Zr-tannin. | |
As discussed above, using tannins as the building block to chelate with Zr4+ can help form robust Ar–O2–Zr–O2–Ar frameworks with abundant acidic and basic sites, which seem to be key factors for the MPV reduction of carbonyl compounds to alcohols. Based on the experimental results in this work, and according to previous reports,25,26,30,31 we proposed a possible mechanism for the Zr-tannin-catalyzed MPV reaction (Scheme 2). 2-PrOH was first dissociated into alkoxide and hydrogen on the acidic–basic coupled sites (–Ar–O2–Zr–); meanwhile, the carbonyl group in the substrate was activated by electron-withdrawing Brønsted acid sites (–Ar–OH), forming the six-membered ring transition state. Next, the direct hydride transfer between carbonyl groups and 2-PrOH resulted in the generation of the corresponding alcohol and acetone.
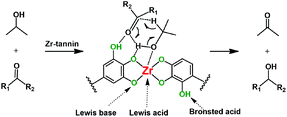 |
| Scheme 2 Possible mechanism for the Zr-tannin-catalyzed MPV reduction of carbonyl compounds with 2-PrOH. | |
The Zr-tannin possessed a high specific surface area of 162 m2 g−1. Moreover, it showed good wettability for substrates as demonstrated by contact angle tests (Fig. S7†). We thus proposed that apart from the acid–base synergistic active sites, the porous structure and surface wettability of Zr-tannin may also contribute to its high catalytic activity. In order to confirm this, we used gallic acid (the homologue of tannin) as the building block to prepare another control catalyst Zr-GA, which is a solid but non-porous material with a similar composition to that of Zr-tannin. However, Zr-GA displayed a much lower activity than that of Zr-tannin in the reduction of FF to FA, giving only 11.3% conversion and 17.1% selectivity (Fig. 2A). Therefore, the rich porosity and good hydrophilicity of Zr-tannin may greatly accelerate the mass transfer of substrates, which allows a fast reaction between substrates and the acid–base active sites.
Conclusions
In summary, we have demonstrated the successful application of the natural resource tannin as the building block for immobilizing Zr4+ catalyst via a facile one-step assembly method. The obtained Zr-tannin hybrid was found to be a highly efficient catalyst for MPV reductions using 2-PrOH as the hydrogen source under mild reaction conditions. Furthermore, Zr-tannin possessed stable recyclability and good universality for various substrates. The remarkable catalytic performance of Zr-tannin can be attributed to the acidic (originating from Zr4+) and basic (originating from –Ar–O2–) cooperative active sites, porous structure, and good wettability for reactants. The utilization of tannins as building blocks for both fabricating biomacromolecule catalytic materials and producing valuable platform chemicals presents a sustainable chemical conversion process.
Conflicts of interest
The authors declare no competing financial interest.
Acknowledgements
The authors thank the National Natural Science Foundation of China (no. 21978115), MOE & SAFEA for the 111 Project (No. B13025), and the Open Fund of Jiangsu Key Laboratory for Biomass Energy and Material (No: JSBEM201906). The authors were also supported by the Central Laboratory of School of Chemical and Material Engineering.
Notes and references
- D. A. Evans, S. G. Nelson, M. R. Gagne and A. R. Muci, J. Am. Chem. Soc., 1993, 115, 9800–9801 CrossRef CAS.
- T. Ooi, T. Miura and K. Maruoka, Angew. Chem., Int. Ed., 1998, 37, 2347–2349 CrossRef CAS.
- K. Nishide, Y. Shigeta, K. Obata and M. Node, J. Am. Chem. Soc., 1996, 118, 13103–13104 CrossRef CAS.
- H. Y. Liu, Q. Q. Mei, S. P. Li, Y. D. Yang, Y. Y. Wang, H. Z. Liu, L. R. Zheng, P. F. An, J. Zhang and B. X. Han, Chem. Commun., 2018, 54, 908–911 RSC.
- C. M. Piquerasa, V. Pucciaa, D. A. Vegab and M. A. Volpe, Appl. Catal., B, 2016, 185, 265–271 CrossRef.
- F. Gonell, M. Boronat and A. Corma, Catal. Sci. Technol., 2017, 7, 2865–2873 RSC.
- R. López-Asensioa, J. A. Ceciliaa, C. P. Jiménez-Gómeza, C. García-Sanchob, R. Moreno-Tosta and P. Maireles-Torres, Appl. Catal., A, 2018, 556, 1–9 CrossRef.
- J. N. Wei, X. J. Cao, T. Wang, H. Liu, X. Tang, X. H. Zheng, Y. Sun, T. Z. Lei, S. J. Liu and L. Lin, Catal. Sci. Technol., 2018, 8, 4474–4484 RSC.
- A. H. Valkar, K. H. Cho, S. K. Chitale, D. Y. Hong, G. Y. Cha, U. H. Lee, D. W. Hwang, C. Serre, J. S. Chang and Y. K. Hwang, Green Chem., 2016, 18, 4542–4552 RSC.
- S. Rojas-Buzo, P. Garcia-Garcia and A. Corma, ChemSusChem, 2018, 11, 432–438 CrossRef CAS.
- M. Koehle and R. F. Lobo, Catal. Sci. Technol., 2016, 33, 3018–3026 RSC.
- W. C. Yun, M. T. Yang and K. Y. Lin, J. Colloid Interface Sci., 2019, 543, 52–63 CrossRef CAS PubMed.
- V. L. Sushkevich, I. I. Ivanova, S. Tolborg and E. Taarning, J. Catal., 2014, 316, 121–129 CrossRef CAS.
- S. Song, L. Di, G. Wu, L. J. Guan and L. D. Li, Appl. Catal., B, 2017, 205, 393–403 CrossRef CAS.
- H. P. Winoto, Z. A. Fikri, J. M. Ha, Y. K. Park, H. Lee, D. J. Suh and J. Jae, Appl. Catal., B, 2019, 241, 588–597 CrossRef CAS.
- F. Li, L. J. France, Z. Cai, Y. W. Li, S. J. Liu, H. M. Liu, J. X. Long and X. H. LI, Appl. Catal., B, 2017, 214, 67–77 CrossRef CAS.
- G. Z. Xu, C. Liu, A. Y. Hu, Y. M. Xia, H. J. Wang and X. Liu, Mol. Catal., 2019, 475, 110384 CrossRef CAS.
- A. K. Mohanty, S. Vivekanandhan, J. M. Pin and M. Misra, Science, 2018, 362, 536–542 CrossRef CAS.
- X. Zhang, M. Jiang, N. Niu, Z. Chen, S. Li, S. Liu and J. Li, ChemSusChem, 2018, 11, 11–24 CrossRef.
- T. P. J. Knowles and R. Mezzenga, Adv. Mater., 2016, 28, 6546–6561 CrossRef CAS.
- M. A. Rahim, S. L. Kristufek, S. Pan, J. J. Richardson and F. Caruso, Angew. Chem., Int. Ed., 2019, 58, 1904–1927 CrossRef CAS.
- S. Zhou, F. Dai, Y. Chen, C. Dang and H. Qi, Green Chem., 2019, 21, 1421–1431 RSC.
- H. Li, X. F. Liu, T. T. Yang, W. F. Zhao, S. Saravanamurugan and S. Yang, ChemSusChem, 2017, 10, 1761–1770 CrossRef CAS.
- X. Wang, W. Y. Gao, Z. Niu, L. Wojtas, J. A. Perman, Y. S. Chen, B. Aguila and S. Q. Ma, Chem. Commun., 2018, 54, 1170–1173 RSC.
- J. Song, B. Zhou, H. Zhou, L. Wu, Q. Meng, Z. Liu and B. Han, Angew. Chem., Int. Ed., 2015, 54, 9399–9403 CrossRef CAS.
- H. Li, T. Yang and Z. Fang, Appl. Catal., B, 2018, 227, 79–89 CrossRef CAS.
- H. Li, J. He, A. Riisager, S. Saravanamurugan, B. Song and S. Yang, ACS Catal., 2016, 6, 7722–7727 CrossRef CAS.
- S. Zhou, F. Dai, Z. Xiang, T. Song, D. Liu, F. Lu and H. Qi, Appl. Catal., B, 2019, 248, 31–43 CrossRef CAS.
- Y. F. Sha, Z. H. Xiao, H. C. Zhou, K. L. Yang, Y. M. Song, N. LI, R. X. He, K. D. Zhi and Q. S. Liu, Green Chem., 2017, 19, 4829–4837 RSC.
- L. Hu, T. Li, J. X. Xu, A. Y. He, X. Tang, X. Z. Chu and J. M. Xu, Chem. Eng. J., 2018, 352, 110–119 CrossRef CAS.
- C. Xie, J. L. Song, B. W. Zhou, J. Y. Hu, Z. R. Zhang, P. Zhang, Z. W. Jiang and B. X. Han, ACS Sustainable Chem. Eng. J., 2016, 4, 6231–6236 CrossRef CAS.
- H. Li, Z. Fang, J. He and S. Yang, ChemSusChem, 2017, 10, 681–686 CrossRef CAS.
- J. L. Hao, L. M. Han, Y. F. Sha, X. X. Yu, H. Y. Liu, X. Y. Ma, Y. Z. Yang, H. C. Zhou and Q. S. Liu, Fuel, 2019, 239, 1304–1314 CrossRef CAS.
- A. Soto-Vaca, A. Gutierrez, J. N. Losso, Z. M. Xu and J. W. Finley, J. Agric. Food Chem., 2012, 60, 6658–6677 CrossRef CAS PubMed.
- A. M. Bacelo, S. C. R. Santos and C. M. S. Botelho, Chem. Eng. J., 2016, 303, 575–587 CrossRef.
- Q. Dai, Q. Yu, Y. Tian, X. L. Xie, A. X. Song, F. Caruso, J. C. Hao and J. W. Cui, ACS Appl. Mater. Interfaces, 2019, 11, 29305–29311 CrossRef CAS PubMed.
- P. Chowdhury, P. K. B. Nagesh, E. Hatami, S. Wagh, N. Dan, M. K. Tripathi, S. Khan, B. B. Meibohm, S. C. Chauhan, M. Jaggi and M. M. Yallapu, J. Colloid Interface Sci., 2019, 535, 133–148 CrossRef CAS.
- B. J. Kim, S. Han, K. B. Lee and I. S. Choi, Adv. Mater., 2017, 29, 1700784 CrossRef.
- J. Wei, Y. Liang, Y. X. Hu, B. Kong, J. Zhang, Q. F. Gu, Y. P. Tong, X. B. Wang, S. P. Jiang and H. T. Wang, Angew. Chem., Int. Ed., 2016, 55, 12470–12474 CrossRef CAS.
- T. Zeng, X. L. Zhang, Y. Y. Guo, H. Y. Niu and Y. Q. Cai, J. Mater. Chem. A, 2014, 2, 14807–14811 RSC.
- L. Z. Zhou, P. H. Shao, K. Zhang, L. M. Yang, D. You, H. Shi, S. G. Pavlostathis, W. Q. Lai, D. L. Liang and X. B. Luo, Chem. Eng. J., 2019, 364, 160–166 CrossRef.
- N. A. S. Ramli and N. A. S. Amin, Appl. Catal., B, 2015, 5, 487–498 CrossRef.
- B. B. Li, Z. F. Ju, M. Zhou and D. Q. Yuan, Angew. Chem., Int. Ed., 2019, 58, 7687–7691 CrossRef CAS.
- J. Y. Lee, O. K. Farha, J. Roberts, K. A. Scheidt, S. B. T. Nguyen and J. T. Hupp, Chem. Soc. Rev., 2009, 38, 1450–1459 RSC.
- M. Yoon, R. Srirambalaji and K. Kim, Chem. Rev., 2011, 112, 1196–1231 CrossRef.
- M. Kim, J. F. Cahill, Y. X. Su, K. A. Prather and S. M. Cohen, Chem. Sci., 2012, 3, 126–130 RSC.
- H. Li, Y. Li, Z. Fang and R. L. Smith, Jr., Catal. Today, 2019, 319, 84–92 CrossRef CAS.
- T. Komanoya, K. Nakajima, M. Kitano and M. Hara, J. Phys. Chem. C, 2015, 119, 26540–26546 CrossRef CAS.
- Y. Román-Leshkov and M. E. Davis, ACS Catal., 2011, 1, 1566–1580 CrossRef.
- J. L. Song, L. Q. Wu, B. W. Zhou, H. C. Zhou, H. L. Fan, Y. Y. Yang, Q. L. Mei and B. X. Han, Green Chem., 2015, 17, 1626–1632 RSC.
- H. Y. Luo, D. F. Consoli, W. R. Gunther and Y. Román-Leshkov, J. Catal., 2014, 320, 198–207 CrossRef CAS.
Footnote |
† Electronic supplementary information (ESI) available. See DOI: 10.1039/c9gc03393a |
|
This journal is © The Royal Society of Chemistry 2020 |
Click here to see how this site uses Cookies. View our privacy policy here.