Abstract
In this research, a novel magnetic sonocatalyst nanocomposite, CoFe2O4@Cr-MIL-101/Y zeolite, has been successfully fabricated employing a simple hydrothermal method. The as-prepared catalyst was thoroughly identified using Fourier transform infrared spectroscopy (FTIR), X-ray diffraction (XRD), field emission scanning electron microscopy (FESEM), energy dispersive X-ray spectroscopy (EDS), EDS elemental dot-mapping, transmission electron microscopy (TEM), atomic force microscopy (AFM), vibrating sample magnetometer (VSM), and nitrogen Brunauer–Emmett–Teller (N2-BET) analyses. The procured CoFe2O4@Cr-MIL-101/Y nanocomposite was then assessed for the decomposition of three types of organic dyes namely methylene blue (MB), rhodamine B (RhB) and methyl orange (MO) from water solution using ultrasound irradiation and subsequently monitored via UV-Vis absorption technique. The sonodecomposition reactions of organic dyes were accomplished in the presence of the H2O2 solution as a green oxidizing agent. Furthermore, the influence of various experimental independent factors such as irradiation time, process type, initial dye concentration, catalyst dosage, H2O2 concentration, scavenger type, and catalyst regeneration on the decomposition of MB, RhB and MO were surveyed. Additionally, a first order kinetic model was applied to investigate the sonodecomposition reactions of dye contaminants. The rate constant (k) and half-life (t1/2) data were gained as 0.0675 min−1 and 10.2666 min, respectively, for the decomposition of MB in the US/H2O2/CoFe2O4@Cr-MIL-101/Y system. Besides, evaluating the attained results, the distinctive performance of ˙OH as the radical scavenger originating from H2O2 throughout the sonodecomposition process is vividly approved.
3.9.1. The influence of the irradiation time. The irradiation time is an effective factor for the investigation of the cationic and anionic dyes sonodecomposition reactions using the CoFe2O4@Cr-MIL-101/Y zeolite catalyst. To meticulously evaluate these reactions, a period of time intervals was considered and the gained data studied to survey for all of the sonocatalytic experiments. Investigating the acquired results from the experiments clearly approved the remarkable dependency between the decomposition percentage and different reaction times. Thus, the sonodecomposition processes were done using the determined absorption bands ascribed to the MB organic dye at λmax about 663 nm. At first, the intended amount of CoFe2O4@Cr-MIL-101/Y zeolite catalyst (0.5 g L−1) was transferred into the MB aqueous media (50 mL, 25 mg L−1) and the resulted suspension was exposed to the US irradiation along with H2O2 (40 mmol L−1). As can be observed in Fig. 8a and b, the tangible differences between the gained UV-Vis analysis spectra of the MB aqueous media in the reaction with the CoFe2O4@Cr-MIL-101/Y zeolite catalyst indicates the prominent role of the shaking time. Based on the observations on the attained spectra it becomes crystal clear that the stringency of the punctuated peak assigned to MB (emerged at 663 nm) is directly affected by the time intervals. As time goes on, the intensity of the specified peak diminishes ceaselessly till the point of 60 min at which it becomes almost hard to be spotted which is connoting the high yield decomposition of MB (98.9%). According to the implemented sonocatalytic experiments and evaluated results on decomposition of MB dye, the particular visible light sono decomposition role of the CoFe2O4@Cr-MIL-101/Y zeolite nanocomposite is vividly confirmed.
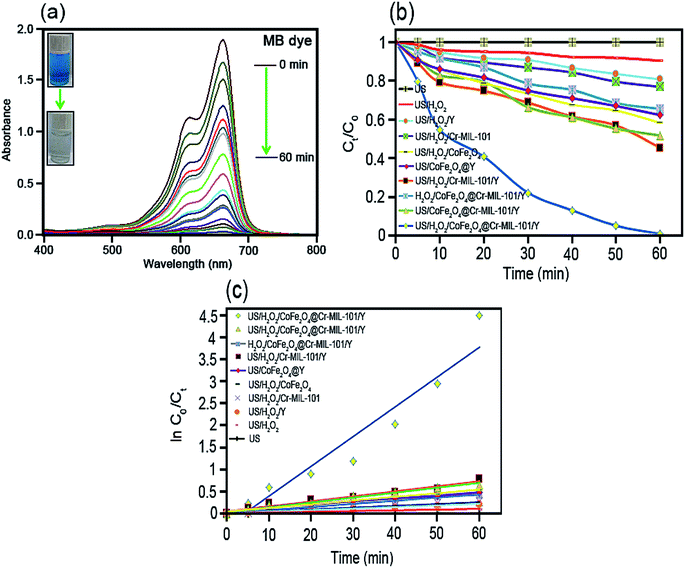 |
| Fig. 8 UV-Vis absorption spectra of the sonodecomposition process of MB dye over the CoFe2O4@Cr-MIL-101/Y catalyst nanocomposite as a function of the irradiation time (a and b), the first order kinetic plot (ln(C0/Ct)) against the irradiation time (c) (optimized experimental conditions; [MB dye]o: 25 mg L−1 (50 mL), [H2O2]: 40 mmol L−1 (2 mL), [catalyst dosage]: 0.5 g L−1, pH: 7 and temperature: 25 ± 1 °C). | |
As it has been displayed in Fig. 8c, plotting ln initial dye concentration versus contact time (min) results in some curves which are of great significance for the investigation of the sonodecomposition reaction kinetics. Elsewhere, the decomposition rate constant symbolized as k (min−1), was calculated using the first order equation of
In the represented equation,
Co is defined as the initial concentration of the understudy dye,
Ct represents the dye concentration at time interval of
t and
k symbolizes the decomposition rate constant. Meanwhile, the half-life (
t1/2) amount was also computed employing the equation of
To specify the mentioned adsorption kinetics, the value of 0.5 g L
−1 of CoFe
2O
4@Cr-MIL-101/Y zeolite was introduced to several containers each comprising 25 mg L
−1 of the considered dye at certain time periods and the subsequent relevant outcomes were demonstrated in
Table 3. To provide an overview on the potentials and workability of each participant component in the decomposition reaction of MB dye, several systems of US, US/H
2O
2, US/H
2O
2/Y, US/H
2O
2/Cr-MIL-101, US/H
2O
2/CoFe
2O
4, US/CoFe
2O
4@Y, US/H
2O
2/Cr-MIL-101/Y, H
2O
2/CoFe
2O
4@Cr-MIL-101/Y, US/CoFe
2O
4@Cr-MIL-101/Y, and US/H
2O
2/CoFe
2O
4@Cr-MIL-101/Y were designed and employed as the relevant results have been represented in
Fig. 8a–c. In addition, the value of 25 mg L
−1 as the initial concentration of MB dye was appointed for all of those specified experiments. In brief, the collected information from the implemented experiments revealed a decomposition sequence consisting of US/H
2O
2/CoFe
2O
4@Cr-MIL-101/Y (98.9%) > US/H
2O
2/Cr-MIL-101/Y (54.5%) > US/CoFe
2O
4@Cr-MIL-101/Y (48.1%) > US/H
2O
2/CoFe
2O
4 (41.5%) > US/CoFe
2O
4@Y (37.56%) > H
2O
2/CoFe
2O
4@Cr-MIL-101/Y (34.38%) > US/H
2O
2/Cr-MIL-101 (23%) > US/H
2O
2/Y (19.1%) > US/H
2O
2 (9.55%) > US (only), with respective constant values of 0.0675, 0.0114, 0.0109, 0.0083, 0.0072, 0.0071, 0.0042, 0.0034, 0.0016, and 0.0000 min
−1 (see
Table 3). Pursuant to the analysis outcomes, US/H
2O
2/CoFe
2O
4@Cr-MIL-101/Y was recognized as the most operative system for the decomposition of MB which is related to the impeding of the electron–hole incorporation by the linkage among Y zeolite, Cr-MIL-101 and CoFe
2O
4. The obtained data illuminates that reaction components including CoFe
2O
4@Cr-MIL-101/Y, US and H
2O
2 undoubtedly exhibit great performance for the decomposition process of MB dye in aqueous solutions. Additionally, applying US along with H
2O
2 method, intensifies the emission of hydroxyl radicals leading to the effectively much higher decomposition efficiency of the desired dye. Also, this decomposition efficiency can be explained as the generation of more oxidizing radicals in the presence of a higher concentration of H
2O
2. On the other hand, it should be noted that the sonocatalytic decomposition process in the absence of H
2O
2 (here focusing on US/CoFe
2O
4@Cr-MIL-101/Y) occurs most probably
via the formation of ˙OH radicals using the degradation of H
2O molecules. In contrast, when H
2O
2 as oxidizing agent was appended to the understudy systems, the generation of ˙OH radicals were considerably accelerated related to the degradation of H
2O
2 via the generated electrons on the catalysts surface. According to what specified above, it is deduced that US/H
2O
2/CoFe
2O
4@Cr-MIL-101/Y, US/CoFe
2O
4@Cr-MIL-101/Y and H
2O
2/CoFe
2O
4@Cr-MIL-101/Y systems have the same mechanism for the degradation of dyes.
Table 3 The attained data for the MB sonodecomposition reactions via different processes (optimized experimental conditions; irradiation time: 60 min, [MB]o: 25 mg L−1 (50 mL), [H2O2]: 40 mmol L−1 (2 mL), [catalyst dosage]: 0.5 g L−1, pH: 7 and temperature: 25 ± 1 °C)
Process type |
Rate constant (k, min−1) |
Half-life (t1/2, min) |
Kinetic equation [ln Co/Ct = at + b] |
US only |
0.0000 |
0.0000 |
y = 0.0000 |
US/H2O2 |
0.0016 |
433.1250 |
y = 0.0016x + 0.0112 |
US/H2O2/Y |
0.0034 |
203.8235 |
y = 0.0034x + 0.0076 |
US/H2O2/Cr-MIL-101 |
0.0042 |
165.0000 |
y = 0.0042x + 0.0145 |
US/H2O2/CoFe2O4 |
0.0071 |
97.6056 |
y = 0.0071x + 0.0090 |
US/CoFe2O4@Y |
0.0072 |
96.2500 |
y = 0.0072x + 0.0486 |
US/H2O2/Cr-MIL-101/Y |
0.0114 |
60.7894 |
y = 0.0114x + 0.0525 |
H2O2/CoFe2O4@Cr-MIL-101/Y |
0.0083 |
83.4939 |
y = 0.0083x + 0.0451 |
US/CoFe2O4@Cr-MIL-101/Y |
0.0109 |
63.5779 |
y = 0.0109x + 0.0378 |
US/H2O2/CoFe2O4@Cr-MIL-101/Y |
0.0675 |
10.2666 |
y = 0.0675x − 0.2700 |