DOI:
10.1039/D0RA01807D
(Paper)
RSC Adv., 2020,
10, 18054-18061
An ultra-sensitive T2-weighted MR contrast agent based on Gd3+ ion chelated Fe3O4 nanoparticles
Received
25th February 2020
, Accepted 24th April 2020
First published on 12th May 2020
Abstract
An ultra-sensitive T2-weighted MR imaging contrast agent was prepared based on Fe3O4 nanoparticles and Gd3+ ions (Fe3O4@Gd). Amino modified Fe3O4 nanoparticles were conjugated to diethylenetriamine pentaacetic acid, and finally coordinated with Gd3+ ions. The nanoparticles had a uniform morphology with a size of 100 nm and a Gd/Fe mass ratio of 1/110. The r2 (transverse relaxivity) of the Fe3O4 nanoparticles increased from 131.89 mM−1 s−1 to 202.06 mM−1 s−1 after coordination with Gd3+ ions. MR measurements showed that the aqueous dispersion of Fe3O4@Gd nanoparticles had an obvious concentration-dependent negative contrast enhancement. Hepatoma cells were selected to test the cytotoxicity and MR imaging effect. The application of Fe3O4@Gd nanoparticles as contrast agents was also exploited in vivo for T2-weighted MR imaging of rat livers. All the results showed the effectiveness of the nanoparticles in MR diagnosis.
Introduction
Early and highly accurate diagnosis has great significance in modern medicine.1 Magnetic resonance imaging (MRI) has been the most powerful and effective diagnostic method due to its many advantages, such as few side effects and non-invasive properties.2–4 MR contrast agents have played an important role in improving sensitivity by enhancing the contrast between normal tissue and diseased tissue.5 The commonly used MR contrast agents are divided into T1-positive agents, such as Gd chelates, and T2-negative agents, such as superparamagnetic iron oxide Fe3O4 nanoparticles.6
High transversal relaxation is necessary for MR contrast agents. For the past few years, a lot of effort has been devoted to the development of novel ultra-sensitive contrast agents.5,7,8 Some researchers tried to use materials of high saturation magnetization (Ms) as an alternative, such as MnFe2O4 and CoFe2O4.9,10 Because the size, shape and surface modification affected the saturation magnetization of nanoparticles, nanoparticles with cube, octapod, rod and other non-spherical shapes had been also exploited as ultrasensitive contrast agents.11,12 In addition to the intrinsic materials properties, r2 enhancement can also be realized by developing aggregates or clusters of magnetic nanoparticles.13–15 Recently, Huang et al. enhanced the transversal relaxation of Fe3O4 nanoparticles by using Gd3+-chelated mesoporous silica shells.16 Enlightened by this, we designed a facile strategy to prepare nanoparticles based on Fe3O4 nanoparticles and Gd3+ ions. It is well known that Gd3+ ions have well coordination with diethylenetriaminepentaacetic acid (DTPA), so many scientists are working on how to conjugate DTPA to Fe3O4 nanoparticles. To achieve this goal, chemicals contain amino group have been used to modify Fe3O4 nanoparticles and then coupled to DTPA via chemical conjugation or electrostatic interaction, such as (3-aminopropyl)triethoxysilane (APS), dopamine, chitosan and polyethyleneimine (PEI).16–18 Here amino group modified Fe3O4 nanoparticles were hydrothermal synthesized and then the nanoparticles were further modified by DTPA, which enabled the following chelation with Gd3+ ions. Besides, the relaxivity increased from 131.89 mM−1 s−1 of Fe3O4 to 202.06 mM−1 s−1 of Fe3O4@Gd nanoparticles while the previous publications reported Gd3+ chelated Fe3O4 nanoparticles always resulted in a decrease of relaxivity.19
The morphology, size and magnetic properties of the formed Fe3O4@Gd nanoparticles were characterized by TEM (transmission electron microscopy), DLS (dynamic light scattering) and VSM (vibrating sample magnetometer). The cytotoxicity and cellular uptake were also investigated by 3-[4,5-dimethylthiazol-2-yl]-2,5-diphenyl tetrazolium bromide (MTT) assay and Prussian blue staining method. The effectiveness of Fe3O4@Gd nanoparticles as MR contrast agents was investigated both in vitro and in vivo.
Materials and methods
Chemicals and reagents
MTT, iron(III) chloride hexahydrate (FeCl3·6H2O), DTPA, gadolinium(III) nitrate hexhydrate [Gd(NO3)3·6H2O], N-(3-dimethylaminopropyl)-N′-ethyl carbodiimide hydrochloride (EDC·HCl) and N-hydroxysuccinimide (NHS) were purchased from Aladdin (Shanghai, China). HepG2 (liver hepatocellular carcinoma) cells were purchased from the Type Culture Collection of the Chinese Academy of Sciences (Shanghai, China). Sprague-Dawley rats (200–220 g) were purchased from Anhui Experimental Animal Center (Hefei, China).
Preparation of Fe3O4@Gd nanoparticles
Amino modified Fe3O4 nanoparticles are prepared according to Li et al.'s publication.20 Briefly, 6.5 g 1,6-hexanediamine, 2.0 g anhydrous sodium acetate and 1.0 g FeCl3·6H2O were dissolved in 30 mL glycol and then transferred into a Teflon lined autoclave and kept at 190 °C for 6 h. The products were then washed with ethanol for many times. And then the nanoparticles are reacted with DTPA with the presence of EDC and NHS in MES buffer under room temperature for 72 h. After that, Gd(NO3)3 were added to the mixture and then stirred for another 24 h. Finally, the nanoparticles were collected by centrifugation and rinsed with water for many times.
Characterization of nanoparticles
The morphology of Fe3O4 nanoparticles and Fe3O4@Gd nanoparticles were characterized by TEM (JEOL, JEM-2100F). DLS measurement was determined by Zetasizer (Nano series, Malvern Instruments). The magnetic measurement was carried out using VSM (MPMS SQUIDM, USA). T2-weighted MR imaging of Fe3O4 nanoparticles and Fe3O4@Gd nanoparticles were measured by an MR scanner at 3.0 T (GE, Milwaukee, USA) as follows: echo time (TE) = 20, 40, 60, 80, 100 and 120 ms; repetition time (TR) = 3000 ms; field of view (FOV) = 20 mm × 20 mm; slice thickness = 4.0 mm; matrix = 320 × 320. IR characterization was performed by an IR spectrometer (Nicolet iN10, Thermo scientific, USA).
In vitro study
Cell culture. HepG2 cells were cultured in RPMI 1640 medium with 10% fetal bovine serum (FBS), 100 mg mL−1 streptomycin, and 100 U mL−1 penicillin at 37 °C under 5% CO2.
Cytotoxicity study. For MTT study, HepG2 cells were seeded on a 96-well plate at a density of 1 × 104 cells per well with. After 24 h, the culture medium was replaced with 100 μL of medium containing 0–40 μg mL−1 of Fe3O4@Gd nanoparticle. The cytotoxicity was evaluated by determining the cell viability after incubation for 24 h.
Prussian blue staining. HepG2 cells (5 × 105) were seeded in 6-well plates and then Fe3O4@Gd nanoparticles was added with the concentration range of 0–40 μg mL−1. After 24 h, the cells were then treated with 4% formaldehyde for 30 min. Then 5% potassium ferrocyanide and 5% hydrochloric acid was added. Finally, cells were imaged by a microscope (Olympus, IX71).
MRI investigation. HepG2 cells (5 × 105) were plated in 6-well plates and then Fe3O4@Gd nanoparticles were added at a concentration of 0–40 μg mL−1. After incubation for 24 h, the cells were collected and re-suspended in 0.5% agarose gel and then imaged by using a 3.0 T MR system (Discovery MR750w, GE Medical Systems, Milwaukee, WI, USA).
In vivo study
Animals. Sprague-Dawley rats (200–220 g) were purchased from the Anhui Experimental Animal Center with the license number “SCXK (Wan) 2011-002”. The rats were housed under at 22 °C with a 12 h light/dark cycle and were allowed free access to food and water. All animal procedures were performed in accordance with the Guidelines for Care and Use of Laboratory Animals of Anhui Medical University and approved by the Animal Ethics Committee of Anhui Medical University.
Prussian blue staining. Liver, heart, spleen, kidney and lung tissues freshly removed from Fe3O4@Gd nanoparticles treated rat were fixed in 4% paraformaldehyde and processed routinely into paraffin, sectioned at a thickness of 3 μm, brain sections were then stained with Prussian blue and counterstained with eosin. Finally, the sections were imaged by a microscope (Olympus, IX71).
MRI investigation. For in vivo MR investigation, SD rats were tail vein injected with Fe3O4@Gd nanoparticles (1 mL, 10 mg per kg of rat body weigh). The rats were imaged before and at 0.5 h, 1 h, and 24 h post injection. T2-weighted MR images were obtained with a matrix size of 256 × 256, slice thickness of 2 mm, and field of view of 60 mm × 60 mm. The signal intensity was also measured.
Results
Materials characterization
Fe3O4@Gd nanoparticles were fabricated by three steps (Scheme 1). First, amino group modified Fe3O4 nanoparticles were hydrothermal synthesized. Second, DTPA were conjugated to amino group through amide bond. Finally, Gd3+ ions were chelated with DTPA to form Fe3O4@Gd nanoparticles. Fig. 1a and b showed the TEM images of Fe3O4 nanoparticles and Fe3O4@Gd nanoparticles, respectively. There was no obvious change between the morphology of the two samples, which had a size ranging from 10–50 nm. To confirm the element composition, energy-dispersive X-ray (EDX) element mapping was performed on a single nanoparticle (Fig. 1c). The results suggested that Gd element is present in the nanoparticle. Inductively coupled plasma atomic emission spectroscopy (ICP-AES) analysis was employed to quantify Fe and Gd content and the results showed the mass ratio of Gd/Fe is 1
:
110. According to the ICP analysis results, each nanoparticles have conjugated to 1.48 × 104 DTPA molecules.
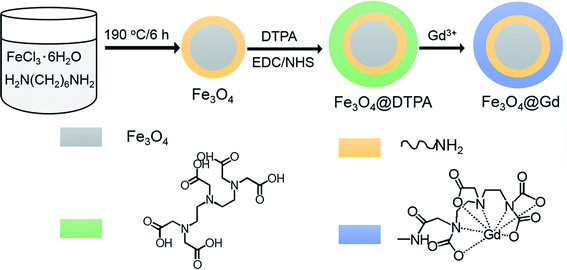 |
| Scheme 1 Schematic illustration of the formation of Fe3O4@Gd nanoparticles. | |
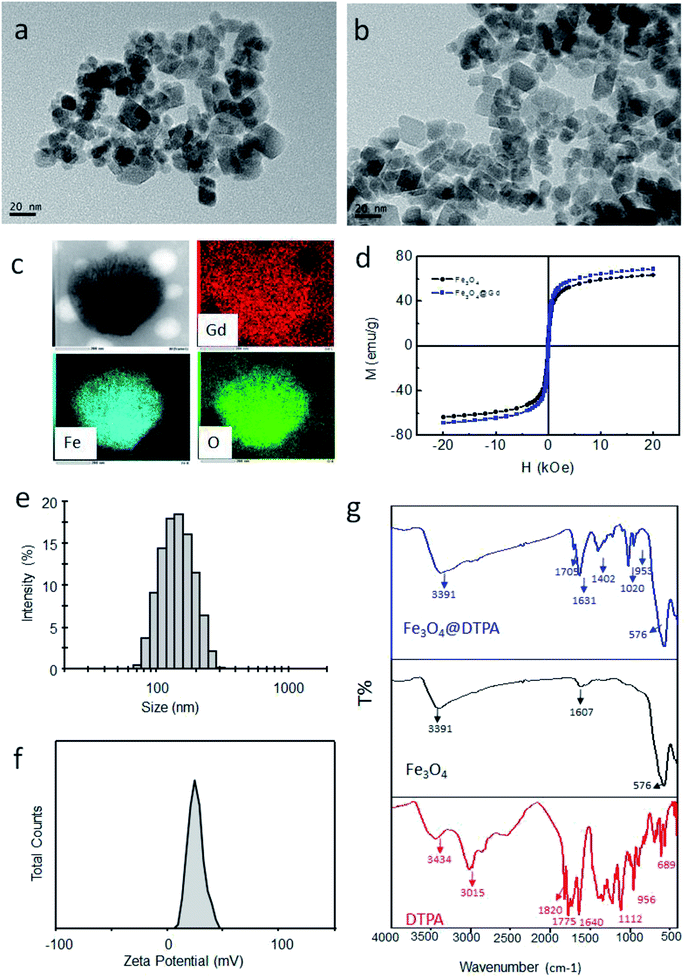 |
| Fig. 1 Characterization of nanoparticles. (a) TEM image of Fe3O4 nanoparticles; (b) TEM image of Fe3O4@Gd nanoparticles; (c) EDX mapping images of one nanoparticle; (d) the magnetic hysteresis loops of Fe3O4 and Fe3O4@Gd nanoparticles at room temperature; (e) size distribution of Fe3O4@Gd nanoparticles; (f) zeta potential of Fe3O4@Gd nanoparticles; (g) IR spectra of Fe3O4@DTPA nanoparticles, Fe3O4 nanoparticles and DTPA. | |
The magnetic properties of the nanoparticles were measured at room temperature. As shown in Fig. 1d, the saturation magnetization value of Fe3O4 and Fe3O4@Gd nanoparticles are 63.35 emu g−1 and 68.65 emu g−1, respectively. After chelated with Gd3+ ions, the saturation magnetization increased. Besides, both the values were lower than that of bulk Fe3O4, which was reasonable because the particle size decreased.20 The remanence and coercivity of both the samples were nearly zero, suggesting the superparamagnetic property of the nanoparticles.
DLS measurements were carried out to measure the size and zeta potential values of the obtained nanoparticles. The hydrodynamic diameter histogram and the zeta potential distribution were presented in Fig. 1e and f. It was noticed that the hydrodynamic size of the nanoparticles was kept around 130 nm, which was larger than that obtained from TEM observation. This might be explained that the small nanoparticles aggregated slightly. However, the aggregates still had a proper size less than 200 nm, which was still proper for bio-applications. It was also worth mentioned that the obtained nanoparticles possessed a zeta potential of +17 mV, which was critical for formation of stable dispersions.
Fig. 1g shows the IR spectra of Fe3O4 nanoparticles before and after conjugated to DTPA and free DTPA. It can be see clearly that all the peaks of DTPA are appeared in the spectrum of the conjugated nanoparticles (Fe3O4@DTPA) with suppressed intensity. The adsorption peak at around 576 cm−1 was the characteristic absorption of Fe–O bond. Besides, there is a new peak at 1704 cm−1, which is ascribed to C
O group of the formatted amide bond. All of these confirmed that DTPA is successfully conjugated to the surface of Fe3O4 nanoparticles via amide bond.
T2 MR relaxometry
To investigate the possibility of Fe3O4@Gd nanoparticles as T2-weighted contrast agents, MRI investigation were carried. Fig. 2 showed the T2-weighted MR images of Fe3O4 and Fe3O4@Gd nanoparticles with various Fe concentrations under different TE time. We observed that the T2-weighted MR signal decreased with the increase of Fe concentration. Fe3O4@Gd nanoparticles exhibited much higher T2-negative contrast than Fe3O4 nanoparticles. The scatter diagram of relaxation rate vs. concentration was linear fitted and the slope was equal to the transverse relaxivities r2. The r2 value of Fe3O4@Gd nanoparticles was measured to be 202.06 mM−1 s−1, higher than that of Fe3O4 nanoparticles without Gd3+ ions coordination.
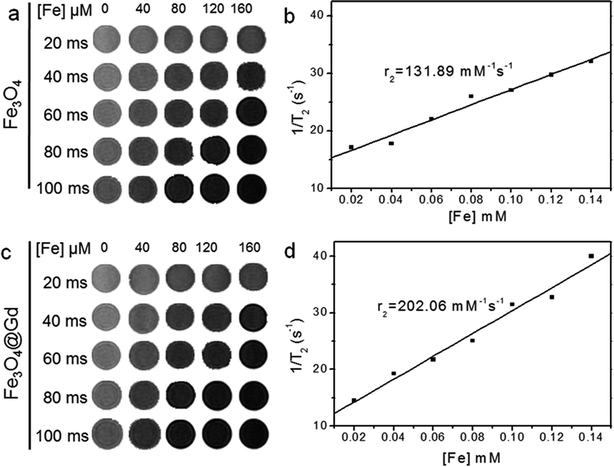 |
| Fig. 2 T2-weighted MR images at different Fe concentration in 0.5% agarose gel at different TE time and the analysis of relaxation rate vs. Fe concentration. (a and b) Fe3O4 nanoparticles. (c and d) Fe3O4@Gd nanoparticles. | |
Cytotoxicity and cellular uptake studies
The biocompatibility is a critical parameter for materials in biomedical application. MTT assay was performed to evaluate the cell viability of HepG2 cells incubated with Fe3O4@Gd nanoparticles. As shown in Fig. 3, there was no obvious concentration-effect observed in [Fe] range of 50–200 μg mL−1. After co-incubated with Fe3O4@Gd nanoparticles for 24 h, the cell viability with the highest concentration up to 200 μg mL−1 had no significant difference. This suggested that Fe3O4@Gd nanoparticles were very low toxic at the tested concentration range, which is beneficial for the further bio-medical applications.
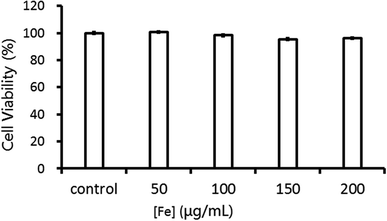 |
| Fig. 3 Cell viability of HepG2 cells incubated with Fe3O4@Gd nanoparticles at various Fe concentrations. | |
To study the cellular uptake of Fe3O4@Gd nanoparticles, HepG2 cells incubated with Fe3O4@Gd nanoparticles with various concentrations were stained by Prussian blue and Eosin. Iron can be stained by Prussian blue and show blue color. As shown in Fig. 4a, HepG2 cells incubated with DMEM exhibited pink color. After incubated with Fe3O4@Gd nanoparticles, blue color were observed because of the internalized nanoparticles. The blue color also showed concentration-effect, as the concentration increased, the blue color became stronger. It was noteworthy that the blue color was located in the cytoplasm instead of nucleus, which suggested the internalized Fe3O4@Gd nanoparticles were mainly distributed in cytoplasm.
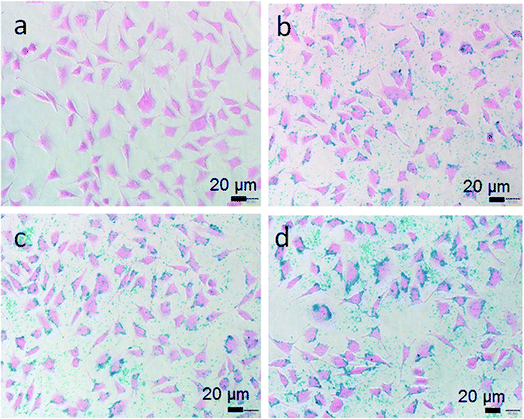 |
| Fig. 4 Prussian blue staining images of HepG2 cells incubated with Fe3O4@Gd nanoparticles at various iron concentration ((a) – control, (b) – 10 μg mL−1, (c) – 20 μg mL−1, (d) – 30 μg mL−1). | |
The MR imaging ability of Fe3O4@Gd nanoparticles was first performed in vitro. HepG2 cells were incubated with Fe3O4@Gd nanoparticles with various concentrations for 24 h and then collected for MR scanning. As shown in Fig. 5a, HepG2 cells after incubated with Fe3O4@Gd nanoparticles displayed a negative enhancement. The MR images of HepG2 cells darkened gradually as Fe concentration increased. HepG2 cells exposed to Fe3O4@Gd nanoparticles exhibited an obvious signal decrease even at the lowest concentration 5 μg mL−1 compared with control cells. When iron concentration greater than 20 μg mL−1, the signal intensity was almost keep stable. This demonstrated that the uptake of Fe3O4@Gd nanoparticles of HepG2 cells was nearly saturated.
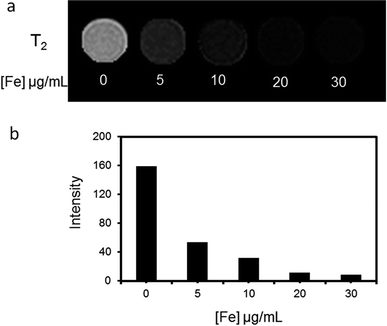 |
| Fig. 5 MR investigation in vitro. (a) T2-weighted MR images of HepG2 cells incubated with Fe3O4@Gd nanoparticles with iron concentration ranging from 0–30 μg mL−1 and (b) the corresponding analysis of MR signal intensity. | |
MR investigation
High r2 relaxivity, low toxicity and good imaging effect in vitro encouraged us to explore there in vivo T2-weighted MR imaging capability. 10 mg Fe3O4@Gd nanoparticles (1 mL, 10 mg per kg of rat body weight) were injected into the SD rat via tail vein. After vein tail injection of our nanoparticles, MR scanning was performed. Fig. 6 showed T2-weighted MRI images of rat liver collected from different time. After injection 30 min, MR images of liver showed slightly changes. The signal intensity of the corresponding T2-weighted images was plotted in Fig. 6e. As long prolonged, the T2 MR signal intensity of liver darkened gradually compared with that before injection. At 24 h post injection, the rat liver showed the lowest signal intensity in T2-weighted MR images. This result demonstrated that the negative enhancement of MRI could be achieved by using our Fe3O4@Gd nanoparticles as contrast agents.
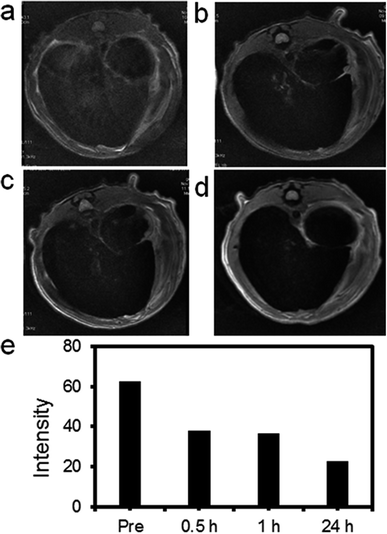 |
| Fig. 6 MR images of rat liver before and after vein tail injection of Fe3O4@Gd nanoparticles (10 mg kg−1). (a) Pre-injection; (b) 30 min after injection; (c) 60 min after-injection; (d) 24 h after injection; (e) the corresponding T2-weighted MR signal intensity of the liver. | |
In vivo biodistribution
Prussian blue staining was used to investigate the in vivo biodistribution of the Fe3O4@Gd nanoparticles. At 24 h post-injection, different organs including heart, liver, spleen, lung, and kidney were harvested and then stained by Prussian blue and Eosin (Fig. 7). As shown Fig. 7a and magnified Fig. 7b, it is obvious that large amounts of nanoparticles distributed in the liver, which suggested large amount of Fe3O4@Gd uptake in the liver. A relatively small amount of Fe3O4@Gd uptake can also be found in the other organs, such as lung and kidney. There is almost no Fe3O4@Gd uptake in heart and lung.
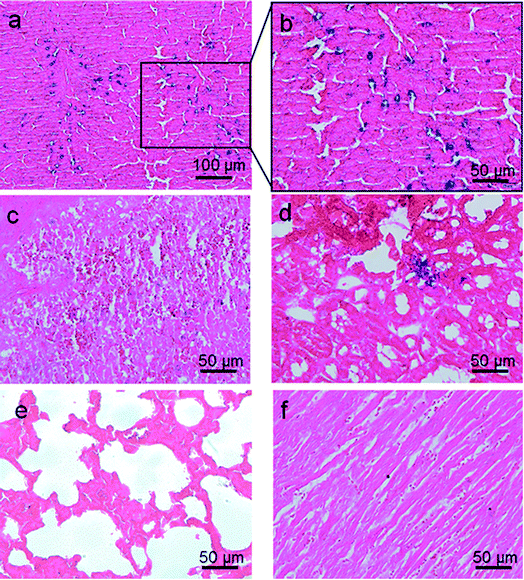 |
| Fig. 7 Prussian blue staining images of the major organs of SD rats ((a and b) – liver, (c) – spleen, (d) – kidney, (e) – lung and (f) – heart). The sections were acquired from rat at 24 h after intravenous injection of 10 mg kg−1 Fe3O4@Gd nanoparticles. | |
Discussion
Here we prepared Gd3+ ions chelated Fe3O4 nanoparticles as contrast agent for T2-weighted MR imaging. Between the two components, one is T1 positive contrast agent and the other is T2 negative contrast agent. For the previous publications, the integration of these two components for MR contrast agents is mainly utilized as T1- and T2-dual mode MR contrast agent.21–23 For instance, Bae, et al. reported a dual mode contrast agent Gd(DTPA) labelled IONP has r2 value of 30.32 mM−1 s−1 and r1 value of 11.17 mM−1 s−1 at 3.0 T with similar size.19 Thorat et al. reported a multifunctional gadolinium-doped iron oxide nanoparticles for targeted magnetic hyperthermia, chemotherapy and T1–T2 dual-model magnetic resonance (MR) imaging with r2 value of 50.47 mM−1 s−1 and r1 value of 1.55 mM−1 s−1 at 3.0 T.24
A similar formulation based on Fe3O4 and Gd3+ has also been reported, Gd3+ ions were introduced via three different methods including direct complex formation, covalent attachment and electrostatically and the results indicated only the nanoparticles obtained by the following two methods work effective.25 Here Fe3O4@Gd nanoparticles were prepared by a very facile conjugate method. Amino modified Fe3O4 nanoparticles were prepared by one-pot hydrothermal synthesis method and then coupled to DTPA and further chelated with Gd3+ ions. The r2 of Fe3O4@Gd nanoparticles (202.06 mM−1 s−1) is higher than that of Fe3O4 nanoparticles (131.89 mM−1 s−1). However, the introduction of Gd3+ has no obvious contribution for the enhancement of longitudinal relaxivity r1, which is only 1 mM−1 s−1. This may be explained by the low content of Gd in our nanoparticles. This different influence of Gd3+ to longitudinal relaxivity and transverse relaxivity are also found by Huang et al.16 Recently, some scientists proposed to classify a contrast agent with r2/r1 > 10 as T2 agents.26 Therefore, we named the Fe3O4@Gd nanoparticles as T2 contrast agents. The reason why transverse relaxivity increased are not clear.
There are many factors affect the relaxivities, such as particle size, aggregation and surface modification. Therefore it is difficult to compare our nanoparticle to other formulation. However, the r2 relaxivity of Fe3O4@Gd nanoparticles is higher than that of commercialized Feridex r2 (148.95 mM−1 s−1),19 suggesting their T2 contrast effect was strong enough to be qualified as T2-weighted MR contrast agents.
It is important to understand the biodistribution of the fabricated Fe3O4@Gd nanoparticles for their bio-medical applications. The particles are mainly distributed in the liver without further modification, which is accordant with the imaging results in vivo MR. Besides, the nanoparticles are also found in spleen and kidney. The presence of Fe3O4@Gd nanoparticles in the kidney may suggest the nanoparticles could be cleared by kidney. Further pharmacokinetic studies of the nanoparticles at different time are necessary for a full understanding of the biodistribution.
Conclusions
A new kind of T2-weighted MR imaging contrast agents based on Fe3O4 nanoparticles and Gd3+ ions were prepared. The introduction of Gd3+ increased the transverse relaxation rate of Fe3O4 nanoparticles but still maintained their good biocompatibility. Both in vitro and in vivo studies showed the nanoparticles had an obvious MR enhancement.
Conflicts of interest
The authors declare no conflict of interest.
Acknowledgements
This work was funded by the National Natural Science Foundation of China (Grant No. 51302004 to M.-M. S.), Anhui Provincial Natural Science Foundation of China (Grant No. 1308085QH141 to M.-M. S.) and University Natural Science Research Project of Anhui Province (Grant No. KJ2018A0172 to Y.-K. W.).
References
- R. L. Siegel, K. D. Miller and A. Jemal, Ca-Cancer J. Clin., 2020, 70, 7–30 CrossRef PubMed.
- O. Gobbo, K. Sjaastad, M. Radomski, Y. Volkov and A. Prina-Mello, Theranostics, 2015, 5, 1249–1263 CrossRef PubMed.
- M. Busquets, J. Estelrich and M.-J. Sánchez-Martín, Int. J. Nanomed., 2015, 10, 1727 CrossRef PubMed.
- R. Weissleder and M. Pittet, Nature, 2008, 452, 580–589 CrossRef PubMed.
- E. Boros, E. M. Gale and P. Caravan, Dalton Trans., 2015, 44, 4804–4818 RSC.
- Z. Li, P. Yi, Q. Sun, H. Lei, H. Zhao, Z. Zhu, S. Smith, M. Lan and M. Lu, Adv. Funct. Mater., 2012, 22, 2387–2393 CrossRef CAS.
- Z. Shen, A. Wu and X. Chen, Mol. Pharm., 2017, 14, 1352–1364 CrossRef PubMed.
- W. Tang, Z. Zhen, C. Yang, L. Wang, T. Cowger, H. Chen, T. Todd, K. Hekmatyar, Q. Zhao, Y. Hou and J. Xie, Small, 2014, 10, 1245–1249 CrossRef PubMed.
- J.-H. Lee, Y.-M. Huh, Y.-w. Jun, J.-W. Seo, J.-T. Jang, H.-T. Song, S. Kim, E.-J. Cho, H.-G. Yoon, J.-s. Suh and J. Cheon, Nat. Med., 2007, 13, 95–99 CrossRef PubMed.
- H. B. Na, I. C. Song and T. Hyeon, Adv. Mater., 2009, 21, 2133–2148 CrossRef.
- Z. Zh, Z. Zhou, J. Bao, Z. Wang, J. Hu, X. Chi, K. Ni, R. Wang, X. Chen, Z. Chen and J. Gao, Nat. Commun., 2013, 4, 2266 CrossRef PubMed.
- S.-H. Noh, W. Na, J.-T. Jang, J.-H. Lee, E. Lee, S. H. Moon, Y. Lim, J.-S. Shin and J. Cheon, Nano Lett., 2012, 12, 3716–3721 CrossRef PubMed.
- K. Hayashi, M. Nakamura, W. Sakamoto, T. Yogo, H. Miki, S. Ozaki, M. Abe, T. Matsumoto and K. Ishimura, Theranostics, 2013, 3, 366–376 CrossRef PubMed.
- M. Wu, D. Zhang, Y. Zeng, L. Wu, X. Liu and J. Liu, Nanotechnol, 2015, 26, 115102 CrossRef PubMed.
- M.-Y. Fei, M.-M. Song, P. Wang, G.-z. Pang, J. Chen, D.-P. Lu, R. Liu, G.-Y. Zhang, T.-T. Zhao and Y.-Q. Yu, RSC Adv., 2020, 10, 5294–5303 RSC.
- C. C. Huang, C. Y. Tsai, H. S. Sheu, K. Y. Chuang, C. H. Su, U. S. Jeng, F. Y. Cheng, C. H. Su, H. Y. Lei and C. S. Yeh, ACS Nano, 2011, 5, 3905–3916 CrossRef PubMed.
- H. Yang, Y. Zhuang, Y. Sun, A. Dai, X. Shi, D. Wu, F. Li, H. Hu and S. Yang, Biomaterials, 2011, 32, 4584–4593 CrossRef CAS PubMed.
- K. Luo, J. Tian, G. Liu, J. Sun, C. Xia, H. Tang, L. Lin, T. Miao, X. Zhao, F. Gao, Q. Gong, B. Song, X. Shuai, H. Ai and Z. Gu, J. Nanosci. Nanotechnol., 2010, 10, 540–548 CrossRef CAS PubMed.
- K. H. Bae, Y. B. Kim, Y. J. Lee, J. Hwang, H. Park and T. G. Park, Bioconjugate Chem., 2010, 21, 505–512 CrossRef CAS PubMed.
- L. Wang, J. Bao, L. Wang, F. Zhang and Y. Li, Chem.–Eur. J., 2006, 12, 6341–6347 CrossRef CAS.
- F. Li, D. Zhi, Y. Luo, J. Zhang, X. Nan, Y. Zhang, W. Zhou, B. Qiu, L. Wen and G. Liang, Nanoscale, 2016, 8, 12826–12833 RSC.
- H. Cai, X. An, S. Wen, J. Li, G. Zhang, X. Shi and M. Shen, Part. Part. Syst. Charact., 2015, 32, 934–943 CrossRef CAS.
- N. Thorat, R. Bohara, H. Yadav and T. Syed, RSC Adv., 2016, 6, 94967–94975 RSC.
- N. Thorat, R. Bohara, S. Tofail, Z. Alothman, M. Shiddiky, S. Hossain, Y. Yamauchi and K. Wu, Eur. J. Inorg. Chem., 2016, 16, 4586–4597 CrossRef.
- A. Szpak, S. Fiejdasz, W. Prendota, T. Straczek, C. Kapusta, J. Szmyd, M. Nowakowska and S. Zapotoczny, J. Nanopart. Res., 2014, 16, 2678 CrossRef PubMed.
- W. Zhang, L. Liu, H. Chen, K. Hu, I. Delahunty, S. Gao and J. Xie, Theranostics, 2018, 8, 2521–2548 CrossRef CAS PubMed.
|
This journal is © The Royal Society of Chemistry 2020 |
Click here to see how this site uses Cookies. View our privacy policy here.