DOI:
10.1039/D0RA06153K
(Paper)
RSC Adv., 2020,
10, 39931-39942
Photocatalytic activity of micron-scale brass on emerging pollutant degradation in water: mechanism elucidation and removal efficacy assessment†
Received
15th July 2020
, Accepted 20th October 2020
First published on 2nd November 2020
Abstract
Alloys or smelted metal mixtures have served as cornerstones of human civilization. The advent of smelted copper and tin, i.e., bronze, in the 4th millennium B.C. in Mesopotamia has pioneered the preparation of other metal composites, such as brass (i.e., mixture of copper and zinc), since the bronze age. The contemporary use of these alloys has expanded beyond using their physical strength. The catalytic chemistry of micron-scale brass or copper–zinc alloy can be utilized to effectively degrade emerging contaminants (ECs) in water, which are presenting significant risks to human health and wildlife. Here, we examine the photocatalytic activity of a commercially available micro-copper–zinc alloy (KDF® 55, MicroCuZn), made with earth abundant metals, for oxidative removal of two ECs. The micron-scale brass is independently characterized for its morphology, which confirms that it has the β-brass phase and that its plasmonic response is around 475 nm. Estriol (E3), a well-known EC, is removed from water with ultraviolet (UV) radiation catalyzed by MicroCuZn and H2O2–MicroCuZn combinations. The synergy between H2O2, UV, and MicroCuZn enhances hydroxyl radical (˙OH) generation and exhibit a strong pseudo-first-order kinetic degradation of E3 with a decay constant of 1.853 × 10−3 min−1 (r2 = 0.999). Generation of ˙OH is monitored with N,N-dimethyl-4-nitrosoaniline (pNDA) and terephthalic acid (TA), which are effective ˙OH scavengers. X-ray photoelectron spectroscopy analysis has confirmed ZnO/CuO–Cu2O film formation after UV irradiation. The second EC studied here is Δ9-tetrahydrocannabinol or THC, a psychotropic compound commonly consumed through recreational or medicinal use of marijuana. The exceptionally high solids–water partitioning propensity of THC makes adsorption the dominant removal mechanism, with photocatalysis potentially supporting the removal efficacy of this compound. These results indicate that MicroCuZn can be a promising oxidative catalyst especially for degradation of ECs, with possible reusability of this historically significant material with environmentally-friendly attributes.
1. Introduction
Being present in trace amounts, pharmaceuticals, hormones, and personal care products manifest new challenges for water treatment.1 Although water treatment technologies are continuing to be developed for effective removal and degradation of a wide variety of Emerging Contaminants (ECs),2 some crucial challenges in these efforts have been identified, which include: high energy consumption, excess chemical inputs, large carbon and physical footprint, increased cost, and lack of reusability.3 There is a critical need for developing smart materials, which can be prepared with earth-abundant resources, to effectively remove ECs from water. One such material that has promise to overcome some of these limitations is brass, a bimetallic alloy made with copper (55–90%) and zinc (10–45%). Brass, a historically significant material that was invented in the bronze age and has served as one of the cornerstones of human civilization, also demonstrated unique advantages in environmental applications; e.g., as biocidal agents4 and in biofuel conversion,5 CO2 reduction,6 air pollution control,7 and water treatment.8,9
One of the cost-effective (average cost of USD 0.019 g−1) micron-scale brass is KDF® 55 (hereafter MicroCuZn), which has become popular in water treatment.10–15 MicroCuZn has extensively been applied in filters to remove manganese,16 chlorine,11 mercury,10,13 antimony(III),17 chromium(VI),12 copper(II),18 and silver nanoparticles.15 It has been suggested that the removal mechanism of heavy metals by MicroCuZn is via reduction of the metal and subsequent formation of amalgams.10 Even though MicroCuZn has been predominantly used for removal of inorganic ions, its efficacy for organic contaminant degradation has hardly been assessed; evaluation of its promise for the same is imperative.
The rationale for using MicroCuZn as an oxidative catalyst for organic degradation emanates from previous reports, where copper foil activated by ozone removed organic pollutants with hydroxyl radical (˙OH) attack.19 Similarly, when brass is exposed to hydrogen peroxide (H2O2),20 a common oxidant, or to photo-radiation,21,22 it can modulate reactive oxygen species (ROS) generation. H2O2 has also been shown to initiate corrosion by electroreduction.20 Besides, it has been confirmed that Cu, upon exposure to ultraviolet radiation (UV), forms a stable oxide film.23–25 The photo-corrosion could be brought about by the photo-induced holes (h+) that transport across the crystals within brass, accelerating the cathodic reactions.22
Emerging pharmaceuticals, particularly Δ9-tetrahydrocannabinol (THC) and estriol (E3), are structurally similar with the presence of a phenol moiety and with their relatively high octanol–water partition coefficients values, i.e., 106.97 and 102.45, respectively; were chosen as model contaminants. THC, the primary chemical compound in marijuana, is a psychotropic substance that belongs to the cannabinoid family.26–28 THC and similar cannabis molecules and their metabolites are suspected of transforming into toxic disinfection byproducts (DBPs) during their passage through the treatment systems.26,28 Similarly, E3 is released into the aquatic environment in notable amounts for their use in hormone replacement therapy,29 and is known to present severe risks to the environment and humans.30 An oxidative transformation of these pharmaceuticals can potentially break open the phenolic structures and reduce potential halogenation during their passage through the treatment systems. Ring-opening and chain-shortening reactions achieved using a potent oxidant can present with a promising route to avoid DBP generation.
The objective of this study is to elucidate the catalytic and photocatalytic mechanisms of a commercially available micron-scale brass to remove the two model ECs (with unique molecular structure and covering for a wide range of solids–water partition coefficient) from water. In particular, the catalytic mechanisms are examined with combinations of MicroCuZn and H2O2, with and without UV irradiation. Oxidative catalytic rate is measured, followed by elucidation of underlying mechanism with monitoring of ˙OH with N,N-dimethyl-p-nitrosoaniline (pNDA), and terephthalic acid (TA). To evaluate the efficacy of the material beyond its oxidative performance, reusability and stability are also examined.
2. Experimental
2.1. Materials
pNDA (97%), E3 (≥97%), TA (98%), and 2-hydroxyterephthalic acid (2-THA, 97%) were procured from Sigma-Aldrich (St. Louis, MO, USA). THC (97%) in methanol was obtained from Restek (Bellefonte, PA, USA). H2O2 (30%) was procured from J. T. Baker (Phillipsburg, NJ, USA). HPLC grade acetonitrile (99.99%) was purchased from Fisher Scientific (Fair Lawn, NJ, USA). All solutions and HPLC mobile phases were prepared with ultrapure water (Millipore, Burlington, MA, USA). These chemicals were reagent grade and used with no further purification.
KDF® 55 (MicroCuZn) was procured from KDF Fluid Treatment Inc. (Three Rivers, MI, USA). Fig. S1† shows MicroCuZn material, as used in this study. The MicroCuZn was ultrasonically washed with acetone, ethanol, and deionized (DI) water for 10 min each, and then dried at 90 °C for 6 h.
2.2. Material characterization
Surface morphology was examined with scanning electron microscopy (SEM) using a FEI Quanta FEG 650 SEM (FEI Company, Hillsboro, OR, USA), equipped with an energy dispersive X-ray spectroscopy (EDS) attachment (Bruker, Madison, WI, USA). Ferret's diameter, projected area, and circularity distributions were obtained from SEM images for 48 grains, chosen randomly. Transmission electron microscopy (TEM) images were collected with a JEOL 2010F TEM (Japan Electronics Co. Ltd, Tokyo, Japan). SEM and TEM images were analyzed with ImageJ (NIH, Bethesda, ML). X-ray diffraction (XRD) analysis was conducted on R-Axis Spider (Rigaku Corporation, Japan) diffractometer. The phase identification was conducted with QualX2.0 software, developed by Altomare et al.31 Nitrogen adsorption isotherms were generated at 77.4 K, with a Quantachrome Autosorb-1 gas adsorption analyzer to determine specific Brunauer–Emmett–Teller (BET) surface area (Quantachrome Instruments, Boynton Beach, FL, USA). Prior to analysis, the samples were outgassed at 293.15 K for 40 h. Diffused reflectance measurement was obtained by forming pellets and conducting measurements on a Lambda 365 UV-vis spectrophotometer (PerkinElmer, Waltham, MA, USA) with BaSO4 as the reference. X-ray photoelectron spectroscopy (XPS) was conducted with a Kratos AXIS Ultra DLD (Shimadzu Corporation, Japan), equipped with a monochromatic Al Kα (1486.6 eV) X-ray source. The C 1s peak at binding energy of 284.5 eV was used as an energy reference. XPS spectrum for Cu 2p3/2 region was deconvoluted into four components; i.e., copper metal (Cu(0)), cuprous oxide (Cu2O), cupric oxide (CuO) and copper hydroxide (Cu(OH)2) with regard to binding energy values reported earlier.32 The Zn-region was deconvoluted for Zn 2p3/2, taking into account two components; i.e., zinc metal (Zn(0)) and zinc oxide (ZnO), based on binding energies that have been extensively reported.33 The XPS spectra were fitted with XPS peak 4.1 software, following Raymund W. M. Kwok (The Chinese University of Hong Kong, China), with a 80
:
20 Gaussian–Lorenzian mixed-function having the Shirley background correction.
2.3. Batch photocatalytic conditions
The photoreactor was constructed with a 100 mL cylindrical water-cooled, jacketed glass vessel (Fig. S2a†), placed on a thermostatic bath (F250 Julabo, Seelbach, Germany) that maintained 20 °C for all experiments. To ensure that THC adsorption to the reactor wall does not interfere with the photocatalytic degradation assessment, future studies should explore reactor materials (such as silanized glass) with minimum THC sorption propensity. The initial concentrations of E3, THC, pNDA, and TA were 10, 1, 10 and 500 μM, respectively. All experiments were adjusted to pH 7.0 with 10 mM NaOH solutions, except for the TA solutions (i.e., pH 9.0) to ensure adequate TA solubility.34 pH 7.0 was selected because of copper's thermodynamic instability in deionized H2O (with dissolved oxygen) at pH below 7.0 according to the Pourbaix diagram for copper at 25 °C.35 Future studies may consider examining photocatalytic behavior of brass in alkaline media.
The system was set in an enclosed wooden box to avoid interference from ambient light (Fig. S2b†). The catalyst load was maintained at 10 g L−1. A high catalyst load was chosen due to its low superficial area (0.0149 m2 g−1), as reported elsewhere.18 When 0.1 M H2O2 was added to the test solutions, pH was adjusted to 7.0, prior to catalyst addition. The number of samples was selected to minimize perturbation in the total stock volume. We decided that the sample volume to be withdrawn should be less than 10% of the total volume.
The UV irradiation source was a 10 W Light Emitting Diode (LED, Epistar Co, Taipei, China). The diffraction spectrum of the lamp was obtained with a lab-made spectrophotometer,36 which showed that the LED had a narrow emission band between 363 nm and 415 nm with a primary peak at 382 nm (Fig. S2c†). Photon flux was measured with a ferrioxalate actinometer37 and was determined to be 9.9 μeinstein per min. Irradiation intensity was obtained with a digital radiometer (PMA 2100, Solar light, Glenside, PA, USA) with detectors for UV and visible range radiation (PMA 2107 and PMA 2140). The irradiation intensity measured was 4.7 W m−2, distributed 99.6% below 400 nm, which confirms emission occurring at the UV range based on the diffraction spectrum (Fig. S2c†).
2.4. Continuous photocatalytic packed reactor conditions
Reusability and stability were demonstrated through a continuous packed-bed photocatalytic reactor (Fig. S3†). The continuous reactor was a quartz column with dimensions of 1 mm × 10 mm × 100 mm (Fig. S3a†). The column was packed with 2.5 g MicroCuZn (Fig. S3†) and was irradiated with two 10 W LED (UV, Epistar Co, Taipei, China) bulbs. The photon flux was 39.8 μeinstein per min. Experiments were conducted in triplicates and the mean and standard deviation values were reported. The MicroCuZn was reused and ultrasonically washed with acetone, ethanol, and DI water for 10 min, and then dried at 90 °C for 6 h. Experiments were carried out at room temperature (21 ± 1 °C).
2.5. Hydroxyl radical generation
The catalytic and photocatalytic performance were studied with two ˙OH scavengers, i.e., pNDA and TA. pNDA has been reported previously as an effective ˙OH scavenger;38,39 however, a recent study suggested that pNDA can be susceptible to reductive bleaching.40 Thus TA was used to confirm and validate effective ˙OH generation.
In aqueous solution and at neutral pH, pNDA gives an intense yellow color owing to conjugated double bonds in the molecule with a strong absorption band at 440 nm.41 When pNDA reacts with ˙OH,39 it becomes colorless at 440 nm;42 this change is commonly named as pNDA bleaching, which was recorded with a Carry 8454 UV-visible spectrophotometer (Agilent Technologies, Santa Clara, CA, USA). The ˙OH generation in the batch reactor, based on pNDA bleaching, is described with eqn (1).
|
 | (1) |
where, [
pNDA] represents concentration of
pNDA (M), [˙OH]
ss represents steady-state concentration of ˙OH (M), and
kobs is the kinetic rate constant.
Photo-hydroxylation reaction of TA produces 2-THA, a fluorescent substance analyzed with an LS-5 fluorescence spectrophotometer (PerkinElmer, Oak Brook, IL, USA) by excitation at λ = 315 nm and fluorescence detection at λ = 425 nm.43 With a steady-state assumption, ˙OH production in the batch reactor can be calculated with eqn (2).44
|
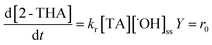 | (2) |
where [2-THA] represents 2-THA molar concentration (M),
t is time (min),
r0 is a zero-order rate of 2-THA production (M min
−1),
kr is the reaction rate constant (4.4 × 10
9 M
−1 s
−1), [˙OH]
ss is the steady-state molar concentration (M), and
Y is yield as was described earlier.
44 It is to be noted that
eqn (1) and
(2) though capture the kinetics of ˙OH radical generation, these are utilized to determine reaction rate constants only for the cases when the data collected fit pseudo-first kinetics.
2.6. Analytical methods
E3 and THC concentrations were analyzed with high-performance liquid chromatography (HPLC). HPLC spectra were obtained with a Nexera LC-2040C (Shimadzu Corporation, Kyoto, Japan), equipped with an autosampler, UV-visible detector Shimadzu RF-20A, and a Phenomenex Kinetex column (100 × 4.60 mm; 2.6 μm) kept at 55 °C. The detection of E3 and THC was conducted at 197 nm and 190 nm, respectively. The system used an isocratic mobile phase acetonitrile–water (30
:
70, v/v) at a flow rate of 0.75 mL min−1 and an injection volume of 10 μL for E3 detection, and acetonitrile–water (68
:
32, v/v) at a flow rate of 1.0 mL min−1 for THC. The detection limit of E3 and THC was 0.1 mg L−1 and 0.01 mg L−1, respectively. Dissolved metal concentrations (i.e., for Cu and Zn ions) were quantified with inductively coupled plasma optical emission spectroscopy (ICP-OES) using a Varian 710 ES (Palo Alto, CA) in an argon plasma flame at 7000 K, supported by the ICP Expert II™ software for simultaneous measurement and analysis of all wavelengths of interest. Prior to analysis, samples were diluted by a factor of 10 in 2% (v/v) nitric acid. The detection limit of ICP-OES was 0.01 mg L−1 for both Cu and Zn.
3. Results and discussion
3.1. MicroCuZn alloy characterization
The MicroCuZn (Fig. 1a) is a mixture of different sized grains with irregular rounded particles, as shown in representative TEM images (Fig. 1b). MicroCuZn has a density of 2.91 g cm−3. Maximum and minimum Feret's diameter (Fig. S4†), i.e., the longest and shortest distance between any two points along the particle boundary, are determined to be 0.826 ± 0.144 mm (range 0.44 to 1.11 mm) and 0.496 ± 0.09 mm (range 0.31 to 0.69 mm), respectively. Average projected area (Fig. S4d†) is estimated to be 0.261 ± 0.068 mm2 (range 0.11 to 0.41 mm2) with circularity of 0.482 ± 0.126 (range 0.21 to 0.77); which indicate elongated materials (Fig. S4†).
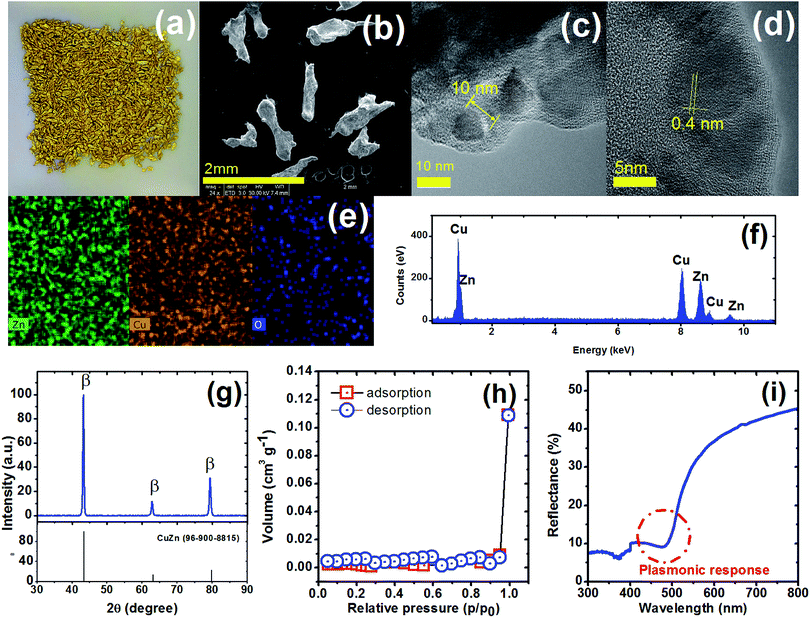 |
| Fig. 1 Optical and morphological characterization of MicroCuZn. (a) Photograph showing MicroCuZn particles; (b) SEM image showing size and shape of MicroCuZn; (c and d) TEM and high-resolution TEM micrographs showing MicroCuZn structure and lattice fringes; (e) SEM/EDS mapping of elemental distribution; (f) elemental composition shown with SEM-EDS spectrum; (g) matching β-phase with experimental data of XRD; (h) nitrogen gas adsorption/desorption hysteresis curves employed in specific BET surface area analysis; and (i) diffuse reflectance spectrum showing plasmonic response in the bulk material near 475 nm. | |
High-resolution TEM micrographs show 10 nm wide crystals (Fig. 1c), having defined lattice fringes with 0.4 nm spacing (Fig. 1d). The elemental distribution appears to be homogeneous (Fig. 1e) with 47.2 wt% copper, 44.4 wt% zinc. Other detected elements form the EDS analysis are shown in Table S1.† According to the Cu and Zn content, the MicroCuZn composition corresponds to the α + β phase as observed in the phase diagram; the analysis is based on Kaprara et al.12 method (Fig. S5†). XRD analysis (Fig. 1g) further confirms the dominance of the β-phase with defined peaks at 43.36° and 79.47°, when compared with the Crystallography Open Database (card 9008814).31 Kaprara et al.12 suggested (1 0 0) crystal planes in MicroCuZn, which are a result of the crystallization process.
The specific BET surface area (Fig. 1h) is determined to be 0.003 m2 g−1. Based on the International Union of Pure and Applied Chemistry (IUPAC) classification,45 the isotherm in Fig. 1h indicates a reversible type II behavior, obtained with a non-porous or macroporous adsorbent. The surface homogeneity can be confirmed by comparing the specific surface area and the specific external surface area (eqn (3)).
|
 | (3) |
where,
γ is specific external surface area (m
2 g
−1),
Se is particle surface area (m
2),
mp is particle weight (g
−1),
a is average projected area (m
2),
m is average particle weight (g
−1). Considering that the average particle has Ferret diameter (
a) and that its average weight is 0.009 ± 0.003 mg,
γ is determined to be 0.029 ± 0.0015 m
2 g
−1, which is in the same order of magnitude as the one obtained from the specific surface area analysis; these results confirm that the catalyst particles are likely non-porous, thus the catalytic or adsorption processes will likely take place on the surface of the catalyst.
The diffuse reflectance spectrum (Fig. 1i) shows a sharp decrease and then an increase near 475 nm (2.6 eV), which suggest a plasmonic response in MicroCuZn. The plasmonic response in the bulk β-phase is suggesting that the optical property is due to the inter-band transition from Fermi level (Ef) to the vacant conduction sp states.46,47
3.2. Removal of E3 and THC from water
Fig. 2a shows that E3 removal in dark is negligible, while MicroCuZn can remove only <4% of E3 after 48 h. The low E3 (with log
Kow = 2.45) removal is likely due to adsorptive partitioning, which agrees well with low sorption propensity showed on activated carbon.48 E3 shows a slow decrease in the presence of H2O2, likely due to the generation of ˙OH (eqn (4)) in the presence of this oxidant.49 |
H2O2 → OH− + ˙OH → 1/2O2 + 2H2O
| (4) |
˙OH is generated in the presence of H2O2 at pH 7.0 and above. It is to be noted that all experiments for contaminant removal have been conducted in pH 7.0, while the terephthalic acid (TA) measurements were conducted at pH 9.0 (to ensure solubility of TA). TA is a substance that reacts with ˙OH radical and the responses measured for 2-THA production indicate ˙OH radical production. Data presented in Fig. S6† shows that, MicroCuZn under UV irradiation produces ˙OH radicals. However, uncatalyzed decomposition of H2O2 can take place because of temperature, pH, surface effects, impurities, and photo-radiation.50
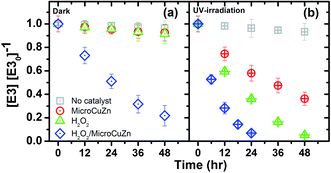 |
| Fig. 2 E3 degradation (10 μM, pH 7) with MicroCuZn (10 g L−1) at 20 °C and with H2O2 (0.1 M); (a) in dark and (b) under UV-irradiation (9.9 μeinstein per min). | |
E3 decay increases when MicroCuZn and H2O2 react in concert and reaches 84.3% removal in 48 h. Such decay is likely caused by corrosion of the catalyst (eqn (5))20 that produces ˙OH radicals via catalytic H2O2 disproportionation near the catalyst–water interface and by Fenton-like reaction of Cu in the bulk water (eqn (6) and (7)).35,51 Zhou et al. suggested that Cu+ could be released following the reaction pathway shown in eqn (6).51
|
Brass (Cu + Zn) → xCu2+ + yZn2+ + 2(x + y)e−
| (5) |
|
Cu0(s) + 1/2H2O2 → Cu+ + OH−
| (6) |
|
Cu+ + H2O2 → Cu2+ + ˙OH + OH−
| (7) |
Fig. 2b shows E3 removal under UV irradiation. E3 degradation by photolysis alone (i.e., in the absence of any catalyst or oxidant) is 3.1% after 48 h; this result is significantly lower than the previously reported photo-removal of E3 that used a backlight fluorescent lamp and a germicidal lamp.52 The decreased photo-removal can be attributed to the intensity and the emission peak of the lamp, which did not overlap with the molar absorption of E3 in this study (Fig. S7†).
E3 considerably decreases in the UV/MicroCuZn system, with 62.7% removal after 48 h, which is significantly higher than the extent of removal observed with the catalyst in the dark (Fig. 2a). The rate of decay followed pseudo-first-order kinetics with a rate constant of 0.335 × 10−3 min−1 (r2 = 0.996). The removal was likely because of the direct ˙OH attack of E3 and h+ oxidation. This assumption is based on that photocorrosion of brass that can produce ROS, as shown by others.23 Dissolved O2 and H2O2 causes brass corrosion when irradiated with UV. Cu/Zn ratio in brass is a critical factor in determining the susceptibility of dezincification; e.g., brass with zinc content of ∼15% is generally considered resistant to dezincification.53 Lin and Frankel studied atmospheric corrosion of copper and found that UV promotes Cu2O formation.54 Zhang et al. verified the presence of hydrozincite, simonkolleite, and CuO, Cu2O, ZnO in the corrosion products of brass with 40% Cu content.55 Sun et al. demonstrated that UV irradiation promoted atmospheric corrosion of brass alloy and detected formation of Cu(OH)2, CuO, and Cu2O in dark, and CuO, Cu2O, ZnO, Zn5(OH)8Cl2·H2O, Zn5(CO3)2(OH)6 when UV-irradiated.22 Ibrahim et al. found copper corrosion in the presence and absence of a low gamma irradiation and cited formation of Cu2O.23 Zhdan and Castle demonstrated CuO growth on the surface of brass.56 Even though, E3 may be prone to degradation and thereby produce less estrogenic compounds57 and to mineralization because of ˙OH attacks,58 further studies are necessary to unravel the mechanisms underlying such photo-removal.
The E3 concentration substantially decreases in UV/H2O2 system; i.e., by 94.7% after 48 h, which is significantly greater than H2O2 oxidation in the dark. Such a decrease may be attributed to the UV-mediated (i.e., emitting between 363 nm and 415 nm) photolysis of H2O2, as shown in eqn (8).59 It has been reported that H2O2 photolysis is effective at wavelength lower than 380 nm.60 The E3 degradation by H2O2 with low- and medium-pressure mercury lamp irradiation has been well-documented.61
The systematic increase in the rate of E3 degradation (Fig. 2) is an illustration of the possible synergy between the irradiating UV energy, oxidizing agent H2O2 and the catalyst MicroCuZn. The UV/H2O2/MicroCuZn system accomplishes the fastest E3 degradation, following pseudo-first-order kinetics with a decay rate constant of 1.853 × 10−3 min−1 (r2 = 0.999); this value is three times greater than the H2O2/MicroCuZn system in the dark. The extent of removal in this case is the greatest among all conditions studied. The absence of the catalyst or that of H2O2 has shown to decrease the E3 degradation rate.
The electrical energy per order (EEO) index is used to assess efficiency in terms of electrical energy consumption for degrading contaminants.62 EEO index for UV/H2O2/MicroCuZn is obtained as 1.03 × 103 kW h m−3; which is the lowest of all the values, indicating that this condition is the most energy efficient. The EEO value estimated here is consistent with the reported advanced oxidation energy efficiency for UV/H2O2/MicroCuZn, presented in Miklos et al.63 This material thus can become a practical, effective, and energy-efficient option for oxidative E3 removal from water.
Fig. 3 shows that THC concentration decreases over time in both dark and UV irradiated conditions. In dark, the glass reactor and MicroCuZn exhibit significant adsorption for THC, with 81.2% and 91% of removal over 300 min, respectively. It seems THC's hydrophobicity (log
Kow > 5) and electrostatic attraction towards MicroCuZn and silicates (within the glass) are likely responsible for such adsorption.27,64,65 The THC results indicate that the removal is caused by a combination of adsorption and possible photoreaction. After adsorption, THC may be oxidized via ˙OH radicals. The complete mineralization of THC towards CO2 and H2O may take place by photocatalytic and heterogeneous photo-Fenton treatment.65,66 It is to be noted that contribution of photoreaction alone in the removal of this hydrophobic compound, cannot be tweezed out.
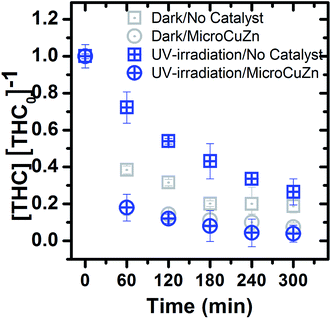 |
| Fig. 3 THC concentration history (1 μM initial concentration at pH 7) to elucidate MicroCuZn (10 g L−1) catalysis effects. Experiment were conducted at 20 °C and UV-irradiation was 9.9 μeinstein per min. | |
THC removal in the dark/no catalyst and UV/no catalyst conditions are determined to be 81.2% and 74%, respectively. It can thus be concluded that THC photolysis is not the dominant removal process upon 300 min of UV irradiation. Not only that the intensity and emission peaks of the lamps do not overlap with THC molar absorption in the UV-visible region (Fig. S7†), as was also the case for E3, extent of THC adsorption on the glass container might have been influenced during UV irradiation. That is, localized heating of the glass surfaces or radicals generated (in the solution) during the irradiation process could have contributed to partial desorption of THC from glass, thus reduced the overall removal when compared to the catalyst-free case in the dark. Special reactors (e.g., silanized glass) to suppress THC adsorption onto glass can be utilized for untangling photo-catalytic removal from that via adsorption.
3.3. Hydroxyl radical production
We hypothesized in Section 3.2 that E3 removal is primary caused by photoreaction with ROS, in particular from ˙OH. pNDA is a ˙OH probe, that is useful for measuring the photocatalytic performance in water treatment with the following advantages: (i) it has selective 1
:
1 reactivity with ˙OH,67–70 (ii) it has a high reaction rate with ˙OH, which is in the order of 1010 M−1 s−1 (ref. 70 and 71) and (iii) it does not react with singlet oxygen (1O2), superoxide anions (O2˙−) or other peroxy compounds.41 Generation of ˙OH is measured with pNDA bleaching under identical experimental conditions.
In general, pNDA bleaching (see Fig. 4) in the dark and under UV irradiation follows similar trend as observed in Fig. 2. In the dark (Fig. 4a), pNDA blank stays unchanged, while it slightly decreases (about 10%) owing to adsorption onto MicroCuZn. Similar observation has been reported earlier for aniline adsorption onto brass.72 A decrease of 15% is detected due to ˙OH generation in the presence of H2O2 (eqn (4)). pNDA bleaching was also reported earlier at an elevated temperature (50 °C).73
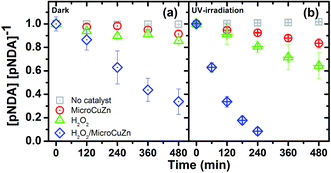 |
| Fig. 4 pNDA bleaching (10 μM, pH 7) with MicroCuZn (10 g L−1) at 20 °C and with needed H2O2 (0.1 M); (a) in dark and (b) under UV irradiation (9.9 μeinstein per min). Note that pH values have been measured before and after the experiments and are reported in Table S2.† | |
The concentration of pNDA decreases rapidly due to ˙OH production in the presence of H2O2, which then catalyzes MicroCuZn. ˙OH production under this condition followed a catalytic reaction on the MicroCuZn surface in the dark and Fenton-like reaction at neutral pH (eqn (6) and (7)). Excess H2O2 in the solution may accelerate MicroCuZn corrosion and thereby cause leaching of Cu and Zn ions into the bulk solution.74 Pham et al.75 confirmed that Fenton-like reaction can be initiated by Cu ions at neutral pH and Hobbs and Abbot73 reported pNDA bleaching by ionic Cu at comparable magnitude as measured in this study. H2O2 can reduce Cu2+, generating O2˙− and Cu+, which then can catalyze H2O2 to produce ˙OH and Cu2+ (eqn (6) and (7)). Conversely, it has been reported that Zn2+ does not significantly influence the formation of ˙OH in a Fenton-like reaction.76
H2O2 can be decomposed50 and catalyzed75,77,78 by Cu2+ to produce ˙OH, causing Cu+ and Cu2+ dissolution. However, at pH 7 (i.e., the condition used in the contaminant degradation experiments), Cu+ and Cu2+ ions form stable CuOH and Cu(OH)2 species, respectively (eqn (9)–(11)), which can subsequently redeposit and form Cu2O.35
|
Cu2+ + OH− → Cu(OH)2
| (10) |
pNDA is reduced at a moderate rate in the UV/MicroCuZn system because of ˙OH attacks. A recent study has questioned the selectivity of pNDA to ˙OH, suggesting that pNDA bleaching could be due to a reduction reaction.40 To probe this question, TA is used to assess MicroCuZn photocatalytic activity (in UV/MicroCuZn system). 2-THA generation shown in Fig. S7† confirms that UV/MicroCuZn can produce ˙OH. The evidence of ˙OH generation is detected from not only pNDA bleaching but also from 2-THA fluorescence in MicroCuZn/UV system, which supports the assumption that MicroCuZn photocatalytic activity is caused by the ˙OH attack.
It is well known that when the MicroCuZn alloy is used in a catalytic process, oxidation of Cu and Zn takes place, which leads to formation of CuO, Cu2O, ZnO.23,79 Furthermore, when ZnO and CuxO combine, a (p–n) heterojunction is formed,80,81 which is effective in enhanced utilization of photoenergy and modulation of e−/h+ recombination. Though it is challenging to pin point electron excitation and transfer within a complex alloy (and subsequent oxidized form of the alloy) through measurements, reasonable analysis can be made and conclusions can be drawn from the energetic band positioning of the oxidized forms of Cu and Zn.
Typically, upon photo-irradiation, promotion of electrons to the conduction band (CB), leaves holes behind at the valence band (VB). The excited or hot-electrons (e−) are known to transfer to the surrounding dissolved oxygen molecules and produce ˙O2−, while holes (h+) are known to transfer to −OH and produce ˙OH. O2− is also known to form ˙OH through a cascading reaction sequence82 while recombination of e−/h+ pair can compromise the radical production process. In the complex mixture of CuxO/ZnO, the relative positioning of VB for Cu2O makes electron excitation to the CB of this oxide phase, likely (Fig. 5a). The UV irradiation at 363 nm to 415 nm wavelength provides necessary photo-energy for hot e− production, not only from the Cu2O, but also from the other two oxide phases (i.e., CuO and ZnO as shown in Fig. 5b). Hot e− generated within the Cu2O phase can migrate to the CB of either ZnO and CuO, while the photogenerated h+ from these phases can migrate toward the VB of the Cu2O phase.81 Such e− and h+ transfer within the complex oxide phases of the alloy likely modulates (i.e., delays or prevents) recombination of the photogenerated e−/h+ pair.81
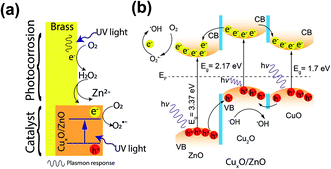 |
| Fig. 5 Photocatalytic mechanism on MicroCuZn surface: (a) photocorrosion initiation and formation of patches; and (b) electron–hole transfer mechanism with hydroxyl radical generation where CB is conduction band, VB is valence band, and Ef is Fermi level.81 | |
In order to corroborate the formation of a complex oxide film (ZnO/CuxO), XPS analyses were conducted. Fig. 6 shows the overall and deconvoluted XPS spectra obtained for samples in the dark and those exposed to UV irradiation for 12 h. The overall spectrum (Fig. 6a) shows O, C, Zn, and Cu characteristic peaks, where the high intensity Zn 2p3/2 peaks have likely occurred due to the high zinc content within MicroCuZn. Specific XPS spectra of Cu 2p3/2 region (Fig. 6b and c) show a significant decrease in the Cu(OH)2 peak and an increase in the Cu2O peak. Ibrahim et al.23 suggested that Cu corrosion can lead to CuO–Cu2O formation. Zn 2p3/2 region deconvolution (Fig. 6d and e) shows that the UV irradiated case has an increase in metallic zinc content (Zn(0)). Such increase may be due to the Zn atoms rearrangement, as suggested by Zhou.83
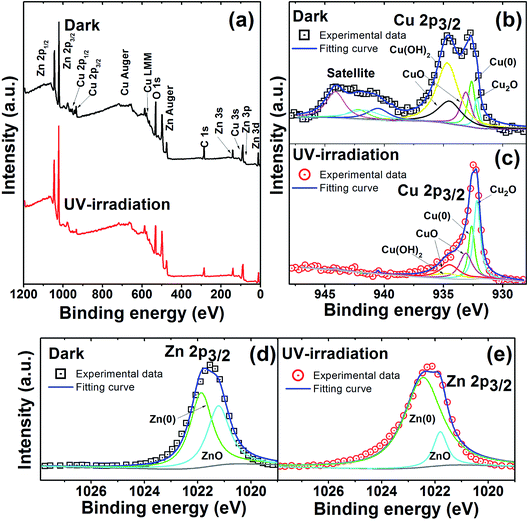 |
| Fig. 6 (a) Overall XPS spectrum with identified characteristic peaks for MicroCuZn in dark and when UV irradiated over 12 h in deionized water. Deconvoluted XPS spectra for Cu 2p3/2 region in (b) dark and when (c) UV-irradiated. Deconvoluted XPS spectra for Zn 2p3/2 region in (d) dark and when (e) UV-irradiated. | |
The complex oxide film formation is guided by the reactions under non-irradiated and irradiated conditions. In non-irradiated conditions, selective leaching of Zn occurs to form Cu-rich areas.84 Such dezincification of the surfaces has been intensively investigated elsewhere,56,85 however, a consensus on formation mechanism has not been reached.83 It can be hypothesized that preferential dissolution of Zn from the Cu–Zn lattice will likely take place due to zinc's equilibrium potential being more negative, when compared to Cu.83 It is also important to note that upon dezincification formation of zinc hydroxide or oxides may take place.86 Whittle et al. suggested that ZnO can be formed due to reaction with Cu2O that can generate patchy mixed oxide phases of CuxO/ZnO on the MicroCuZn surfaces.87 Furthermore, Cu-rich areas can be oxidized to Cu2O88 and subsequently to CuO,23 eventually leading to growth of CuxO patches. Moreover, H2O2 can be decomposed50 and catalyzed75,77,78 by Cu2+ to produce ˙OH, causing Cu+ and Cu2+ dissolution and redeposition. However at pH 7, Cu+ and Cu2+ forms stable oxide forms CuOH and Cu(OH)2, respectively, which can also redeposit and form Cu2O.35 UV irradiation may increase the dezincification of brass89 and consequently may increase the Cu-rich areas.
This also indicates that the photocatalytic activity of the catalyst may have been caused by the increase of the number of semiconductor-like areas or patches on the surface. Recently, it has been reported Zn/CuxO is an effective photocatalyst for water treatment90,91 or for water splitting.92
3.4. Reusability of MicroCuZn photocatalytic activity
Reusability and stability of MicroCuZn is shown in Fig. 7, where the left panels show pNDA signal strength and dissolved Cu and Zn concentration under a prolonged irradiation condition (4 h flow through operation at 1 mL min−1); while the panels on the right show the same for an alternating irradiating and dark condition cycling. Fig. 7a shows that pNDA bleaching remains unchanged during the dark phase, followed by a strong bleaching phase when the catalyst is activated under UV irradiation. The alternating dark-illuminated cyclic exposure of the catalyst show effectiveness of pNDA bleaching at the same extent, even after three cycles of use (Fig. 7c). Fig. 7b and d demonstrate that the MicroCuZn catalysts are stable and do not dissolve ionic Cu (<0.01 mg L−1) or Zn (<0.1 mg L−1) significantly.
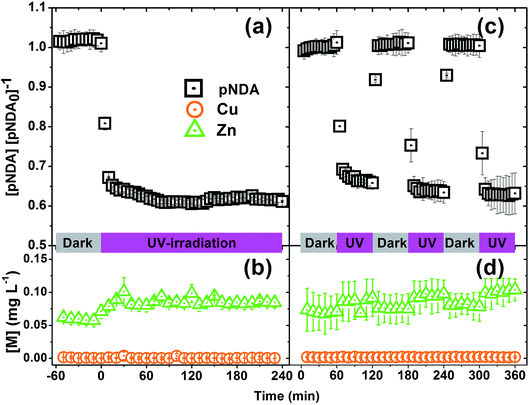 |
| Fig. 7 Photocatalytic activity of MicroCuZn in a continuous packed photocatalytic reactor (1 mL min−1) for the pNDA bleaching (10 μM, pH 7). Stability shown via (a) photobleaching history and (b) low propensity of metal leaching. Performance under cyclic in dark and illuminated conditions show with (c) cyclic recovery of photobleaching ability and (d) low propensity of metal leaching. | |
4. Conclusions
Characterization of MicroCuZn confirmed that commercially available KDF® 55 was a β-brass with homogenous elemental distribution and plasmonic response near 475 nm. H2O2 increased its catalytic activity. The material was shown to degrade two structurally relevant model ECs with phenolic moieties and high octanol–water partitioning coefficients (i.e., both highly hydrophobic). The photodegradation and removal from water were systematically evaluated to unravel the underlying mechanisms. Photocatalytic mechanism of MicroCuZn was likely initiated in CuZn alloy crystalline arrays by the plasmonic response, which introduced hot electrons that can be transferred to the neighboring dissolved oxygen molecules to produce ˙O2−. These radicals caused brass corrosion to initiate a cascading reaction sequence for ˙OH generation. When MicroCuZn is stimulated with UV radiation, a semiconductor oxide film grew on the MicroCuZn surfaces. We suggest that a mixture of ZnO, CuO, and Cu2O may be responsible for MicroCuZn photocatalytic activity for E3 removal. On the other hand, adsorption effect was observed to be a critical process for THC removal from water, while photocatalysis mildly enhance the removal efficacy.
Micron-scale brass can be effectively applied for oxidative removal of ECs. These micron-scale alloys can be considered as promising photo-oxidative alternatives considering their low-cost, earth-abundancy, stability during reaction, and potential reusability. In addition, these catalysts are considered non-toxic because of their low-leaching potential. Further research is necessary to evaluate the efficacy of these materials in a more complex aquatic background (e.g., in the presence of natural organic matter, turbidity, ionic strength, etc.) as well as to test their effectiveness in environmentally pertinent levels of ECs (i.e., at ng L−1) in continuous reactors. Such studies will facilitate transferability of these materials from laboratory to engineering practice. Mechanistic understanding of the pollutant degradation pathways that are elucidated here will enable strategic micro-brass synthesis for water treatment purposes and facilitate future engineering solutions (e.g., reactor design and material recovery) for municipal level or point-of-use water treatment systems and ensure safe and sustainable deployment of this millennia-old alloy as an effective catalyst.
Conflicts of interest
There are no conflicts to declare.
Acknowledgements
This research was supported by the University of Texas System and the Consejo Nacional de Ciencia y Tecnología (CONACYT) through the ConTex Postdoctoral Fellowship Program. The opinions expressed are those of the authors and do not represent the views of these funding agencies. Authors thank the University of Texas at Austin for granting financial resources, access to facilities, and training to conduct this research. Thanks are extended to Olivia Franco for his technical assistance to obtain diffuse reflectance data from the National Polytechnic Institute of Mexico (IPN), to Viridiana Maturano for obtaining BET isotherms from the National Autonomous University of Mexico (UNAM).
References
- B. Petrie, R. Barden and B. Kasprzyk-Hordern, A review on emerging contaminants in wastewaters and the environment: current knowledge, understudied areas and recommendations for future monitoring, Water Res., 2015, 72, 3–27 CrossRef CAS.
- O. M. Rodriguez-Narvaez, J. M. Peralta-Hernandez, A. Goonetilleke and E. R. Bandala, Treatment technologies for emerging contaminants in water: a review, Chem. Eng. J., 2017, 323, 361–380 CrossRef CAS.
- G. Crini and E. Lichtfouse, Advantages and disadvantages of techniques used for wastewater treatment, Environ. Chem. Lett., 2019, 17, 145–155 CrossRef CAS.
- G. Grass, C. Rensing and M. Solioz, Metallic copper as an antimicrobial surface, Appl. Environ. Microbiol., 2011, 77, 1541–1547 CrossRef CAS.
- G. Bottari, A. J. Kumalaputri, K. K. Krawczyk, B. L. Feringa, H. J. Heeres and K. Barta, Copper-Zinc Alloy Nanopowder: A Robust Precious-Metal-Free Catalyst for the Conversion of 5-Hydroxymethylfurfural, ChemSusChem, 2015, 8, 1323–1327 CrossRef CAS.
- Y. Feng, Z. Li, H. Liu, C. Dong, J. Wang, S. A. Kulinich and X. Du, Laser-Prepared CuZn Alloy Catalyst for Selective Electrochemical Reduction of CO2 to Ethylene, Langmuir, 2018, 34, 13544–13549 CrossRef CAS.
- A. Chafidz, Megawati, C. R. Widyastuti, V. Augustia, K. Nisa and Ratnaningrum, Application of copper-zinc metal as a catalytic converter in the motorcycle muffler to reduce the exhaust emissions, IOP Conf. Ser. Earth Environ. Sci., 2018, 167, 1–8 Search PubMed.
- F. Fu, Z. Cheng and J. Lu, Synthesis and use of bimetals and bimetal oxides in contaminants removal from water: a review, RSC Adv., 2015, 5, 85395–85409 RSC.
- A. Zaleska-Medynska, M. Marchelek, M. Diak and E. Grabowska, Noble metal-based bimetallic nanoparticles: the effect of the structure on the optical, catalytic and photocatalytic properties, Adv. Colloid Interface Sci., 2016, 229, 80–107 CrossRef CAS.
- J.-H. Richard and H. Biester, Mercury removal from contaminated groundwater: performance and limitations of amalgamation through brass shavings, Water Res., 2016, 99, 272–280 CrossRef CAS.
- H. S. Majdi, M. S. Jaafar and A. M. Abed, Using KDF material to improve the performance of multi-layers filters in the reduction of chemical and biological pollutants in surface water treatment, S. Afr. J. Chem. Eng., 2019, 28, 39–45 Search PubMed.
- E. Kaprara, P. Seridou, V. Tsiamili, M. Mitrakas, G. Vourlias, I. Tsiaoussis, G. Kaimakamis, E. Pavlidou, N. Andritsos and K. Simeonidis, Cu-Zn powders as potential Cr(VI) adsorbents for drinking water, J. Hazard. Mater., 2013, 262, 606–613 CrossRef CAS.
- A. Sites and L. Oberholtzer, Mercury point-of-entry treatment study, unpublished report, NJ Department of Environmental Protection and Energy, Trenton, NJ, 1992, p. 43 Search PubMed.
- D. P. Fourness, Evaluation of Decentralized Alternatives for Separate Treatment and Supply of Indoor Water: Fort Collins Case Study, MSc thesis, Color. State Univ., 2015, 154.
- N. A. Hanks, Silver Nanoparticle and Silver Ion Water Contamination: Assessment of Phytoremediation and Point-of-use Filtration Media, PhD thesis, University of Cincinnati, 2015, p. 128.
- A. Carrière, M. Brouillon, S. Sauvé, M. F. Bouchard and B. Barbeau, Performance of point-of-use devices to remove manganese from drinking water, J. Environ. Sci. Health, Part A: Toxic/Hazard. Subst. Environ. Eng., 2011, 46, 601–607 CrossRef.
- K. Jing, X. Guo, Y. Jiang, Q. Wu, S. Wang, X. Shi, N. Zhou and Z. Zhu, Removal of Antimony(III) from Aqueous Solutions Using Copper-Zinc Alloy Filter Media, Open Journal of Water Pollution and Treatment, 2014, 1–10 CrossRef.
- A. Catennaci, Heavy metal removal from water: characterization and applicability of unconventional media, PhD thesis, Politecnico di Milano, 2014, p. 129.
- Q. Wang, Y. Zhang, H. Wang and L. Ma, A pathway of free radical generation via copper corrosion and its application to oxygen and ozone activation for the oxidative destruction of organic pollutants, Res. Chem. Intermed., 2018, 44, 7391–7410 CrossRef CAS.
- F. M. Al Kharafi, I. M. Ghayad and R. M. Abdullah, Effect of hydrogen peroxide on the dezincification of brass in acidified sodium sulfate solution under free corrosion conditions, J. Mater. Environ. Sci., 2010, 1, 58–69 CAS.
- T. D. Burleigh, C. Ruhe and J. Forsyth, Photo-Corrosion of Different Metals during Long-Term Exposure to Ultraviolet Light, Corrosion, 2003, 59, 6 CrossRef.
- X. Sun, Initial NaCl-induced atmospheric corrosion of a dual-phase Cu60-40Zn alloy - Effect of UV illumination, Int. J. Electrochem. Sci., 2018, 13, 8150–8169 CrossRef CAS.
- B. Ibrahim, D. Zagidulin, M. Behazin, S. Ramamurthy, J. C. Wren and D. W. Shoesmith, The corrosion of copper in irradiated and unirradiated humid air, Corros. Sci., 2018, 141, 53–62 CrossRef CAS.
- Å. Björkbacka, C. M. Johnson, C. Leygraf and M. Jonsson, Radiation Induced Corrosion of Copper in Humid Air and Argon Atmospheres, J. Electrochem. Soc., 2017, 164, C201–C206 CrossRef.
- S. Deng, H. Lu and D. Y. Li, Influence of UV light irradiation on the corrosion behavior of electrodeposited Ni and Cu nanocrystalline foils, Sci. Rep., 2020, 10, 3049 CrossRef CAS.
- O. G. Apul, L. S. Rowles, A. Khalid, T. Karanfil, S. D. Richardson and N. B. Saleh, Transformation potential of cannabinoids during their passage through engineered water treatment systems: a perspective, Environ. Int., 2020, 137, 105586 CrossRef CAS.
- A. Khalid, L. S. Rowles, M. Ateia, M. Xiao, I. Ramirez-Sanchez, D. Bello, T. Karanfil, N. B. Saleh and O. G. Apul, Mesoporous activated carbon shows superior adsorption affinity for 11-nor-9-carboxy-Δ9-tetrahydrocannabinol in water, npj Clean Water, 2020, 3, 2 CrossRef CAS.
- N. B. Saleh, O. Apul and T. Karanfil, The Genesis of a Critical Environmental Concern: Cannabinoids in Our Water Systems, Environ. Sci. Technol., 2019, 53, 1746–1747 CrossRef CAS.
- Z. Liu, G. Lu, H. Yin and Z. Dang, Do we underestimate the concentration of estriol in raw municipal wastewater?, Environ. Sci. Pollut. Res., 2015, 22, 4753–4758 CrossRef CAS.
- M. Adeel, X. Song, Y. Wang, D. Francis and Y. Yang, Environmental impact of estrogens on human, animal and plant life: a critical review, Environ. Int., 2017, 99, 107–119 CrossRef CAS.
- A. Altomare, N. Corriero, C. Cuocci, A. Falcicchio, A. Moliterni and R. Rizzi, QUALX2.0: a qualitative phase analysis software using the freely available database POW_COD, J. Appl. Crystallogr., 2015, 48, 598–603 CrossRef CAS.
- M. C. Biesinger, Advanced analysis of copper X-ray photoelectron spectra, Surf. Interface Anal., 2017, 49, 1325–1334 CrossRef CAS.
- M. C. Biesinger, L. W. M. Lau, A. R. Gerson and R. S. C. Smart, Resolving surface chemical states in XPS analysis of first row transition metals, oxides and hydroxides: Sc, Ti, V, Cu and Zn, Appl. Surf. Sci., 2010, 257, 887–898 CrossRef CAS.
- K. Ishibashi, A. Fujishima, T. Watanabe and K. Hashimoto, Detection of active oxidative species in TiO2 photocatalysis using the fluorescence technique, Electrochem. Commun., 2000, 2, 207–210 CrossRef CAS.
- P. V. F. de Sousa, A. F. de Oliveira, A. A. da Silva and R. P. Lopes, Environmental remediation processes by zero valence copper: reaction mechanisms, Environ. Sci. Pollut. Res., 2019, 26, 14883–14903 CrossRef CAS.
- R. D. Lorenz, A simple webcam spectrograph, Am. J. Phys., 2014, 82, 169–173 CrossRef CAS.
- S. L. Murov, I. Carmichael and G. L. Hug, Handbook of Photochemistry, CRC Press, New York, NY, 2nd edn, 1993 Search PubMed.
- N. Nordin, L.-N. Ho, S.-A. Ong, A. H. Ibrahim, A. L. Abdul Rani, S.-L. Lee and Y.-P. Ong, Hydroxyl radical formation in the hybrid system of photocatalytic fuel cell and peroxi-coagulation process affected by iron plate and UV light, Chemosphere, 2020, 244, 125459 CrossRef CAS.
- I. Kraljic and C. N. Trumbore, p-Nitrosodimethylaniline as an OH radical scavenger in radiation chemistry, J. Am. Chem. Soc., 1965, 87, 2547–2550 CrossRef CAS.
- S. Mortazavian, E. R. Bandala, J.-H. Bae, D. Chun and J. Moon, Assessment of p-nitroso dimethylaniline (pNDA) suitability as a hydroxyl radical probe: investigating bleaching mechanism using immobilized zero-valent iron nanoparticles, Chem. Eng. J., 2020, 385, 123748 CrossRef CAS.
- M. E. Simonsen, J. Muff, L. R. Bennedsen, K. P. Kowalski and E. G. Søgaard, Photocatalytic bleaching of p-nitrosodimethylaniline and a comparison to the performance of other AOP technologies, J. Photochem. Photobiol., A, 2010, 216, 244–249 CrossRef CAS.
- J. Muff, L. R. Bennedsen and E. G. Søgaard, Study of electrochemical bleaching of p-nitrosodimethylaniline and its role as hydroxyl radical probe compound, J. Appl. Electrochem., 2011, 41, 599–607 CrossRef CAS.
- L. T. T. Tuyen, D. A. Quang, T. T. Tam Toan, T. Q. Tung, T. T. Hoa, T. X. Mau and D. Q. Khieu, Synthesis of CeO2/TiO2 nanotubes and heterogeneous photocatalytic degradation of methylene blue, J. Environ. Chem. Eng., 2018, 6, 5999–6011 CrossRef CAS.
- S. E. Page, W. A. Arnold and K. McNeill, Terephthalate as a probe for photochemically generated hydroxyl radical, J. Environ. Monit., 2010, 12, 1658–1665 RSC.
- K. S. W. Sing, Reporting physisorption data for gas/solid systems with special reference to the determination of surface area and porosity (Recommendations 1984), Pure Appl. Chem., 1985, 57, 603 CAS.
- V. J. Keast, J. Ewald, K. S. B. De Silva, M. B. Cortie, B. Monnier, D. Cuskelly and E. H. Kisi, Optical properties and electronic structure of the Cu–Zn brasses, J. Alloys Compd., 2015, 647, 129–135 CrossRef CAS.
- I. I. Sasovskaya and V. P. Korabel, Optical properties of α- and β-CuZn brasses in the region of quantum absorption, Phys. Status Solidi, 1986, 134, 621–630 CrossRef CAS.
- F. Ogata, H. Tominaga, H. Yabutani and N. Kawasaki, Removal of Estrogens from Water Using Activated Carbon and Ozone, J. Oleo Sci., 2011, 60, 609–611 CrossRef CAS.
- A. Zhang and Y. Li, Removal of phenolic endocrine disrupting compounds from waste activated sludge using UV, H2O2, and UV/H2O2 oxidation processes: effects of reaction conditions and sludge matrix, Sci. Total Environ., 2014, 493, 307–323 CrossRef CAS.
- T. W. Haas, Kinetics of the uncatalyzed, alkaline decomposition of hydrogen peroxide, 1960, p. 66 Search PubMed.
- P. Zhou, J. Zhang, Y. Zhang, J. Liang, Y. Liu, B. Liu and W. Zhang, Activation of hydrogen peroxide during the corrosion of nanoscale zero valent copper in acidic solution, J. Mol. Catal. A: Chem., 2016, 424, 115–120 CrossRef CAS.
- H. M. Coleman, V. Vimonses, G. Leslie and R. Amal, Removal of contaminants of concern in water using advance oxidation techniques, Water Sci. Technol., 2007, 55, 301–306 CrossRef CAS.
- E. Sarver, Y. Zhang and M. Edwards, Review of Brass Dezincification Corrosion in Potable Water Systems, Corros. Rev., 2010, 28, 155–196 CAS.
- H. Lin and G. S. Frankel, Atmospheric corrosion of Cu by UV, ozone and NaCl, Corros. Eng., Sci. Technol., 2013, 48, 461–468 CrossRef CAS.
- X. Zhang, X. Liu, I. Odnevall Wallinder and C. Leygraf, The protective role of hydrozincite during initial corrosion of a Cu40Zn alloy in chloride-containing laboratory atmosphere, Corros. Sci., 2016, 103, 20–29 CrossRef CAS.
- P. A. Zhdan and J. E. Castle, Corrosion of brass in ultrapure water, Surf. Interface Anal., 2002, 34, 180–184 CrossRef CAS.
- S. Li and W. Sun, Photocatalytic degradation of 17α-ethinylestradiol in mono- and binary systems of fulvic acid and Fe(III): application of fluorescence excitation/emission matrixes, Chem. Eng. J., 2014, 237, 101–108 CrossRef CAS.
- I. M. Ramírez-Sánchez, S. Tuberty, M. Hambourger and E. R. Bandala, Resource efficiency analysis for photocatalytic degradation and mineralization of estriol using TiO2 nanoparticles, Chemosphere, 2017, 184, 1270–1285 CrossRef.
- C.-H. Liao and M. D. Gurol, Chemical Oxidation by Photolytic Decomposition of Hydrogen Peroxide, Environ. Sci. Technol., 1995, 29, 3007–3014 CrossRef CAS.
- S. Lu
ák and P. Sedlák, Photoinitiated reactions of hydrogen peroxide in the liquid phase, J. Photochem. Photobiol., A, 1992, 68, 1–33 CrossRef. - G. F. IJpelaar, D. J. H. Harmsen, E. F. Beerendonk, R. C. van Leerdam, D. H. Metz, A. H. Knol, A. Fulmer and S. Krijnen, Comparison of Low Pressure and Medium Pressure UV Lamps for UV/H2O2 Treatment of Natural Waters Containing Micro Pollutants, Ozone: Sci. Eng., 2010, 32, 329–337 CrossRef CAS.
- J. R. Bolton and M. I. Stefan, Fundamental photochemical approach to the concepts of fluence (UV dose) and electrical energy efficiency in photochemical degradation reactions, Res. Chem. Intermed., 2002, 28, 857–870 CrossRef CAS.
- D. B. Miklos, C. Remy, M. Jekel, K. G. Linden, J. E. Drewes and U. Hübner, Evaluation of advanced oxidation processes for water and wastewater treatment – a critical review, Water Res., 2018, 139, 118–131 CrossRef CAS.
- D. A. Devault, T. Néfau, Y. Levi and S. Karolak, The removal of illicit drugs and morphine in two waste water treatment plants (WWTPs) under tropical conditions, Environ. Sci. Pollut. Res., 2017, 24, 25645–25655 CrossRef CAS.
- Y. Valcárcel, F. Martínez, S. González-Alonso, Y. Segura, M. Catalá, R. Molina, J. C. Montero-Rubio, N. Mastroianni, M. López de Alda, C. Postigo and D. Barceló, Drugs of abuse in surface and tap waters of the Tagus River basin: heterogeneous photo-Fenton process is effective in their degradation, Environ. Int., 2012, 41, 35–43 CrossRef.
- M. Catalá, N. Domínguez-Morueco, A. Migens, R. Molina, F. Martínez, Y. Valcárcel, N. Mastroianni, M. López de Alda, D. Barceló and Y. Segura, Elimination of drugs of abuse and their toxicity from natural waters by photo-Fenton treatment, Sci. Total Environ., 2015, 520, 198–205 CrossRef.
- C. A. Martínez-Huitle, M. A. Quiroz, C. Comninellis, S. Ferro and A. De Battisti, Electrochemical incineration of chloranilic acid using Ti/IrO2, Pb/PbO2 and Si/BDD electrodes, Electrochim. Acta, 2004, 50, 949–956 CrossRef.
- W. Bors, C. Michel and M. Saran, On the nature of biochemically generated hydroxyl radicals. Studies using the bleaching of p-nitrosodimethylaniline as a direct assay method, Eur. J. Biochem., 1979, 95, 621–627 CrossRef CAS.
- N. N. Barashkov, D. Eisenberg, S. Eisenberg, G. S. Shegebaeva, I. S. Irgibaeva and I. I. Barashkova, Electrochemical chlorine-free AC disinfection of water contaminated with Salmonella typhimurium bacteria, Russ. J. Electrochem., 2010, 46, 306–311 CrossRef CAS.
- L. Zang, P. Qu, J. Zhao, T. Shen and H. Hidaka, Photocatalytic bleaching of p-nitrosodimethylaniline in TiO2 aqueous suspensions: a kinetic treatment involving some primary events photoinduced on the particle surface, J. Mol. Catal. A: Chem., 1997, 120, 235–245 CrossRef.
- A. B. R. Farhataziz, Selected specific rates of reactions of transients from water in aqueous solutions III: hydroxyl radical and perhydroxyl radical and their radical ions, Washington, 1977 Search PubMed.
- M. N. Desai and V. K. Shah, Aromatic amines as corrosion inhibitors for 70/30 brass in nitric acid, Corros. Sci., 1972, 12, 725–730 CrossRef CAS.
- G. C. Hobbs and J. Abbot, The Role of the Hydroxyl Radical in Peroxide Bleaching Processes, J. Wood Chem. Technol., 1994, 14, 195–225 CrossRef CAS.
- D. Yano, M. Murayama, M. Takahashi, H. Kobayashi and K. Yamanaka, Inhibition of Copper Corrosion by Removal of H2O2 From CO2-Dissolved Water Using Palladium Catalysts, ECS Trans., 2013, 58, 151–158 CrossRef.
- A. N. Pham, G. Xing, C. J. Miller and T. D. Waite, Fenton-like copper redox chemistry revisited: hydrogen peroxide and superoxide mediation of copper-catalyzed oxidant production, J. Catal., 2013, 301, 54–64 CrossRef CAS.
- L. C. Friedrich, M. A. Mendes, V. O. Silva, C. L. P. S. Zanta, A. Machulek Jr and F. H. Quina, Mechanistic implications of zinc(II) ions on the degradation of phenol by the Fenton reaction, J. Braz. Chem. Soc., 2012, 23, 1372–1377 CrossRef CAS.
- T. Ozawa and A. Hanaki, The first ESR spin-trapping evidence for the formation of hydroxyl radical from the reaction of copper(II) complex with hydrogen peroxide in aqueous solution, J. Chem. Soc., Chem. Commun., 1991, 330–332 RSC.
- J. K. Kim and I. S. Metcalfe, Investigation of the generation of hydroxyl radicals and their oxidative role in the presence of heterogeneous copper catalysts, Chemosphere, 2007, 69, 689–696 CrossRef CAS.
- P. Qiu and C. Leygraf, Initial oxidation of brass induced by humidified air, Appl. Surf. Sci., 2011, 258, 1235–1241 CrossRef CAS.
- R. Yatskiv, S. Tiagulskyi, J. Grym, J. Vaniš, N. Bašinová, P. Horak, A. Torrisi, G. Ceccio, J. Vacik and M. Vrňata, Optical and electrical characterization of CuO/ZnO heterojunctions, Thin Solid Films, 2020, 693, 137656 CrossRef CAS.
- P. Nandi and D. Das, ZnO-CuxO heterostructure photocatalyst for efficient dye degradation, J. Phys. Chem. Solids, 2020, 143, 109463 CrossRef CAS.
- V. Augugliaro, V. Loddo, M. Pagliaro, G. Palmisano and L. Palmisano, Clean by Light Irradiation: Practical Applications of Supported TiO2, RSC Publishing, Cambridge, UK, 2010 Search PubMed.
- P. Zhou, An in situ kinetic investigation of the selective dissolution mechanism of Cu alloys, PhD thesis, Pierre and Marie Curie University, 2017, 181.
- L. Burzyńska, Comparison of the spontaneous and anodic processes during dissolution of brass, Corros. Sci., 2001, 43, 1053–1069 CrossRef.
- O. Kozaderov, K. Shikhaliev, C. Prabhakar, A. Tripathi, D. Shevtsov, A. Kruzhilin, E. Komarova, A. Potapov, I. Zartsyn and Y. Kuznetsov, Corrosion of α-Brass in Solutions Containing Chloride Ions and 3-Mercaptoalkyl-5-amino-1H-1,2,4-triazoles, Appl. Sci., 2019, 9, 2821 CrossRef CAS.
- M. C. Bastos, L. F. A. Proença, M. M. M. Neto and I. T. E. Fonseca, Electrochemical studies on the corrosion of brass in seawater under anaerobic conditions, J. Solid State Electrochem., 2008, 12, 121–131 CrossRef CAS.
- D. P. Whittle and G. C. Wood, The Establishment and Growth of Zinc Oxide on 74:26 Brass, Br. Corros. J., 1968, 3, 294–300 CrossRef CAS.
- G. Hultquist, P. Szakálos, M. J. Graham, A. B. Belonoshko, G. I. Sproule, L. Gråsjö, P. Dorogokupets, B. Danilov, T. AAstrup, G. Wikmark, G.-K. Chuah, J.-C. Eriksson and A. Rosengren, Water Corrodes Copper, Catal. Lett., 2009, 132, 311–316 CrossRef CAS.
- E. A. Thompson and T. D. Burleigh, Accelerated corrosion of zinc alloys exposed to ultraviolet light, Corros. Eng., Sci. Technol., 2007, 42, 237–241 CrossRef CAS.
- M. Li, Z. Wang, Q. Zhang, C. Qin, A. Inoue and W. Guo, Formation and evolution of ultrathin Cu2O nanowires on NPC ribbon by anodizing for photocatalytic degradation, Appl. Surf. Sci., 2020, 506, 144819 CrossRef CAS.
- B. Y. Valles-Pérez, M. A. Badillo-Ávila, G. Torres-Delgado, R. Castanedo-Pérez and O. Zelaya-Ángel, Photocatalytic activity of ZnO
+
CuO thin films deposited by dip coating: coupling effect between oxides, J. Sol-Gel Sci. Technol., 2020, 93, 517–526 CrossRef. - H. Yoo, S. Kahng and J. Hyeun Kim, Z-scheme assisted ZnO/Cu2O-CuO photocatalysts to increase photoactive electrons in hydrogen evolution by water splitting, Sol. Energy Mater. Sol. Cells, 2020, 204, 110211 CrossRef CAS.
Footnote |
† Electronic supplementary information (ESI) available. See DOI: 10.1039/d0ra06153k |
|
This journal is © The Royal Society of Chemistry 2020 |
Click here to see how this site uses Cookies. View our privacy policy here.