DOI:
10.1039/C9RA09769D
(Review Article)
RSC Adv., 2020,
10, 7384-7395
Application of MoS2 in the cathode of lithium sulfur batteries
Received
22nd November 2019
, Accepted 20th January 2020
First published on
18th February 2020
Abstract
Molybdenum disulfide (MoS2) with a two-dimensional layered structure can effectively inhibit the shuttle effect of lithium–sulfur batteries (Li–S batteries). It contains metal–sulfur bonds and combines with polysulfides through electrostatic bonds or chemical bonds. In this paper, the structure and properties of MoS2 are briefly introduced, and the research progress on the design, preparation, structure and properties of MoS2 as a cathode material for Li–S batteries in recent years is reviewed. The effects of MoS2 structure and its composition with carbon materials or metallic oxides on the performance of the electrode materials are analyzed. Finally, the existing problems and possible future research directions are pointed out.
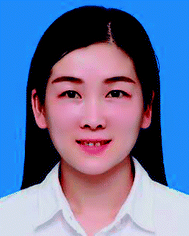 Yanyun Liu | Liu Yanyun graduated from the School of Materials Science and Engineering, Shaanxi University of Science and Technology in 2015. She is currently a student in the School of Metallurgical Engineering of Xi'an University of Architecture and Technology for her Master's degree. She is mainly focusing on the research of lithium sulfur batteries. |
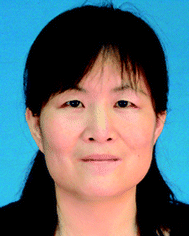 Chunjuan Cui | Cui Chunjuan obtained her M.E. degree and PhD degree in Materials Processing Engineering from Northwestern Polytechnical University and is now serving in the School of Metallurgical Engineering, Xi'an University of Architecture and Technology. She is currently a professor and doctoral supervisor. She performed collaborative research in 2013–2014 at the University of Kentucky, USA. She has published more than 50 journal papers, and more than 30 of them were indexed by SCI and EI. She has also published an academic monograph on metal solidification theory and application technology. Her team's research interests are theory and technology of directionally solidified metal matrix composites, and lithium sulfur batteries. |
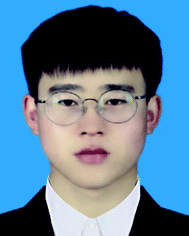 Yue Liu | Liu Yue graduated from the School of Metallurgical Engineering, Xi'an University of Architecture and Technology in 2019, majoring in metal material engineering. He is currently a student in the School of Metallurgical Engineering of Xi'an University of Architecture and Technology for his Master's degree. His main direction is lithium sulfur battery research. |
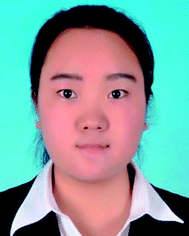 Wei Liu | Liu Wei graduated from the School of Material Engineering of Xi'an Aeronautical University in 2018. She is currently a graduate student in the School of Metallurgical Engineering, Xi'an University of Architecture and Technology. Now, she is mainly focusing on the research of high temperature oxidation and corrosion of the directionally solidified Ni–Si eutectic composites. |
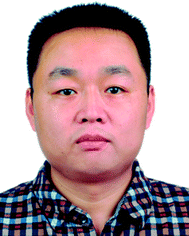 Jian Wei | Wei Jian obtained his M.E. degree and PhD degree in Materials Processing Engineering from Northwestern Polytechnical University and is now serving in the School of Metallurgical Engineering, Xi'an University of Architecture and Technology. He is currently a professor and doctoral supervisor. As a professor in the School of Materials and Mineral Resources of Xi'an University of Architecture and Technology, he is mainly engaged in the research of one-dimensional nanomaterials, lithium-ion batteries and fiber composite materials. He has published two university planning textbooks and more than 50 academic papers. He is also the Director of the Youth Working Committee of the China Society of Materials Research. |
1. Introduction
With the development of social economy and the continuous increase of the population in the world, the total amount of energy consumed by humans and society is increasing. Traditional energy production and consumption has caused global warming and ecological environmental destruction. At the same time, according to current energy consumption patterns, global fossil energy will be exhausted in the future. Therefore, energy storage and transformation is the key to coping with the climate crisis and achieving sustainable development of human society.1
Li–S batteries are rechargeable and generally consist of a sulfur-based cathode, binder, separator, organic liquid electrolyte, lithium anode and current collector. A schematic diagram of a Li–S battery is shown in Fig. 1.2
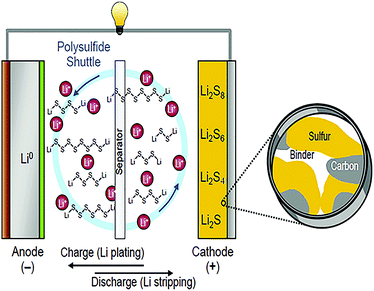 |
| Fig. 1 Schematic diagram of a Li–S battery.2 | |
The working principle of Li–S batteries is shown in Fig. 2.3 During the discharging process, sulfur in the solid phase (S8(s)) is firstly dissolved in the electrolyte and then transforms to S8(l). Then S8(l) is gradually reduced to high-order lithium polysulfides (Li2Sn, 4 ≤ n ≤ 8), which are easily soluble in the electrolyte and diffuse from the cathode to the electrolyte. As discharge continues, the high order lithium polysulfides are further reduced to low order lithium polysulfides (Li2S2 and Li2S) which are less soluble in the electrolyte. During the charging process, the discharge products (Li2S2 and Li2S) are gradually oxidized to high order lithium polysulfides and eventually oxidized to elemental sulfur.
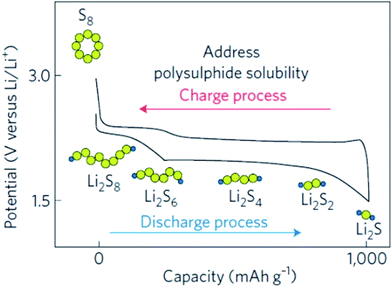 |
| Fig. 2 Voltage profiles of a Li–S battery.3 | |
Metallic lithium has very high electro-negativity while possessing the lowest density among all metals, leading to its highest specific capacity (3861 mA h g−1).4 Element sulfur has a theoretical specific capacity of 1675 mA h g−1.5 Thus, Li–S batteries can reach high theoretical gravimetric and volumetric energy densities of 2500 W h kg−1 and 2800 W h L−1, respectively. Given a complete reaction to Li2S, the energy densities are 3–5 times as high as those of commercial lithium-ion batteries.6,7 Moreover, sulfur also has the advantages of low cost, non-toxicity and high storage capacity as compared with that of conventional lithium-ion batteries.8 Furthermore, Li–S batteries have more possibility of commercialization than lithium-air batteries. Therefore, Li–S batteries have good application prospects in energy storage to reduce the use of fossil fuels and protect the environment.
2. Research situation of Li–S batteries
The development of Li–S batteries was initiated in 1962. Herbert9 was the first person to have the idea to use sulfur as the cathode in Li–S batteries. General Motors also proposed to use sulfur as an active material for thermal batteries and put the batteries into early electric vehicle projects.10 In 1976, Whittingham et al.11 successfully researched Li–TiS2 secondary batteries using layered TiS2 as the cathode and metal lithium as the anode. However, it failed to be commercialized due to safety problems such as “lithium dendrites”. In 2009, Nazar12 with his team in Canada stimulated attention toward Li–S batteries when they successfully compounded high performance cathode materials of Li–S batteries with ordered mesoporous carbon CMK-3 and sulfur. At present, the representative manufacturers of Li–S batteries in the world are Sion Power, Poly plus and Moltech in the US, Oxis in the UK and Samsung in Korea, among which the US is expected to reach 500 W h kg−1 as the energy density of Li–S batteries in the coming years.
Although Li–S batteries have many advantages, there are many factors that have gravely hindered their commercialization, for example, the insulating nature of sulfur and the lower electronic and ionic conductivity of the discharging products result in poor electrode reaction kinetics,13 and polysulfides formed in the cathode can be easily dissolved in the electrolyte and aggregate into the anode through the diaphragm during the charging and discharging processes. The redundant reaction is called the “shuttle effect” of polysulfides and results in capacity loss and cycling decay.14–16 The volume expansion of sulfur during charging and discharging can be up to 80%. This causes the powdering and collapse of the electrode material and worsens the electrical contact between the electrode material and the collector. The decline of the lithium storage capacity will be accelerated to some extent as a result.17
There are two main research points in order to promote the commercialization of Li–S batteries. The first one is improving the conductivity of the cathode through compounding with high conductivity materials during the preparation of the initial electrode materials. The second one is adding materials that have high adsorption for polysulfides to inhibit the dissolution of polysulfides. Carbon materials have been paid more attention in recent years due to their excellent electrical conductivity, non-toxicity, and effective inhibition of the shuttle effect. However, the physically weak bonding between carbon and polysulfides is not strong enough to completely inhibit the shuttle effect. Instead, the application of transition metal sulfides can effectively solve this problem. The metal–sulfur bonds can combine with polysulfides through electrostatic or chemical bonds,18 which can effectively inhibit the dissolution of polysulfides. The emergence of metal sulfides, such as MoS2, has attracted researchers' attention.
3. Research situation of MoS2 as a cathode material of Li–S batteries
MoS2 is a kind of typical graphene-like two-dimensional layered transition metal sulfide. It has a high theoretical storage capacity of 670 mA h g−1 when it reacts with Li+ according to the conversion reaction (MoS2 + 4Li+ + 4e− → Mo + 2Li2S), which is much higher than that of graphene (372 mA h g−1).19 Each layer is composed of S–Mo–S by covalent bonding in a hexagonal sandwich-like structure. In other words, each layer of Mo is sandwiched between two layers of S atoms, and the layers are connected by weak van der Waals forces.20 A schematic diagram of the structural unit is shown in Fig. 3.21
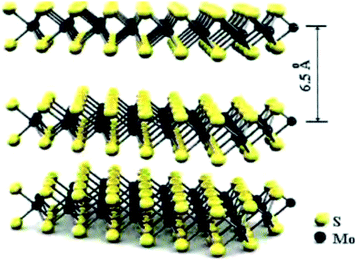 |
| Fig. 3 Structural unit diagram of MoS2.21 | |
This special structure makes the interaction forces inside the layer strong, while the interaction between layers is relatively weak, and this makes it easy for external substances to be embedded.22 At the same time, double-layer MoS2 also has good storage capacity of charge, and the Mo atoms have an oxidation state from 2 valence to 6 valence. High theoretical lithium storage capacity is produced by these characteristics.23 When used as the cathode material of Li–S batteries, the electrochemical reactions between MoS2 and Li+ are shown as follows:24–26
| MoS2 + xLi+ + xe− → LixMoS2, | (1) |
| LixMoS2 + (4 − x)Li+ + (4 − x)e− → Mo + 2Li2S, | (2) |
The strong adsorption of MoS2 can prevent polysulfide groups from dissolving in Li–S batteries. Thus, it can ensure lower volume expansion and better cycling stability over several thousands of charge/discharge cycles. Fig. 4 illustrates the polysulfide immobilization and conversion processes on the surface of nitrogen-doped carbon@MoS2 (NC@MoS2). It is found that MoS2 can accelerate the redox reaction kinetics through strong adsorption of polysulfides, fast electron transfer and catalysis for fast redox.
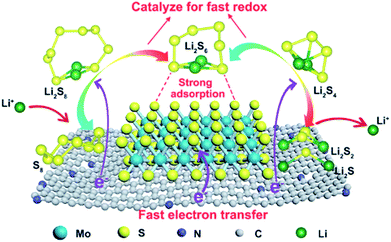 |
| Fig. 4 Polysulfide immobilization and conversion processes on the surface of NC@MoS2.27 | |
Wu27 proposed density functional theory (DFT) calculations to explore the catalytic effect of NC@MoS2 on polysulfide demobilization and conversion. Soluble long-chain polysulfide Li2S6 was chosen as the prototype for calculations. Fig. 5(a–c) display the optimized geometries of Li2S6 adsorption on carbon matrix, NC, and MoS2 (001) surfaces. The adsorption energies for NC and MoS2 are −0.66 and −0.57 eV respectively, which are larger than that of a pure carbon matrix. This indicates a stronger polysulfide adsorptivity. Therefore, MoS2 possesses superior anchoring ability for polysulfides.
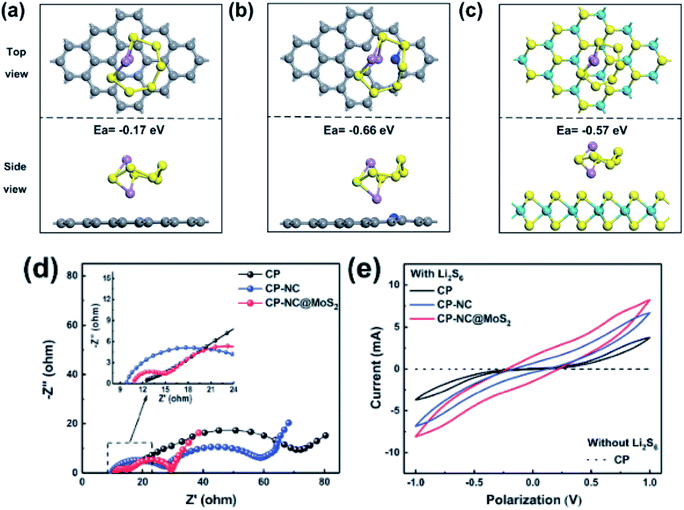 |
| Fig. 5 Optimized geometries of Li2S6 on (a) carbon matrix, (b) NC, and (c) MoS2 (001) surfaces, in which grey, yellow, blue, purple, and green spheres represent C, N, S, Li, and Mo, respectively; Nyquist plots (d) and CV (e) curves of Li2S6 symmetric cells employing CP, CP-NC, and CP-NC@MoS2 as working electrodes.27 | |
Then, symmetric cells with a Li2S6 catholyte were assembled to study the transition kinetics of polysulfide conversion. As shown in Fig. 5(d), CP-NC@MoS2 electrodes exhibit a decreased charge-transfer resistance, implying enhanced reaction kinetics of polysulfide conversion. Fig. 5(e) shows the CV curves of Li2S6 symmetric cells recorded at 50 mV s−1 within a voltage window of −1.0 V to 1.0 V. The CP-NC@MoS2 electrode shows significantly increased redox currents, further confirming faster redox kinetics in the soluble region.
Remarkably, the effective polysulfide adsorption ability and high electronic conductivity of MoS2 enable fast redox of Li–S batteries. Therefore, MoS2 plays a dominant role in the redox reaction kinetics of polysulfide conversion.
Fig. 6 shows the energy profile of polysulfide conversion on a MoS2 (001) surface.28 It was confirmed that MoS2 participates in the polysulfide conversion and accelerates the kinetics of the polysulfide redox reactions in Li–S batteries. The polysulfide conversion on the MoS2 (001) surface undergoes a “lithiation-cleavage–lithiation-cleavage–lithiation-cleavage” mechanism from the reactant S8@MoS2 to the product Li2S@MoS2. The polysulfides need to coordinate with the MoS2 surface to accelerate electron transfer, and the system energy decreases significantly after reduction. The fast conversion of the soluble polysulfides decreases their accumulation in the sulfur cathode and inhibits their subsequent loss from the cathode. The suppression of this loss mechanism leads to a more sustained cyclability.29,30
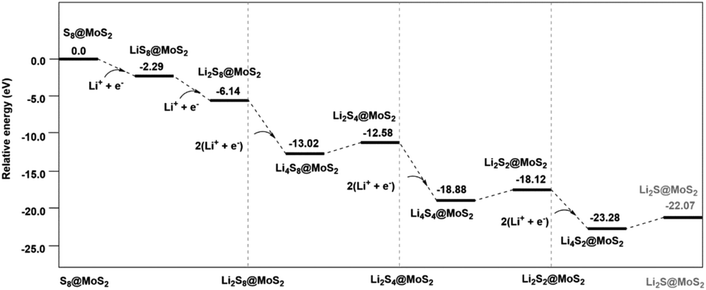 |
| Fig. 6 Energy profile of the polysulfide conversion mechanism on the MoS2 (001) surface.28 | |
3.1 Effect of MoS2 structure on electrical properties
A change in the MoS2 structure has a great influence on the capacity and cycling stability of electrode materials.31 Therefore, optimizing the structure of MoS2 nanomaterials can effectively improve the electrochemical performance of Li–S batteries. There are three main types: one-dimensional MoS2 like nanotubes, two-dimensional MoS2 like nanosheets, and three-dimensional MoS2 like nanoparticles, nanoflowers and other special nano-structured cathode materials.
3.1.1 One-dimensional MoS2 nanomaterials.
Qiu32 synthesized tubular MoS2/S composites with a template method. The tubular structure has double the surface area and is favorable for the fixation and infiltration of sulfur into the MoS2 nanotubes as shown in Fig. 7. The initial capacity of the tubular MoS2/S composites can reach 650 mA h g−1 at 100 mA g−1. However, the reversible specific capacity decreases to 450 mA h g−1 after 50 cycles. The electrochemical properties are not good. MoS2 and sulfur can be compounded to some extent, but sulfur cannot well penetrate into the tube to form a MoS2/S composite, and only mixing of the two phases can occur.
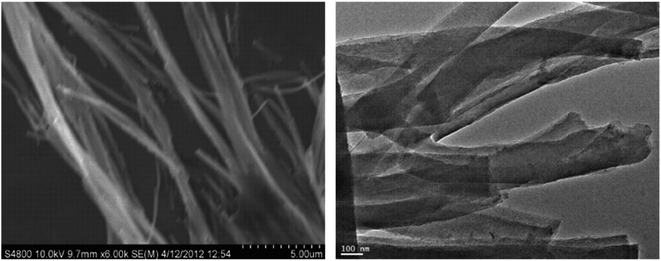 |
| Fig. 7 TEM image of MoS2 tubes.32 | |
However, Qiu did not point out the mechanism of inhibiting the shuttle effect of MoS2 nanotubes in Li–S batteries. There are few reports on the application of one-dimensional MoS2 nanomaterials in Li–S batteries at present, and further study in this area is required. If MoS2 nanotubes with a mesoporous structure can be synthesized, the loading rate of sulfur and the cyclic properties of the electrode materials will be greatly improved. This structure contributes to the inflow of sulfur through the micropores of the MoS2 nanotubes at 155 °C (at 155 °C, the S8 is mainly short linear sulfur chains, and the viscosity of molten sulfur is the lowest33). On the one hand, MoS2 nanotubes have double the surface area, so the loading of sulfur is increased, and more reactive sites are provided for the electrochemical reaction. On the other hand, in the process of electrochemical reaction, molybdenum polysulfides can be controlled or adsorbed inside the MoS2 nanotubes, which can inhibit the shuttle effect of Li–S batteries.
3.1.2 Two-dimensional MoS2 nanomaterials.
Two-dimensional nanomaterials have been extensively studied due to their special structures and excellent physical properties, such as high specific surface area. Two-dimensional structures can form networks through overlapping and stacking. They also have a large surface area which can provide more reactive sites and an open pore structure. These properties are very helpful for enabling effective anchoring of polysulfides and enhancing the reaction kinetics.34
Zhao35 proposed a MoS2/mesoporous carbon hollow sphere (MCHS) structure as an efficient sulfur cathode in order to deal with the shuttle effect, avoid active material loss and prevent performance decay of Li–S batteries. Ultrathin multi-layer MoS2 nanosheets are uniformly distributed in the mesoporous carbon hollow spheres, enhancing the physical adsorption and chemical entrapment functionalities towards the soluble polysulfides. Polar MoS2 can trap polysulfides via strong chemical bonds, so the polysulfides are largely fixed and remain inside the conductive MCHS as shown in Fig. 8. Benefiting from these structural advantages, the sulfur-impregnated MoS2/MCHS cathode presents remarkable electrochemical performance.
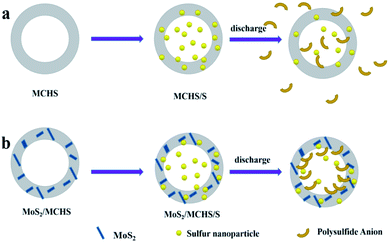 |
| Fig. 8 Proposed adsorption mechanism of polysulfides for the (a) MCHS/S and (b) MoS2/MCHS/S electrodes.35 | |
Zhao36 studied MoS2 nanosheets by liquid phase exfoliation. As shown in Fig. 9, the layered MoS2 nanosheets can achieve a good coordination with graphene and control polysulfides on graphene. Yu37 deposited an ultrathin layer of MoS2 (10–40 nm) nanoflakes by a liquid-based self-assembly method in the cathode of Li–S batteries. The results show that the reversible specific capacity reaches 1010 mA h g−1 at 0.5C, and the capacity fading is 0.11% per cycle over 400 cycles. The MoS2 interlayer inhibits polysulfide diffusion across the separator and remarkably improves the battery performance. Moreover, the MoS2 films with a high density of catalytic active edges can achieve high areal sulfur loading.
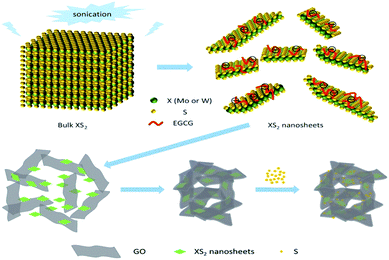 |
| Fig. 9 Proposed adsorption mechanism of polysulfides for XS2 (Mo or W).36 | |
There are still many researchers studying the application of two-dimensional MoS2 in Li–S batteries.38–41 Many properties of two-dimensional MoS2 are highly sought after for application in batteries. Two-dimensional MoS2 has a large surface area, while maintaining a low size, especially thin-layer MoS2, which can provide more active sites, and the electrode materials and electrolyte can fully contact each other. This is helpful to realize fast electrochemical reaction kinetics. In addition, the thin layer of MoS2 can achieve a good match with graphene, improving the capacity of the electrode materials.42 At the same time, it can effectively inhibit the shuttling of polysulfides. At present, the preparation methods of thin layer MoS2 mainly include the stripping method,43 high temperature solid phase method,44,45 template method46 and hydrothermal method.47 The MoS2 layers are connected with each other by weak van der Waals forces, and delamination can be realized in theory. However, the prepared MoS2 in most actual exploration processes is a small amount of thin layer only in the liquid state. Therefore, it is difficult to realize a large number of thin layer products of MoS2, which has become the main difficulty in their exploration.
3.1.3 Three-dimensional MoS2 nanomaterials.
For three-dimensional nanomaterials, electrons can move freely in all three dimensions. Different morphologies, such as nanoparticles and nanoclusters, have diverse properties.
At present, the design of nano-core-shell structures has become a research hotspot. This is because the core structure can provide supportive materials for the synthesis of MoS2 shell structures, which can fix polysulfides inside the shell, effectively inhibiting the dissolution of polysulfides, and improving the material's reversible cycling performance. Cheng48 synthesized a core–shell structured MoS2@S cathode material through a chemical route using MnCO3 as a template, as shown in Fig. 10. A picture of the core–shell structured MoS2@S is shown in Fig. 11. The initial discharge capacity of the material is 800.6 mA h g−1 at 1C rate, and the capacity retention rate is 79% after 200 cycles. For comparison, a MoS2/S cathode was also synthesized. It is found that the electrochemical performance of the core–shell structures MoS2@S cathode is much improved as compared with the MoS2/S composite. The high capacity and cycling stability of MoS2@S cathode materials are attributed to the mechanical inhibition and chemical combination of the polar MoS2 shell with lithium polysulfides, which further illustrates that the core–shell structure of MoS2@S can effectively inhibit the shuttling of polysulfides.
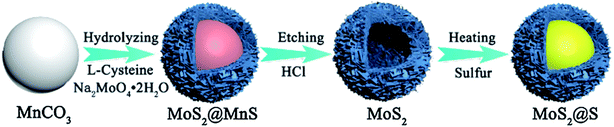 |
| Fig. 10 Schematic of the synthesis route of the core–shell structured MoS2@S spherical cathode.48 | |
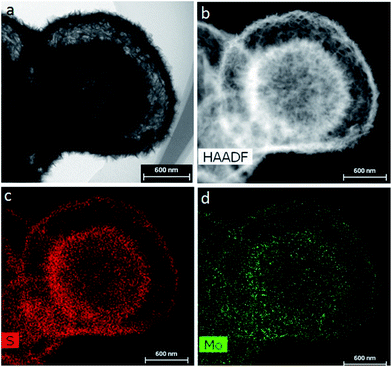 |
| Fig. 11 (a) TEM image, (b) HAADF image, and (c) S and (d) Mo element mapping of the MoS2 shells.48 | |
Other researchers have also49–53 devoted their attention to the application of core–shell structured MoS2 nanocomposites. The double-layer core–shell structure has good prospects. This special structure can effectively alleviate the shuttle effect of batteries. On the other hand, it can improve the sulfur loading rate and buffer the volume expansion of sulfur during the charging and discharging processes. However, the sulfur load is hard to improve, because the infiltration of sulfur is often not sufficient and uniform. And in the process of experiments, superior formation conditions of the core–shell structure need to be explored when the repeatability is not high.
Flower-like nanomaterials have a large surface area because of their unique and complex morphology. Therefore, the application of flower-like nanomaterials in the cathode of Li–S batteries can effectively improve the loading rate of sulfur and the capacity of the batteries. Tang54 synthesized MoS2 nanoflowers consisting of many irregular MoS2 nanosheets with an average diameter of about 1–2 µm by a facile CTAB-assisted hydrothermal method at 180 °C for 24 h. Zhang55 successfully synthesized high-purity MoS2 nanoflowers by a hydrothermal method. The particle diameter is around 300 nm, consisting of tens of hundreds of MoS2 petals, and the thickness of each layered petal is about 10 nm. Through gradient experiments, it was found that silicotungstic acid played an important role in the formation of the nano-flower MoS2. Xiong56 fabricated hierarchical porous MoS2/C flower-like microspheres as shown in Fig. 12. The initial discharge capacity of the porous MoS2/C flower-like microsphere electrode was 1125.9 mA h g−1. The discharge capacity remained at 916.6 mA h g−1 after 400 cycles at 200 mA g−1. This is attributed to the unique porous composite structure of MoS2/C effectively improving the contact area between the active substances and electrolytes. This also provides more channels for the rapid transmission of electrons and ions during charging and discharging, contributing to a fast kinetic reaction and effectively improving the electrochemical performance of the batteries.
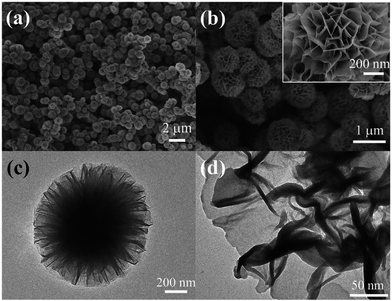 |
| Fig. 12 Morphological and structural characterization of the MoS2/C flower-like microspheres: (a and b) FESEM images with low and high magnification, respectively; (c and d) TEM images with low and high magnification, respectively.56 | |
Flower-like nanomaterials have been studied a lot among all kinds of battery materials, because the flower structure has a large specific surface area, which can effectively improve the loading of active materials. Compared with the core–shell structure, the synthesis process is relatively mature, and has been widely studied.57,58
Therefore, different structures of MoS2 have great impacts on the electrochemical properties of materials. In the current research, three-dimensional MoS2 is widely researched as the cathode material of Li–S batteries, while the research of two-dimensional MoS2 is mostly conducted in the diaphragm. Few research studies have paid attention to the application of one-dimensional MoS2 in Li–S batteries, which is worth exploring. Nano-mesoporous materials have the advantages of high specific surface area, well-ordered channels, high porosity and good thermal stability. As a battery material, they can retain high porosity and uniform aperture distribution on adjusting the size of the material. So the design of mesoporous MoS2 nanomaterials can improve the reversibility, rate performance of discharge and cycling life of electrode materials.59–62
3.2 Preparation and properties of MoS2/carbon composite cathode materials
In spite of many advantages, MoS2 has another serious disadvantage: poor conductivity which cannot guarantee rapid electron transfer in the process of electrode reaction. Therefore, simple structure design of MoS2 is not enough for high-rate performance. At present, conductive nanoporous MoS2 cathode materials with excellent electrochemical properties have been put forward, such as MoS2/carbon nanocomposites.63,64 Carbon materials that have an interconnected conductive network structure can effectively improve the conductivity and dynamic performance of electrodes.65 It has been found that the strong chemical and electronic coupling between MoS2 nanoparticles and graphene nanosheets could allow rapid electron transportation through the conductive graphene substrate.66,67
At present, the synthesis methods of MoS2/carbon composites in Li–S batteries are mainly based on chemical methods. They include vapor phase synthesis, like chemical vapor deposition, and liquid phase methods, such as hydrothermal methods, solvothermal methods, template methods, thermal decomposition and precipitation methods, etc.
3.2.1 Chemical vapor deposition.
Chemical vapor deposition (CVD) is a direct synthesis method to obtain a uniform MoS2 coating on targeted carbon substrates. Namjo et al.68 obtained a uniform MoS2 coating on the surface of porous carbon structures with nano-sized pores by chemical vapor deposition. Mugyeom et al.69 synthesized MoS2–SRGO composites by one-pot deposition of MoO2 on SRGO and simultaneous reduction of GO to SRGO in supercritical methanol followed by sulfurization. The obtained MoS2–SRGO composites contain an 11–14 layer crystalline MoS2 phase.
However, the deposition temperature of CVD is relatively high, generally at 900–1200 °C, which is not conducive to the preparation of inorganic materials. In recent years, with the improvement of industrial production requirements, the process and equipment of CVD have been continuously improved. Not only are a variety of new heating sources used, but also auxiliary methods such as plasma, laser and electron beam are used to reduce the reaction temperature, making its application scope wider.70,71
3.2.2 Hydrothermal methods.
Hydrothermal methods are a common method for material preparation. Ren72 prepared multi-dimensional N-doped porous carbon/MoS2/CNT nano-structures by a facile hydrothermal process. The synergistic action of polar MoS2 and porous carbon imbedded with electro-conductive acid–CNTs could construct a conductive network for the rapid transfer of ions/electrons. As the cathode of Li–S batteries, N-doped porous carbon/MoS2/CNT nanostructures deliver a high discharge capacity of 1313.4 mA h g−1 at 0.1C and good cycling stability with an average capacity fade of 0.059% per cycle after 500 cycles at 1.0C. This can significantly strengthen the chemical attraction with polysulfides. Chen73 prepared graphene-like-MoS2/graphene (GL-MoS2/G) composites by a hydrothermal method assisted with cetylammonium bromide cationic surfactant and subsequent heat treatment. You74 prepared a three-dimensional porous MoS2/rGO foam based sulfur cathode by a facile hydrothermal method. Few-layer MoS2 nanosheets with mixed 1T and 2H phases were developed on reduced graphene oxide, forming a continuous 3D porous network.
Solvothermal reactions are a development of hydrothermal reactions, where the reaction occurs in an organic solvent or non-aqueous solvent (such as organic amine, alcohol, ammonia, carbon tetrachloride or benzene, etc.).
Huo75 prepared S/N co-doped reduced graphene oxide modified with MoS2 by a one-pot solvothermal method with L-cysteine mixed in ethylene glycol. The specific surface area of this compound gel was estimated to be 151.41 m2 g−1. Its large specific surface area can promote the contact between the electrode and electrolyte, and the porous structure provides transport channels for electrons and ions. Electrochemical tests revealed that the capacity retention of the electrode was 91.32% after 500 cycles. The size and morphology of the phase particles can also be controlled by a solvothermal method, and the product also has good dispersion.
Hydrothermal methods can directly obtain powders with high purity, good dispersity and good crystal shape, with no need for burning treatment at high temperature, and the preparation process is relatively simple and the production cost is relatively low.76 However, solvothermal reactions are not as safe as hydrothermal reactions according to practical experience. So it would be better to avoid reactions that produce large quantities of gases and heat in solvothermal reactions.
3.2.3 Template methods.
Template methods are a very important technique to synthesize nanocomposites. Jiang77 synthesized nitrogen-doped hollow mesoporous carbon spheres (NMCS) using SiO2 as a template. Wang78 synthesized TiO2 nanotubes using MnO2 nanowires as templates and coated them with nitrogen-doped carbon (NC). Ultra-thin MoS2 nanosheets were grown on them by a hydrothermal method to obtain layered nanostructures of TiO2@NC@MoS2. Yin50 firstly prepared MoS2 and carbon on the surface of SiO2 nanoparticles as a sacrificial template by a sol–gel method with CTAB. Then, MoS2/carbon hollow microspheres wrapped with petaloid MoS2 lamellae and amorphous carbon were prepared by alkali liquor etching of the silica microsphere matrix. The average diameter of the MoS2/C hollow microspheres was approximately 200–300 nm. As the cathode material of a lithium battery, the first charge–discharge capacity was more than 1400 mA h g−1 at a current density of 100 mA g−1. This technique has the following advantages: simple experimental device, high flexibility, accurate control (size, morphology and structure), and effective prevention of agglomeration. Therefore, it has attracted wide attention.79
3.2.4 Thermal decomposition methods.
Li80 successfully studied MoS2-decorated coaxial nanocable carbon aerogel composites (MoS2/CNCA) by phenolic reaction and treatment at high temperature in a sulfur atmosphere. The MoS2-decorated carbon aerogel (CA) with carbon nanotubes as fine wires presented a narrow distribution of pore size, an interconnected structure and high conductance. The discharge capacity was 1314 mA h g−1 at 0.2C with the capacity retention of 81% after 200 cycles. These improved results can be attributed to MoS2 as a network entangled structure. However, the reaction is usually endothermic as heat is required to break chemical bonds in the compound. If decomposition is sufficiently exothermic, it can easily lead to thermal runaway and possibly an explosion.
In summary, hydrothermal methods and template methods are widely applied at present in the synthesis of Li–S battery cathode materials. Hydrothermal methods can synthesize nanomaterials in different dimensions in one step, such as nanotube structures, two-dimensional nanosheet structures, three-dimensional nanoflower-like structures, and hierarchical porous structures. The template method is more conducive to synthesizing core–shell structures, and it provides a good idea for solving the shuttle effect of Li–S batteries. In addition to the above methods, physical methods such as stripping and vacuum condensation, and chemical methods such as precipitation are also commonly used to prepare nanomaterials. However, these methods are less used to prepare MoS2 cathode composites for Li–S batteries.
3.3 Preparation and properties of MoS2/metal oxide composite cathode materials
Metal oxides, which have chemical bonds or electrostatic adsorption with polysulfides, can effectively inhibit the shuttling of polysulfides and improve the charge–discharge performance of materials as cathode materials of Li–S batteries. Zhang81 synthesized MoS2/SnO2 nanocomposites by a two-step hydrothermal method, and SnO2 was uniformly loaded on the surface of the MoS2 nanosheets. After loading SnO2 nanoparticles, the diffusion of lithium polysulfides was greatly inhibited, and the conductivity of the material was also improved by embedding Mo atoms. Xu82 first prepared TiO2@PNT coaxial nano-materials by a sol–gel method. Porous TiO2 nanotubes were obtained by roasting the PNT template in air. Furthermore, MoS2 nanosheet@TiO2 nanotube structures were successfully prepared by a solvothermal method. Porous TiO2 nanotubes as supporting materials slow down the agglomeration of MoS2 and make it distribute more uniformly. This intelligent design has remarkable lithium storage performance.
The use of metal oxides in cathode materials of Li–S batteries is of great significance. However, excessive introduction of metal oxides will affect the overall energy density of the batteries because of the high density of metal oxide. Moreover, their conductivity cannot meet the needs of cathode materials. Therefore, there are few studies on the application of MoS2 and metal oxides in Li–S battery cathode materials, and most of the current studies are on lithium-ion battery electrode materials.
4. Conclusions
As the cathode material of Li–S batteries, MoS2 can accelerate the redox reaction kinetics during the discharge/charge process through strong adsorption of polysulfides, fast electron transfer and catalysis for fast redox. It has great potential advantages for Li–S batteries.
(1) Strong adsorption of polysulfide lithium: as the cathode material of Li–S batteries, MoS2 has a great advantage in reducing the “shuttle effect” that has gravely hindered their commercialization. MoS2 has strong adsorption for sulfur-containing groups, thus preventing polysulfides from dissolving. Thus, it can ensure better cycling stability over several thousands of charge/discharge cycles.
(2) Graphene-like two-dimensional layered structure: MoS2 is a kind of typical graphene-like two-dimensional layered transition metal sulfide. Each layer of Mo is sandwiched between two layers of S atoms, and the layers are connected by weak van der Waals forces. This special structure makes the interaction forces inside the layer strong, while the interactions between layers are relatively weak, and this makes it easy for external substances to be embedded, especially those having a good match with graphene.
(3) Good storage capacity: MoS2 has a high theoretical storage capacity of 670 mA h g−1 when it reacts with Li+, which is much higher than that of graphene (372 mA h g−1). The Mo atom has an oxidation state from 2 valence to 6 valence. These characteristics determine its high theoretical lithium storage capacity. It can effectively solve the insulating nature and lower electronic and ionic conductivity of sulfur.
Many methods have been developed for the synthesis of MoS2 nanocompounds, and hydrothermal methods and template methods are widely applied at present. Hydrothermal methods can synthesize nanomaterials in different dimensions in one step, such as nanotube structures, two-dimensional nanosheet structures, three-dimensional nanoflower-like structures, and hierarchical porous structures. Template methods are more conducive to synthesizing core–shell structures, and provide a good idea for solving the shuttle effect of Li–S batteries. The progress of these methods has made great contributions to the development of Li–S batteries.
Conflicts of interest
There are no conflicts to declare.
Acknowledgements
The authors would like to thank the National Natural Science Foundation of China (51201121), the Science and Technology Foundation for Selected Overseas Chinese Scholars of Shaanxi Province (2015), the Key Industry Innovation Chain (group) Project of Shaanxi Province (No. 2019ZDLGY 04-04), and the Research Project of Shaanxi Engineering Technology Research Center for Wear-resisting Materials (2016NMZX03) for financial support.
References
- Z. Liua, H. Haoa, X. Chenga and F. Zhaoa, Critical issues of energy efficient and new energy vehicles development in China, Energy Policy, 2018, 115, 92–97 CrossRef.
- Y. Diao, K. Xie, X. Hong and S. Xiong, Analysis of the sulfur cathode capacity fading mechanism and review of the latest development for Li-S battery, Acta Chim. Sin., 2013, 71(4), 508–518 CrossRef CAS.
- A. Manthiram, Y. Fu, S. Chung, C. Zu and Y. Su, Rechargeable lithium-sulfur batteries, Chem. Rev., 2014, 114(23), 11751–11787 CrossRef CAS PubMed.
- G. Hu, C. Xu, Z. Sun, S. Wang, H. Cheng and F. Li, 3D Graphene-foam-reduced-graphene-oxide hybrid nested hierarchical networks for high-performance Li-S batteries, Adv. Mater., 2016, 28(8), 1603–1609 CrossRef CAS PubMed.
- W. Hu, H. Zhang, M. Wang, H. Zhang and C. Qu, Tri-modal mesoporous carbon/sulfur nanocomposite for high performance Li-S battery, Electrochim. Acta, 2016, 190, 322–328 CrossRef CAS.
- A. Manthiram, S. Chung and C. Zu, Lithium–sulfur batteries: progress and prospects, Adv. Mater., 2015, 27(12), 1980–2006 CrossRef CAS PubMed.
- L. Chen and L. L. Shaw, Recent advances in lithium–sulfur batteries, J. Power Sources, 2014, 267, 770–783 CrossRef CAS.
- Z. Zheng, F. Gu and X. Zhao, Progress in lithium sulfur battery cathode materials, Chemistry, 2018, 81(2), 99–108 CAS.
-
H. Danuta and U. Juliusz, Electric dry cells and storage batteries, US Pat., 3043896, 1962 Search PubMed.
- S. M. Selis, J. P. Wondowski and R. F. Justus, A high-rate, high-energy thermal battery system, J. Electrochem. Soc., 1964, 111(1), 6–13 CrossRef CAS.
- M. S. Whittingham, Electrical energy storage and intercalation chemistry, Science, 1976, 192(4244), 1126–1127 CrossRef CAS PubMed.
- X. Ji, K. T. Lee and L. F. Nazar, A highly ordered nanostructured carbon–sulphur cathode for lithium–sulphur batteries, Nat. Mater., 2009, 8(6), 500–506 CrossRef CAS PubMed.
- A. Manthiram, Y. Fu and Y. Su, Challenges and prospects of lithium–sulfur batteries, Acc. Chem. Res., 2013, 46(5), 1125–1134 CrossRef CAS PubMed.
-
L. Yin, Research on sulfur-based cathode materials for rechargeable lithium batteries, Doctoral dissertation, Shanghai Jiao Tong University, 2012.
- Z. Chen, R. Fang, C. Liang, Y. Gan and W. Zhang, Recent progress in sulfur cathode for Li-S batteries, Mater. Rev., 2018, 32(9), 1401–1411 Search PubMed.
- H. Wang, Y. Yang, Y. Liang, J. T. Robinson, Y. Li and A. Jackson, Graphene-wrapped sulfur particles as a rechargeable lithium-sulfur-battery cathode material with high capacity and cycling stability, Nano Lett., 2011, 11(7), 2644–2647 CrossRef CAS PubMed.
- M. A. Lowe, J. Gao and H. D. Abruna, Mechanistic insights into operational lithium–sulfur batteries by in situ x-ray diffraction and absorption spectroscopy, RSC Adv., 2014, 4(35), 18347 RSC.
- Z. Xu, H. You, L. Zhang and Q. Yang, Recent development of polysulfide barriers for Li-S batteries, Carbon, 2017, 124, 722 CrossRef.
-
W. Zhao, Structure design and applications of graphene and transition metal dichalcogenide a first-principles study, Doctoral dissertation, Tsinghua University, 2015.
- T. Stephenson, L. Zhi, B. Olsen and D. Mitlin, Lithium ion battery applications of molybdenum disulfide (MoS2) nanocomposites, Energy Environ. Sci., 2013, 7(1), 209–231 RSC.
- H. Fei, Z. Hui, F. Hao, L. Zhen-Hua, R. Meng and L. I. Xue-Jun, Research progress of molybdenum disulfide nano materials used for chemical power source, Advances in New and Renewable Energy, 2015, 3(5), 375–383 Search PubMed.
- J. Liu, A. Fu, Y. Wang, P. Guo, H. Feng and H. Li, Spraying coagulation-assisted hydrothermal synthesis of MoS2/carbon/graphene composite microspheres for lithium-ion battery applications, ChemElectroChem, 2017, 4(8), 2027–2036 CrossRef CAS.
- P. T. Dirlam, J. Park, A. G. Simmonds, K. Domanik, C. B. Arrington and J. L. Schaefer, Elemental sulfur and molybdenum disulfide composites for Li–S batteries with long cycle life and high-rate capability, ACS Appl. Mater. Interfaces, 2016, 8(21), 13437–13448 CrossRef CAS PubMed.
- Y. Wei, Z. Kong, Y. Pan, Y. Cao, D. Long and J. Wang, Sulfur film sandwiched between few-layered MoS2 electrocatalysts and conductive reduced graphene oxide as robust cathode for advanced lithium-sulfur battery, J. Mater. Chem. A, 2018, 6(14), 5899–5909 RSC.
- J. Xiao, X. Wang, X. Yang, S. Xun, G. Liu and P. K. Koech, Electrochemically induced high capacity displacement reaction of PEO/MoS2/graphene nanocomposites with lithium, Adv. Funct. Mater., 2011, 21(15), 2840–2846 CrossRef CAS.
- X. Fang, X. Yu, S. Liao, Y. Shi, Y. Hu and Z. Wang, Lithium storage performance in ordered mesoporous MoS2 electrode material, Microporous Mesoporous Mater., 2012, 151, 418–423 CrossRef CAS.
- W. Yang, W. Yang, L. Dong, X. Gao, G. Wang and G. Shao, Enabling immobilization and conversion of polysulfides through nitrogen-doped carbon nanotubes/ultrathin MoS2 nanosheets core-shell architecture for lithium-sulfur batteries, J. Mater. Chem. A, 2019, 7, 13103–13112 RSC.
- Q. Zhang, X. Zhang, M. Li, J. Liu and Y. Wu, Sulfur-deficient MoS2−x promoted lithium polysulfides conversion in lithium-sulfur battery: a first-principles study, Appl. Surf. Sci., 2019, 487, 452–463 CrossRef CAS.
- H. Lin, L. Yang, X. Jiang, G. Li, T. Zhang and Q. Yao, Electrocatalysis of polysulfide conversion by sulfur-deficient MoS2 nanoflakes for lithium–sulfur batteries, Energy Environ. Sci., 2017, 10, 1476–1486 RSC.
- J. Kibsgaard, Z. Chen, B. N. Reinecke and T. F. Jaramillo, Engineering the surface structure of MoS2 to preferentially expose active edge sites for electrocatalysis, Nat. Mater., 2012, 11(11), 963–969 CrossRef CAS PubMed.
- Q. Xu, S. Tian, T. Li, Z. Wang and L. Li, Application of polypropylene separator coated with molybdenum disulfide in lithium sulfur batteries, J. Funct. Polym., 2018, 31(05), 478–485 CAS.
-
B. Qiu, Synthesis of transition metal sulfides (MoS2,CoS2) and investigation of its electrochemical performance, Doctoral dissertation, Beijing University of Technology, 2013.
-
F. Sun, Preparation and performance study of sulfur cathode materials for lithium sulfur batteries, Doctoral dissertation, East China University of Science and Technology, 2014.
- J. Zhang, J. Zhang, K. Liu, T. Yang, J. Tian, C. Wang, M. Chen and X. Wang, Abundant defects-induced interfaces enabling effective anchoring for polysulfides and enhanced kinetics in lean electrolyte lithium-sulfur batteries, ACS Appl. Mater. Interfaces, 2019, 11(50), 46767–46775 CrossRef CAS PubMed.
- Y. Zhao, Q. Zhuang, W. Li, H. Peng, G. Li and Z. Zhang, Encapsulation of few-layer MoS2 in the pores of mesoporous carbon hollow spheres for lithium-sulfur batteries, Nanomaterials, 2019, 9, 1247 CrossRef CAS PubMed.
- H. Zhao, H. Wu, J. Wu, J. Li, Y. Wang, Y. Zhang and H. Liu, Preparation of MoS2/WS2 nanosheets by liquid phase exfoliation with assistance of epigallocatechin gallate and study as an additive for high-performance lithium-sulfur batteries, J. Colloid Interface Sci., 2019, 552, 554–562 CrossRef CAS PubMed.
- X. Yu, G. Zhou and Y. Cui, Mitigation of shuttle effect in Li-S battery using a self-assembled ultrathin molybdenum disulfide interlayer, ACS Appl. Mater. Interfaces, 2019, 11(3), 3080–3086 CrossRef CAS PubMed.
- A. Y. S. Eng, J. L. Cheong and S. S. Lee, Controlled synthesis of transition metal disulfides (MoS2 and WS2) on carbon fibers: effects of phase and morphology toward lithium-sulfur battery performance, Applied Materials Today, 2019, 16, 529–537 CrossRef.
- H. Lin, S. Zhang, T. Zhang, H. Ye, Q. Yao, G. Zheng and J. Lee, Simultaneous cobalt and phosphorous doping of MoS2 for improved catalytic performance on polysulfide conversion in lithium–sulfur batteries, Adv. Energy Mater., 2019, 9(38), 1902096 CrossRef.
- S. Majumder., M. Shao, Y. Deng and G. Chen, Ultrathin sheets of MoS2/G-C3N4 composite as a hosting material of sulphur for lithium-sulphur batteries with long cycle life and high rate capability, J. Power Sources, 2019, 431, 93–104 CrossRef CAS.
- M. Liu, C. Zhang, J. Su, X. Chen, T. Ma, T. Huang and A. Yu, Propelling polysulfide conversion by defect-rich MoS2 nanosheets for high-performance lithium-sulfur batteries, ACS Appl. Mater. Interfaces, 2019, 11(23), 20788–20795 CrossRef CAS PubMed.
- H. Xue, J. Wang, S. Wang, M. Sohail, C. Feng, Q. Wu, H. Li, D. Shi and Y. Zhao, Core-shell MoS2@graphene composite microspheres as stable anode for li-ion batteries, New J. Chem., 2018, 42, 15340–15345 RSC.
- S. Ji, Z. Yang, C. Zhang, Z. Liu, W. W. Tjiu and I. Y. Phang, Exfoliated MoS2 nanosheets as efficient catalysts for electrochemical hydrogen evolution, Electrochim. Acta, 2013, 109(11), 269–275 CrossRef CAS.
- N. Elizondo-Villarreal, R. Velazquez-Castillo, D. H. Galvan, A. Camacho and M. Jose Yacaman., Structure and catalytic properties of molybdenum sulfide nanoplatelets, Appl. Catal., A, 2007, 328(1), 88–97 CrossRef CAS.
- K. Liu, W. Zhang, Y. H. Lee, Y. Lin, M. Chang and C. Su, Growth of large-area and highly crystalline MoS2 thin layers on insulating substrates, Nano Lett., 2012, 12(3), 1538–1544 CrossRef CAS PubMed.
- C. M. Zelenski, G. L. Hornyak and P. K. Dorhout, Synthesis and characterization of CdS particles within a nanoporous aluminum oxide template, Nanostruct. Mater., 1997, 9(1–8), 173–176 CrossRef CAS.
- M. Wang, G. Li, H. Xu, Y. Qian and J. Yang, Enhanced lithium storage performances of hierarchical hollow MoS2 nanoparticles assembled from nanosheets, ACS Appl. Mater. Interfaces, 2013, 5(3), 1003–1008 CrossRef CAS PubMed.
- S. Cheng, X. Xia, H. Liu and Y. Chen, Core-shell structured MoS2@S spherical cathode with improved electrochemical performance for lithium-sulfur batteries, J. Mater. Sci. Technol., 2018, 34(10), 202–208 Search PubMed.
- J. Wu, N. You, X. Li, H. Zeng, S. Li, Z. Xue, Y. Ye and X. Xie, SiO2@MoS2 core–shell nanocomposite layers with high lithium ion diffusion as a triple polysulfide shield for high performance lithium–sulfur batteries, J. Mater. Chem. A, 2019, 7, 7644–7653 RSC.
- N. Li, Z. Liu, Q. Gao, X. Li, R. Wang, X. Yan and Y. Li, In situ synthesis of concentric C@MoS2 core–shell nanospheres as anode for lithium ion battery, J. Mater. Sci., 2017, 52(22), 13183–13191 CrossRef CAS.
- F. Gong, L. Peng, H. Liu, Y. Zhang, D. Jia, S. Fang, F. Li and D. Li, 3D core-shell MoS2 superspheres composed of oriented nanosheets with quasi molecular superlattices: mimicked embryo formation and li-storage properties, J. Mater. Chem. A, 2018, 6, 18498–18507 RSC.
- N. Li, H. Zhao, Y. Zhang, Z. Liu, X. Gong and Y. Du, Core–shell structured CeO2@MoS2 nanocomposites for high performance symmetric supercapacitors, CrystEngComm, 2016, 18, 4158–4164 RSC.
- X. Cao, H. Li, J. He, L. Kang, R. Jiang, F. Shi, H. Xu, Z. Lei and Z. Liu, Preparation and formation process of α-MnS@MoS2 microcubes with hierarchical core/shell structure, J. Colloid Interface Sci., 2017, 507, 18–26 CrossRef CAS PubMed.
- G. Tang, J. Sun, C. Wei, K. Wu, X. Ji, S. Liu, H. Tang and C. Li, Synthesis and characterization of flowerlike MoS2 nanostructures through CTAB-assisted hydrothermal process, Mater. Lett., 2012, 86(11), 9–12 CrossRef CAS.
- Y. Zhang, L. Mei, H. Liu, M. Tao and Z. Huang, Hydrothermal synthesis and characterization of flowerlike molybdenum disulfide microspheres, Nanosci. Nanotechnol. Lett., 2017, 9(3), 281–285 CrossRef.
- Q. Xiong and Z. Ji, Controllable growth of MoS2/C flower-like microspheres with enhanced electrochemical performance for lithium ion batteries, J. Alloys Compd., 2016, 673, 215–219 CrossRef CAS.
- Z. A. Ghazi, X. He, A. M. Khattak, N. A. Khan and Z. Tang, MoS2/celgard separator as efficient polysulfide barrier for long-life lithium-sulfur batteries, Adv. Mater., 2017, 29(21), 1606817 CrossRef PubMed.
- P. Guo, D. Liu, Z. Liu, X. Shang, Q. Liu and D. He, Dual functional MoS2/graphene interlayer as an efficient polysulfide barrier for advanced lithium-sulfur batteries, Electrochim. Acta, 2017, 256, 28–36 CrossRef CAS.
- X. Wang, Z. Zhang, Y. Chen, Y. Qu, Y. Lai and J. Li, Morphology-controlled synthesis of MoS2 nanostructures with different lithium storage properties, J. Alloys Compd., 2014, 600(25), 84–90 CrossRef CAS.
- X. Fang, X. Guo, Y. Mao, C. Hua, L. Shen and Y. Hu, Mechanism of lithium storage in MoS2 and the feasibility of using Li2S/Mo nanocomposites as cathode materials for Lithium-sulfur batteries, Chem.–Asian J., 2012, 7(5), 1013–1017 CrossRef CAS PubMed.
- Y. Kim and J. B. Goodenough, Lithium insertion into transition-metal monosulfides: tuning the position of the metal 4s band, J. Phys. Chem. C, 2014, 112(38), 15060–15064 CrossRef.
- H. Wei, Y. Ding, H. Li, Q. Zhang, N. Hu, L. Wei and Z. Yang, MoS2 quantum dots decorated reduced graphene oxide as a sulfur host for advanced lithium-sulfur batteries, Electrochim. Acta, 2019, 327, 134994 CrossRef CAS.
-
K. Zhang, Preparation of MoS2/Mo2C composite material and application in photo-assisted electrocatalytic hydrogen evolution, Doctoral dissertation, Harbin Institute of Technology, 2016.
- Y. Li, H. Wang, L. Xie, Y. Liang, G. Hong and H. Dai, MoS2 nanoparticles grown on graphene: an advanced catalyst for the hydrogen evolution reaction, J. Am. Chem. Soc., 2011, 133(19), 7296–7299 CrossRef CAS PubMed.
-
Z. Li, Study on sulfur/carbon composite cathode materials for lithium-sulfur batteries, Doctoral dissertation, Harbin Institute of Technology, 2014.
- W. Ahn, K. B. Kim, K. N. Jung, K. H. Shin and C. S. Jin, Synthesis and electrochemical properties of a sulfur-multi walled carbon nanotubes composite as a cathode material for lithium sulfur batteries, J. Power Sources, 2012, 202, 394–399 CrossRef CAS.
- S. Zhang, M. Zheng, J. Cao and H. Pang, Porous carbon/sulfur composite cathode materials for lithium-sulfur batteries, Prog. Chem., 2016, 28(8), 1148–1155 Search PubMed.
- J. Namjo, K. Han-Ki, K. Won-Sik, Y. C. Ji, H. Ji-Hyung and N. Joo-Youn, Uniform coating of molybdenum disulfide over porous carbon substrates and its electrochemical application, Chem. Eng. J., 2018, 356, 292–302 Search PubMed.
- M. Choi, S. K. Koppala, D. Yoon, J. Hwang, S. M. Kim and J. Kim, A route to synthesis molybdenum disulfide-reduced graphene oxide (MoS2-RGO) composites using supercritical methanol and their enhanced electrochemical performance for Li-ion batteries, J. Power Sources, 2016, 309, 202–211 CrossRef CAS.
- S. Luo, X. Qi, L. Ren, G. Hao, Y. Fan and Y. Liu, Photoresponse properties of large-area MoS2 atomic layer synthesized by vapor phase deposition, J. Appl. Phys., 2014, 116(16), 149 CrossRef.
- N. Perea-Lopez, Z. Lin, N. R. Pradhan, A. Iniguez-Rabago, A. Laura Elias and A. Mccreary, CVD-grown monolayered MoS2 as an effective photosensor operating at low-voltage, 2D Materials, 2014, 1(1), 011004 CrossRef.
- J. Ren, Y. Zhou, L. Xia, Q. Zheng, J. Liao, F. Xie and D. Lin, Rational design of multidimensional N-doped porous carbon/MoS2/CNTs nano-architecture hybrid for high performance lithium-sulfur batteries, J. Mater. Chem. A, 2018, 6(28), 13835–13847 RSC.
-
Q. Chen, Synthesis of metal sulfides/graphene composites and their electrochemical lithium storage performance, Doctoral dissertation, Zhejiang University, 2016.
- Y. You, Y. Ye, M. Wei, W. Sun, Q. Tang, J. Zhang, H. Li and J. Xu, Three-dimensional MoS2/rGO foams as efficient sulfur hosts for high-performance lithium-sulfur batteries, Chem. Eng. J., 2019, 355, 671–678 CrossRef CAS.
- J. Huo, Y. Xue, X. Zhang and S. Guo, Hierarchical porous reduced graphene oxide decorated with molybdenum disulfide for high-performance supercapacitors, Electrochim. Acta, 2018, 292, 639–645 CrossRef CAS.
- D. W. Maru, K. Zeng, M. Zhang, Y. Li and Y. Liu, Flower-like molybdenum disulfide/carbon nanotubes composites for high sulfur utilization and high-performance lithium-sulfur battery cathodes, Appl. Surf. Sci., 2019, 473, 540–547 CrossRef.
- S. Jiang, M. Chen, X. Wang, Z. Wu, P. Zeng, C. Huang and Y. Wang, MoS2-coated N-doped mesoporous carbon spherical composite cathode and CNT/Chitosan modified separator for avanced lithium sulfur batteries, ACS Sustainable Chem. Eng., 2018, 6(12), 16828–16837 CrossRef CAS.
- S. Wang, B. Guan, L. Yu and X. Lou, Rational design of three-layered TiO2@Carbon@MoS2 hierarchical nanotubes for enhanced lithium storage, Adv. Mater., 2017, 29(37), 1702724 CrossRef PubMed.
- J. Lee, J. Kim and T. Hyeon, Synthesis of new nanostructured carbon materials using silica nanostructured templates by Korean research groups, Int. J. Nanotechnol., 2006, 3(2/3), 253–279 CrossRef CAS.
- X. Li, K. Zhao, L. Zhang, Z. Ding and K. Hu, MoS2-decorated coaxial nanocable carbon aerogel composites as cathode materials for high performance lithium sulfur batteries, J. Alloys Compd., 2016, 692, 40–48 CrossRef.
- D. Zhang, Q. Wang, Q. Wang, J. Sun, L. Xing and X. Xue, High capacity and cyclability of hierarchical MoS2/SnO2 nanocomposites as the cathode of lithium-sulfur battery, Electrochim. Acta, 2015, 173, 476–482 CrossRef CAS.
- X. Xu, Z. Fan, S. Ding, D. Yu and Y. Du, Fabrication of MoS2 nanosheet@TiO2 nanotube hybrid nanostructures for lithium storage, Nanoscale, 2014, 6(10), 5245–5250 RSC.
|
This journal is © The Royal Society of Chemistry 2020 |
Click here to see how this site uses Cookies. View our privacy policy here.