DOI:
10.1039/D0SC02605K
(Edge Article)
Chem. Sci., 2020,
11, 8989-8998
Extremely low-frequency phonon material and its temperature- and photo-induced switching effects†
Received
7th May 2020
, Accepted 5th August 2020
First published on 17th August 2020
Abstract
Atomic vibrations due to stretching or bending modes cause optical phonon modes in the solid phase. These optical phonon modes typically lie in the frequency range of 102 to 104 cm−1. How much can the frequency of optical phonon modes be lowered? Herein we show an extremely low-frequency optical phonon mode of 19 cm−1 (0.58 THz) in a Rb-intercalated two-dimensional cyanide-bridged Co–W bimetal assembly. This ultralow frequency is attributed to a millefeuille-like structure where Rb ions are very softly sandwiched between the two-dimensional metal–organic framework, and the Rb ions slowly vibrate between the layers. Furthermore, we demonstrate temperature-induced and photo-induced switching of this low-frequency phonon mode. Such an external-stimulation-controllable sub-terahertz (sub-THz) phonon crystal, which has not been reported before, should be useful in devices and absorbers for high-speed wireless communications such as beyond 5G or THz communication systems.
Introduction
A monoatomic molecule in the gas or liquid phase moves with free motion. Such a movement of a monomolecule is called a translational mode (Fig. 1(i)). By contrast, bonded atoms in a molecule or a solid phase vibrate with other atoms coherently, which is called the vibrational mode. The vibrational motion is observed as the optical phonon mode.1–7 Typical stretching vibrational modes are observed at several tens of terahertz (THz), e.g., CO stretching mode (2143 cm−1, 64 THz) and CN stretching mode (2200 cm−1, 66 THz). If a heavy monoatomic molecule is softly caught by an organic cage or a metal–organic framework cage (Fig. 1(ii)), the translational mode switches to a vibrational mode. The vibrational frequency of the trapped monoatom should be lower than that of a typical bonded atom. To design a low-frequency optical phonon mode, the size and shape of the cage and the chemical affinity of the surrounding framework are both important. Recently, a Cs+ ion encapsulated in a three-dimensional (3D) –M–C
N–M′– framework of a Prussian blue analogue showed a low-frequency vibrational mode of 1.4 THz.8 In this case, the Cs+ ion is surrounded by twelve –C
N– anionic sides of the cubic lattice. By contrast, the number of sides in a two-dimensional (2D) –M–C
N–M′– framework decreases to eight. Thus, the frequency of the vibrational mode should be lower. As shown in Fig. 1(iii), a monoatom in a 2D framework should vibrate at a lower frequency (Movie S1†) compared to that in a hollow box of a 3D framework. From this perspective, soft frameworks based on 2D metal–organic frameworks and coordination polymers9–24 such as cyanide-bridged bimetal assemblies25–40 are strong candidates. The low-frequency vibrational mode (optical phonon) is very attractive from the viewpoint of THz technology.41–43 To date, several examples of low-frequency phonon modes have been reported.44,45 Because sub-THz light-resonating materials have potential as optical devices and absorbers for next-generation wireless communications, i.e., millimetre wave communication46–48 or beyond 5G communication,49 they will take an important role in the era of big data and the Internet of Things (IoT).50,51 Additionally, from the viewpoint of thermoelectrics, low-frequency optical phonon modes due to atomic rattlers have drawn attention.52–55
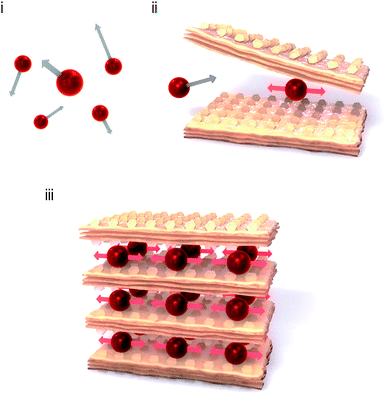 |
| Fig. 1 Strategy to construct an extremely low-frequency optical phonon mode. (i) Monoatomic molecules in the gas phase or liquid phase move with free motion (translational mode). (ii) If a monoatomic molecule is caught by a two-dimensional (2D) metal–organic soft framework, the translational mode switches to a vibrational mode with a low vibrational frequency. (iii) The material in the present work, that is, a 2D layered metal–organic framework with heavy atoms which are lightly enfolded by the soft framework with organic ligands. | |
In the present work, we synthesise a Rb-intercalated 2D cyanide-bridged Co–W bimetal assembly, RbI[CoII(3-cyanopyridine)2][WV(CN)8] (RbCoW), and investigate its optical phonon mode by THz time-domain spectroscopy (THz-TDS). Additionally, its temperature- and photo-induced switching effects are examined from the viewpoint of dynamic functionalities.
Results and discussion
Material and crystal structure
A single crystal of RbCoW was obtained by the slow diffusion method in an H-shaped tube. An aqueous solution of cobalt dichloride hexahydrate, 3-cyanopyridine, and rubidium chloride was diffusively mixed with an aqueous solution of rubidium octacyanidetungstate hydrate and rubidium chloride. After one month, a crystal was obtained. The powder-form sample was obtained by mixing a solution of cobalt dichloride, 3-cyanopyridine, rubidium chloride, and rubidium octacyanidetungstate with a magnetic stirrer for one day. The precipitates were collected and placed under vacuum conditions at room temperature. Inductively coupled plasma mass spectrometry and elemental analysis showed that the formula is RbI[CoII(3-cyanopyridine)2][WV(CN)8] (see Methods).
Single-crystal X-ray analyses revealed that the crystal structure of RbCoW at 300 K is a triclinic system in the P
space group with cell parameters of a = 7.5298(4) Å, b = 13.7545(7) Å, c = 14.0687(6) Å, α = 119.276(4)°, β = 101.020(6)°, γ = 89.974(6)°, and V = 1240.15(12) Å3 (Fig. 2 and Table S1†). The Co–W bridged by cyanides forms 2D layers in the ab-plane, which are stacked along the c-axis and involves the Rb ions between the layers. The asymmetric unit consists of two halves of Co ions, a [W(CN)8] ion, two 3-cyanopyridine molecules, and a Rb ion. The coordination geometry of [W(CN)8] ion is close to a bicapped trigonal prism (C2v). Four cyanide ligands of the [W(CN)8] ion are coordinated to the nearest neighbouring Co ions, and the other four cyanide ligands are free. The coordination geometry of the Co ion is a six-coordinated pseudo-octahedron (D4h). The two axial positions of the Co ion are occupied by the nitrogen atoms of two 3-cyanopyridine molecules, while the cyanide nitrogen atoms of [W(CN)8] ion are in the four equatorial positions.
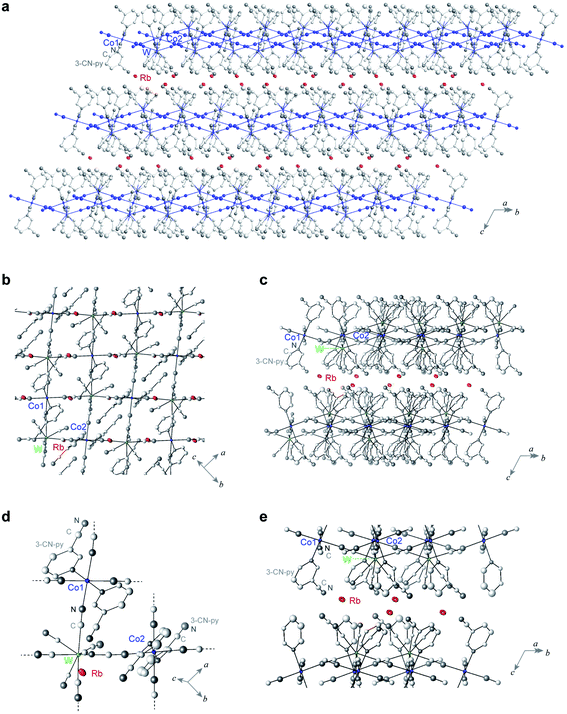 |
| Fig. 2 Crystal structure of RbCoW. (a) Rb-intercalated 2D cyanide-bridged Co–W bimetal assembly. Red ellipsoids indicate the Rb ions. Co, W, C, and N atoms forming the 2D network are shown as blue ellipsoids and balls, whereas the other C and N atoms are represented as light grey and grey balls. (b) Projection of the 2D network layer in the ab-plane. (c) Side view of the layers. (d) Coordination structure of RbCoW. (e) Structural environment around the Rb ions between the layers. Red, blue, and green ellipsoids represent Rb, Co, and W atoms, and light grey and grey balls represent C and N atoms, respectively. Displacement ellipsoids and balls are drawn at the 30% probability level. H atoms are omitted for clarity. | |
First-principles phonon mode calculations
To estimate the frequency of the optical phonon modes of RbCoW, the phonon modes were calculated by the Phonon code from the determined crystal structure and atomic positions (Tables S2–S4,† Methods). The phonon density of states (phonon-DOS) is shown in Fig. 3a and S1.† Additionally, the optical phonon spectrum was calculated based on the transition probabilities of the vibrational modes. The calculated optical phonon mode spectrum shows that RbCoW has eight absorptions in the THz region, i.e., peaks at 0.883 (peak a), 0.997 (peak b), 1.136 (peak c), 1.453 (peak d), 1.682 (peak e), 1.805 (peak f), 1.923 (peak g), and 2.102 THz (peak h) (Fig. 3b). From the video of the atomic movements (Movie S2†), peaks a–c are assigned to the phonon modes of Rb ion vibrations in the ab-plane, while peak d is mainly due to Rb ion vibrations along the crystallographic c-axis. In these phonon modes, the 3-cyanopyridine and cyanide ligands sway together with the Rb ions, supporting the Rb movements. Peaks e and f are attributed to a combination of transverse translational modes of W–CN–Co and 3-cyanopyridine ligand rotation, while peaks g and h originate from the transverse librational modes of W–CN–Co and 3-cyanopyridine ligand rotation. Fig. 3c shows the atomic movements of the intense absorption peaks a–e, and h.
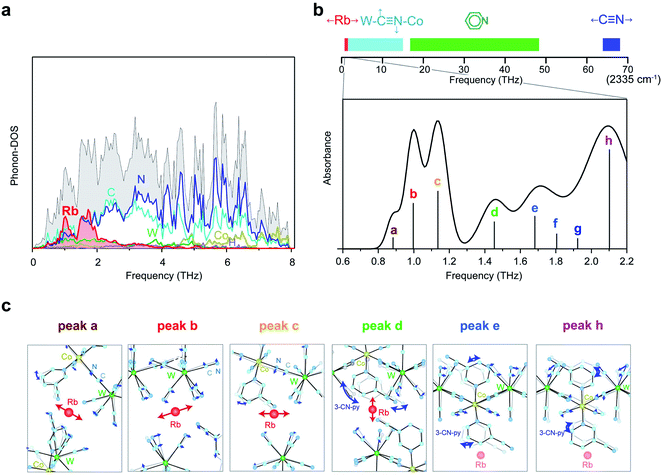 |
| Fig. 3 First-principles phonon mode calculations. (a) Phonon density of states (phonon-DOS) of RbCoW. Black, red, dark yellow, green, light blue, blue, and purple lines indicate the total phonon-DOS and the partial phonon-DOS of Rb, Co, W, C, N, and H, respectively. (b) Upper part is the frequency bar in the rage of 0–70 THz (0–2335 cm−1) indicating the phonon modes of RbCoW. Red, light blue, green, and blue bars represent the frequency regions of the Rb vibration, transverse translational and transverse librational modes of the CN ligands, phonon modes of the pyridine ligands, and CN stretching modes, respectively. The low-frequency region of 0.6–2.2 THz is enlarged to show the optical phonon spectrum calculated from the IR active phonon modes. (c) Atomic movements of the phonon modes corresponding to peaks a–e and h. Peaks a–c are assigned to the phonon modes of Rb ion vibrations in the ab-plane. Atomic movement of peak d is due to Rb ion vibrations along the crystallographic c-axis and 3-cyanopyridine ligand rotation. Peak e is attributed to the combination of transverse translational modes of W–CN–Co and 3-cyanopyridine ligand rotation, and peak h originates from the transverse librational modes of W–CN–Co and 3-cyanopyridine ligand rotation. | |
Optical phonon mode measurements by THz-TDS
Optical phonon modes of RbCoW were measured by THz-TDS. The optical setup is shown in Fig. 4a. The temporal spectrum of the THz pulse wave was acquired in the transmittance mode. Fourier transformation of the temporal waveform yielded the frequency-dependent power logarithm spectrum. The THz absorption spectrum shows that RbCoW has eight absorption peaks: 0.58, 0.78, 1.06, 1.22, 1.30, 1.41, 1.51, and 1.89 THz (Fig. 4b). These observed peaks agree well with peaks a–h in the calculated optical phonon spectrum. Thus, RbCoW possesses an extremely low-frequency optical phonon due to the movement of the Rb ion intercalated between the layers. Such low-frequency optical phonons, with only
of the frequency of a CN stretching mode, are rarely observed.
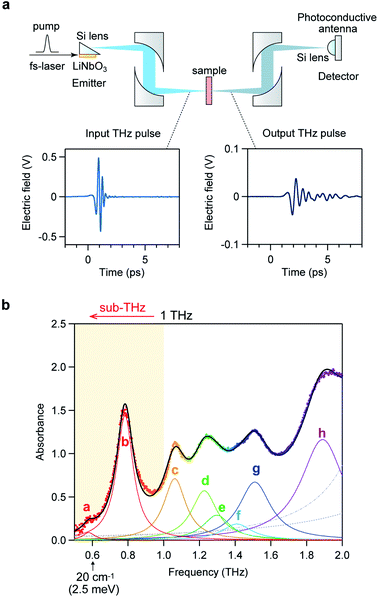 |
| Fig. 4 THz-TDS measurement of RbCoW. (a) Setup of the THz-TDS measurement system and the temporal waveforms of the input and output THz pulses. (b) THz absorption spectrum of RbCoW with peaks at 0.58 (peak a), 0.78 (peak b), 1.06 (peak c), 1.22 (peak d), 1.30 (peak e), 1.41 (peak f), 1.51 (peak g), and 1.89 THz (peak h). Black line shows the fitted curve, coloured lines show the components of each peak, and dots indicate the experimental data. Faint dashed grey lines represent the fitted curves for the higher frequency phonon modes at 2.34 and 2.94 THz. | |
Temperature-induced switching of low-frequency phonon modes
Next, we investigated the temperature dependence of the optical phonon spectra. The peak positions of peaks a–h are almost constant from 300 K to 150 K. However, the intensities of peaks a–h below 150 K decrease and new peaks appear at 0.87, 1.31, and 1.42 THz (Fig. 5a). During the warming process, the intensities of these new peaks decrease, and peaks a–h are recovered at 190 K (Fig. 5b). The spectral simulation indicates that the optical phonon spectra below 150 K are composed of eight peaks (Fig. S2†): 0.69 (peak i), 0.87 (peak j), 0.91 (peak k), 1.14 (peak l), 1.31 (peak m), 1.42 (peak n), 1.58 (peak o), and 1.75 THz (peak p).
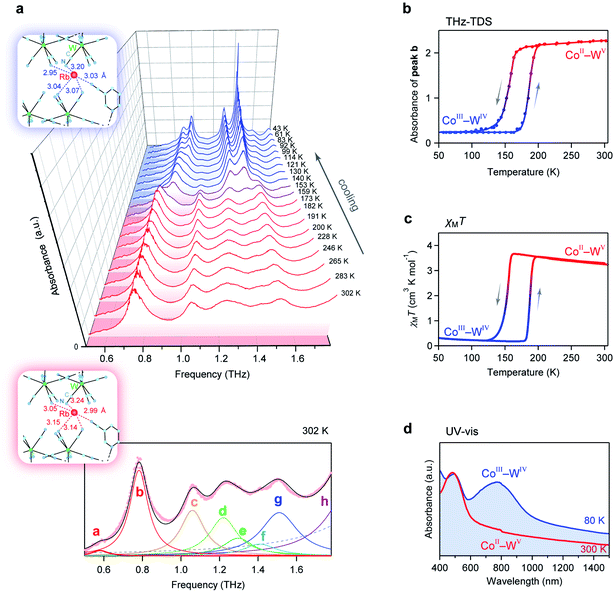 |
| Fig. 5 Temperature-induced switching effect of the phonon modes. (a) Temperature dependence of the THz absorption spectra. Upper blue and lower pink frames show the coordination environments around the Rb ion at 130 K and 300 K, respectively. Lower figure shows the fitting of the absorption spectrum at 302 K. Black line shows the fitted curve, coloured lines show the components of each peak, and pink dots indicate the experimental data. Faint dashed grey line represents the fitted curve of the higher frequency phonon mode. (b) Temperature dependence plot of the intensity of peak b at 0.78 THz. (c) χMT vs. T plot from the magnetic measurement. (d) UV-vis spectra measured at 80 K (blue line) and 300 K (red line). | |
To understand this temperature-induced switching effect, we measured the temperature dependence of the magnetic susceptibility (χM) using a superconducting quantum interference device (SQUID) because both CoII and WV have magnetic spins. Fig. 5c shows the product of χM and temperature (T) vs. T plot. The χMT value of RbCoW at 300 K is 3.24 K cm3 mol−1. This agrees well with the calculated value of 3.30 K cm3 mol−1 based on CoII high spin (HS) (S = 3/2) and WV (S = 1/2) with gCo of 2.50 and gW of 2.00. The χMT value decreases at 150 K and becomes 0.23 K cm3 mol−1. This small χMT value indicates that the low-temperature phase below 150 K has valence states of CoIII low spin (LS) (S = 0) and WIV (S = 0). The transition temperature in the χMT vs. T plot agrees with that in the temperature-dependent THz-TDS data. By contrast, in the warming process, the CoIII(LS)–WIV phase returns to the CoII(HS)–WV phase at 190 K. Therefore, the temperature-induced switching effect of the low-frequency phonon mode of RbCoW can be explained by a charge-transfer-induced spin transition (CTIST).56–58 That is, it is a phase transition between the CoII–WV phase and the CoIII–WIV phase. Fig. 5d shows the UV-vis spectra of the CoII–WV phase at 300 K and the CoIII–WIV phase at 80 K.
The crystal structure of the CoIII–WIV low-temperature phase has a triclinic structure (space group = P
) with crystallographic parameters of a = 7.2253(14) Å, b = 13.340(3) Å, c = 13.839(3) Å, α = 118.671(8)°, β = 100.497(7)°, γ = 90.100(6)°, and V = 1144.6(4) Å3 at 130 K (Fig. S3 and Table S5†), which is isostructural with the CoII–WV phase at 300 K. The lattice constants of the a-, b-, and c-axes of the CoIII–WIV low-temperature phase at 130 K are compressed by 4.2%, 3.1%, and 1.7%, respectively, compared with those of the CoII–WV phase at 300 K. As the temperature decreases, the CoII(S = 3/2)–WV phase shows a charge-transfer-induced phase transition to the CoIII(S = 0)–WIV phase and is accompanied by a spin-crossover from CoIII(HS) to CoIII(LS). The ionic radius of CoII(HS) is 0.89 Å, while that of CoIII(LS) is 0.69 Å. In fact, the average Co–N bond length shrinks from 2.11(2) Å at 300 K to 1.90(3) Å at 130 K (Table S6†). The distances between the Rb ion and the surrounding nitrogen atoms of the cyanide ligands are shorter compared to those at 300 K. Thus, the frequency shifts of the optical phonon modes from 0.58 (peak a) to 0.69 THz (peak i) and from 0.78 (peak b) to 0.87 THz (peak j) are caused by a stronger Rb trapping force due to the contraction of the Rb–N distances (Table S7†).
Photo-induced switching of low-frequency phonon mode
We investigated the photo-induced switching effect of the low-frequency optical phonon modes of RbCoW. At 80 K, RbCoW takes a CoIII–WIV phase and possesses an intense absorption around 780 nm (Fig. 5d). To assign the UV-vis absorption spectrum, the electronic structure of the CoIII–WIV phase of RbCoW was calculated by first-principles calculations using the Vienna Ab initio Simulation Package (VASP) program (see Methods). The density of states (DOS) showed that the top of the valence band just below the Fermi energy (EF) mainly consists of WIV and N components (Co 0%, W 63%, N 37%, C 0%, H 0%), whereas the CoIII and N components (Co 59%, W 0%, N 27%, C 11%, H 3%) mainly contribute to the bottom of the conduction band just above EF (Fig. 6a(i) and S4†). This indicates that the lowest-energy transition in this compound is a charge transfer from WIV to CoIII. Fig. 6a(ii) shows the calculated optical absorption spectrum, which well reproduces the experimental spectrum.
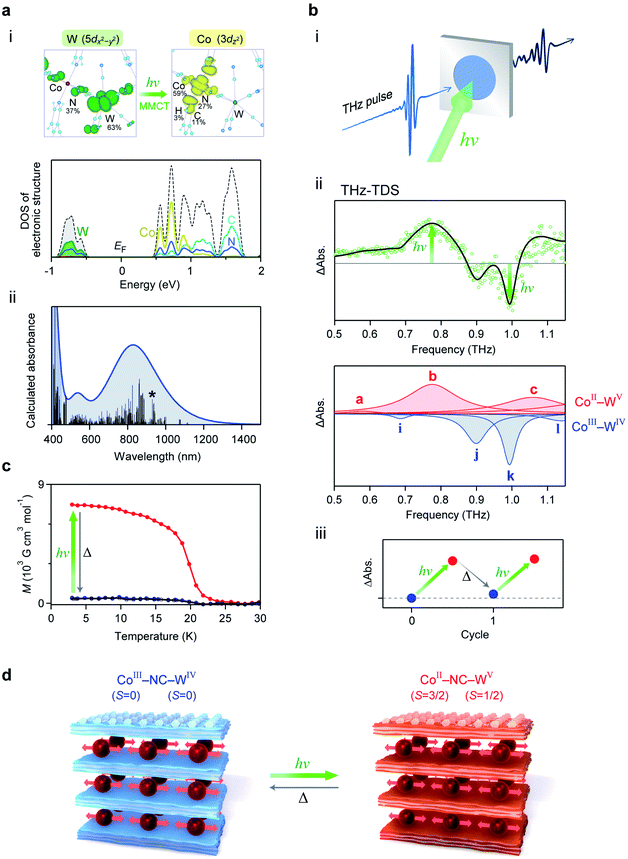 |
| Fig. 6 Electronic structure and photo-induced switching of low-frequency phonon mode. (a) (i) Upper figures show the charge density maps of the valence band top (left) and the conduction band bottom (right) of the allowed transition. Lower graph is the density of states (DOS) of the CoIII–WIV phase near the Fermi energy (EF) obtained from first-principles electronic structure calculations. Black dotted line shows the total DOS. Dark yellow, green, light blue, and blue lines indicate the partial density of states for Co, W, C, and N, respectively. (ii) Calculated optical absorption spectrum from the transition probabilities of the RbCoW CoIII–WIV phase obtained by first-principles calculation. Black bars show the calculated transition probabilities. Line with an asterisk represents the transition corresponding to the transition between the states of the charge density maps in (i). (b) (i) Illustration of the THz-TDS measurement under photo-irradiation. (ii) Upper shows the differential spectrum between the THz absorption spectra before and after light irradiation (green open circles) and the simulated spectrum (black line). The red and blue peaks in the lower graph represent the components from the CoII–WV phase and CoIII–WIV phase, respectively. Based on the temperature-induced phase transition between the CoII–WV and CoIII–WIV phases in Fig. 5a, the ratio of the peak area between these two phases is 1 : 0.63. Differential spectrum was fitted using the ratio of the peak area between the CoII–WV and CoIII–WIV phases. (iii) Photo-thermal reversibility observed as the difference of the THz absorption spectra at 0.78 THz, where the pre-photoirradiation value is set as the standard. (c) Magnetisation vs. temperature plot under a magnetic field of 100 Oe before (blue) and after (red) irradiation with a 785 nm laser at 3 K, and after thermal annealing up to 120 K (black). (d) Illustration of the photo-induced switching between the CoIII–WIV phase and the CoII–WV phase of the 2D layered metal–organic framework. | |
Since the CoIII–WIV phase has an optical transition around 780 nm, 785 nm light was irradiated to the sample inside the THz-TDS system (Fig. 6b(i)). Fig. 6b(ii) shows the observed differential spectrum of the experimental THz spectra before and after irradiation. The absorption peaks at 0.78 THz and 1.08 THz increase by light irradiation, while peaks at 0.90 THz and 0.99 THz decrease. From the simulation based on the change in the ratio between the CoIII–WIV and CoII–WV phases, the increasing components at 0.78 THz and 1.08 THz correspond to peaks b and c of the CoII–WV phase, respectively, while the decreasing components at 0.90 THz and 0.99 THz correspond to peaks j and k of the CoIII–WIV phase, respectively. Additionally, this photo-induced switching effect on the optical phonon mode is repeatedly observed by light irradiation and thermal annealing (5 K → 120 K → 5 K) (Fig. 6b(iii)).
To confirm the mechanism of the photo-induced switching effect, a photoirradiation experiment was performed inside the SQUID magnetometer at 3 K. The observed spontaneous magnetisation with a Curie temperature of 20 K and a coercive field of 4.2 kOe can be explained by the ferromagnetic ordering between the photo-generated CoII and WV (Fig. 6c). Therefore, the photo-induced optical phonon switching of the low-frequency phonon mode originates from the photo-induced phase transition from the CoIII–WIV to the CoII–WV phase, i.e., an optically induced CTIST effect (Fig. 6d).
Conclusion
From the viewpoint of phonon design, we demonstrate extremely low-frequency optical phonon modes of 0.58 THz (580 GHz, 2.4 meV) and 0.78 THz (780 GHz, 3.2 meV). Such sub-THz optical phonon modes, with only
of the frequency of a CN stretching mode, are successfully realised by sandwiching a heavy monoatomic ion by a 2D metal–organic soft framework. Furthermore, in an isostructural analogue compound where Rb is replaced with Cs, the optical phonon modes are observed at even lower frequencies of 0.57, 0.69, and 0.86 THz because Cs is heavier than Rb (Fig. S5†). This study demonstrates that extremely low-frequency optical phonon modes can be designed by selecting the types of heavy ions to be encapsulated, organic ligands, and transition metal ions. It will be possible to design even lower optical phonon modes by choosing the suitable organic ligands. Sub-THz phonon materials may be useful for electromagnetic wave devices as next-generation wireless communications such as beyond 5G and THz communications in an IoT society.
Furthermore, from the viewpoint of dynamic functions of the low-frequency phonon mode, we show temperature- and photo-induced switching effects on the sub-THz phonon modes. Various functionalities such as magnetism, ionic conduction, and external-stimulation responsivity into the 2D metal–organic framework layers can be incorporated using low-frequency phonon 2D layers to study the synergistic effects between these functionalities and the low-frequency optical phonons. For example, an effective way to switch the phonon frequency by external stimuli is to include external-stimuli responsive units such as spin-crossover,59–67 charge-transfer,56–58,68–76 and photo-isomerisation units,77–80 into the framework. External-stimulation-tuneable THz or sub-THz phonon crystals may lead to the development of devices such as optical filters and optical shutters for THz or sub-THz lights.
Methods
Material
Single crystals were prepared by the following procedure. An aqueous solution (2 cm3) of cobalt dichloride hexahydrate (0.3 mmol), 3-cyanopyridine (0.6 mmol), and rubidium chloride (2.7 mmol) was placed on one side of the H-shaped tube, while an aqueous solution (2 cm3) of rubidium octacyanidetungstate hydrate (0.3 mmol) and rubidium chloride (2.7 mmol) was added on the other side. Additionally, a rubidium chloride aqueous solution (0.35 mol dm−3) was used as a buffer solution. After standing for one month, red platelet crystals appeared. The collected crystals were dried under a vacuum or Ar atmosphere at room temperature to give crystals of the target material. Elemental analysis calcd: Co 7.9, W 24.7, Rb 11.5, C 32.3, H 1.1, N 22.6; found: Co 8.1, W 24.7, Rb 11.2, C 32.2, H 1.2, N 22.7.
Single-crystal X-ray diffraction measurements
Single-crystal X-ray diffraction measurements for RbCoW at 300 K and 130 K were performed under a nitrogen flow using a Rigaku R-AXIS Rapid imaging plate area detector with graphite monochromated Mo Kα radiation. The crystal was covered with Apiezon N to prevent hydration. The average correction of the diffraction data was performed in the crystal structure program in Rigaku. The crystal structures at 300 K and 130 K were solved by a direct method using SHELXS-97 on Olex 2-1.2 software. CCDC-1945288 and CCDC-1945289† contain the structural information for the CoII–WV phase at 300 K and the CoIII–WIV phase at 130 K, respectively.
Phonon mode calculation
First-principles phonon mode calculations were conducted using the Phonon code.81 The atomic positions were optimised with an energy cutoff of 500 eV and a 3 × 3 × 3 k-mesh until satisfying a 10−5 eV pm−1 force tolerance in each atom. The phonon mode calculations used 2 × 1 × 1 supercells of the optimised crystal structures. The direct method implemented in the phonon code had a displacement of 2 pm. The transition probabilities of the infrared active optical phonon modes were also calculated. Table S2† shows the list of optical phonon modes.
THz wave absorption measurements
The absorption properties of the polycrystalline sample in the measurement range of 0.5–2 THz were measured by THz-TDS system of Advantest TAS7400TS in the transmittance mode. The measurement at 293 K was performed by forming a polycrystalline sample into a pellet with a thickness of 0.72 mm. The variable-temperature THz absorption measurement was performed using a pellet sample with a thickness of 1.36 mm by placing it between circular polyethylene plates and pressing with a copper wire to improve the thermal connectivity between the sample and cryostat. The photoirradiation measurement of THz spectroscopy was performed by dispersing RbCoW with nujol under an Ar atmosphere and covering between polypropylene plates. The dispersed sample thickness was 0.18 mm.
Magnetic measurements
The magnetic properties were measured using a Quantum Design MPMS 5 SQUID magnetometer. The sample for magnetic property measurements was prepared by placing a polycrystalline sample in a quartz cell. The magnetic susceptibility of RbCoW was measured under 5000 Oe. To measure photo-induced magnetisation, the powder-form sample of RbCoW was spread onto tape and placed on the edge of an optical fibre. Then 785 nm continuous wave laser light was irradiated at 3 K.
Electronic structure calculation
Periodic electronic structure calculation of the CoII–WV phase was carried out using the VASP program.82,83 To take into account the van der Waals interaction, the calculations were based on the van der Waals density functional rev-vdW-DF2. The U–J parameters were set to 7.0 eV for Co 3d electrons and 2.6 eV for W 5d electrons. The wave functions were based on the plane wave with an energy cutoff of 500 eV. A k-mesh of 3 × 3 × 3 was used for Brillouin zone samplings. The electronic iteration convergence was set to 1 × 10−9 eV. Partial DOS were calculated by integrating over the volumes of atomic spheres on atoms with the following radii: W: 1.30 Å, Co: 1.16 Å, N: 0.75 Å, C: 0.77 Å, Rb: 2.16 Å and H: 0.32 Å. The distributions of the charge densities were estimated by Bader analysis.
Conflicts of interest
There are no conflicts to declare.
Acknowledgements
The present research was supported in part by a JSPS Grant-in-Aid for Specially Promoted Research Grant Number 15H05697, Grant-in-Aid for Scientific Research on Innovative Area Soft Crystals (area No. 2903, 17H06366), Grant-in-Aid for Scientific Research(A) Grant Number 20H00369, and IM-LED LIA (CNRS). We acknowledge the Cryogenic Research Center, the University of Tokyo, the Center for Nano Lithography & Analysis, The University of Tokyo supported by MEXT, and Quantum Leap Flagship Program (Q-LEAP) by MEXT. K. N. is thankful to JSPS KAKENHI Grant Number 19K05366 and the Ogasawara Foundation for the Promotion of Science & Engineering. We appreciate Dr K. Nakagawa for the helpful discussions.
References
- A. Einstein, Ann. Phys., 1905, 322, 549–560 CrossRef.
- P. E. Morse, Phys. Rev., 1929, 34, 57–64 CrossRef CAS.
- C. Zener, Phys. Rev., 1931, 37, 556–569 CrossRef CAS.
- M. Born and H. S. Green, Proc. R. Soc. A, 1946, 188, 10–18 CAS.
- G. J. Snyder and E. S. Toberer, Nat. Mater., 2008, 7, 105–114 CrossRef CAS PubMed.
- H.-H. Kim, S. M. Souliou, M. E. Barber, E. Lefrançois, M. Minola, M. Tortora, R. Heid, N. Nandi, R. A. Borzi, G. Garbarino, A. Bosak, J. Porras, T. Loew, M. König, P. J. W. Moll, A. P. Mackenzie, B. Keimer, C. W. Hicks and M. Le Tacon, Science, 2018, 362, 1040–1044 CrossRef CAS PubMed.
- A. Togo and I. Tanaka, Scr. Mater., 2015, 108, 1–5 CrossRef CAS.
- S. Ohkoshi, M. Yoshikiyo, A. Namai, K. Nakagawa, K. Chiba, R. Fujiwara and H. Tokoro, Sci. Rep., 2017, 7, 8088 CrossRef PubMed.
- H. Furukawa, K. E. Cordova, M. O'Keeffe and O. M. Yaghi, Science, 2013, 341, 1230444 CrossRef PubMed.
- R. Kitaura, S. Kitagawa, Y. Kubota, T. C. Kobayashi, K. Kindo, Y. Mita, A. Matsuo, M. Kobayashi, H.-C. Chang, T. C. Ozawa, M. Suzuki, M. Sakata and M. Takata, Science, 2002, 298, 2358–2361 CrossRef CAS PubMed.
- E. Coronado, J. R. Galán-Mascarós, C. J. Gómez-García and V. Laukhin, Nature, 2000, 408, 447–449 CrossRef CAS PubMed.
- M. Dincă and J. R. Long, Angew. Chem., Int. Ed., 2008, 47, 6766–6779 CrossRef PubMed.
- A. Carne-Sanchez, I. Imaz, M. Cano-Sarabia and D. Maspoch, Nat. Chem., 2013, 5, 203–211 CrossRef CAS PubMed.
- H. I. Karunadasa, C. J. Chang and J. R. Long, Nature, 2010, 464, 1329–1333 CrossRef CAS PubMed.
- M. Verdaguer, A. Bleuzen, V. Marvaud, J. Vaissermann, M. Seuleiman, C. Desplanches, A. Scuiller, C. Train, R. Garde, G. Gelly, C. Lomenech, I. Rosenman, P. Veillet, C. Cartier and F. Villain, Coord. Chem. Rev., 1999, 192, 1023–1047 CrossRef.
- J. S. Miller, Chem. Soc. Rev., 2011, 40, 3266–3296 RSC.
- L. Catala, D. Brinzei, Y. Prado, A. Gloter, O. Stéphan, G. Rogez and T. Mallah, Angew. Chem., Int. Ed., 2008, 48, 183–187 CrossRef PubMed.
- B. Sieklucka, R. Podgajny, P. Przychodzeń and T. Korzeniak, Coord. Chem. Rev., 2005, 249, 2203–2221 CrossRef CAS.
- S. Ohkoshi and H. Tokoro, Acc. Chem. Res., 2012, 45, 1749–1758 CrossRef CAS PubMed.
- A. Abhervé, S. Mañas-Valero, M. Clemente-León and E. Coronado, Chem. Sci., 2015, 6, 4665–4673 RSC.
- E. J. Carrington, C. A. McAnally, A. J. Fletcher, S. P. Thompson, M. Warren and L. Brammer, Nat. Chem., 2017, 9, 882–889 CrossRef CAS PubMed.
- L. S. Xie, L. Sun, R. Wan, S. S. Park, J. A. DeGayner, C. H. Hendon and M. Dincă, J. Am. Chem. Soc., 2018, 140, 7411–7414 CrossRef CAS PubMed.
- M. A. Solomos, F. J. Claire and T. J. Kempa, J. Mater. Chem. A, 2019, 7, 23537–23562 RSC.
- L. E. Darago, M. L. Aubrey, C. J. Yu, M. I. Gonzalez and J. R. Long, J. Am. Chem. Soc., 2015, 137, 15703–15711 CrossRef CAS PubMed.
- S. Ferlay, T. Mallah, R. Ouahès, P. Veillet and M. Verdaguer, Nature, 1995, 378, 701–703 CrossRef CAS.
- S. Ohkoshi, K. Arai, Y. Sato and K. Hashimoto, Nat. Mater., 2004, 3, 857–861 CrossRef CAS PubMed.
- S. Margadonna, K. Prassides and A. N. Fitch, Angew. Chem., Int. Ed., 2004, 43, 6316–6319 CrossRef CAS PubMed.
- S. S. Kaye, H. J. Choi and J. R. Long, J. Am. Chem. Soc., 2008, 130, 16921–16925 CrossRef CAS PubMed.
- S. Ohkoshi, S. Takano, K. Imoto, M. Yoshikiyo, A. Namai and H. Tokoro, Nat. Photonics, 2014, 8, 65–71 CrossRef CAS.
- S. G. Duyker, V. K. Peterson, G. J. Kearley, A. J. Studer and C. J. Kepert, Nat. Chem., 2016, 8, 270–275 CrossRef CAS PubMed.
- S. M. Holmes and G. S. Girolami, J. Am. Chem. Soc., 1999, 121, 5593–5594 CrossRef CAS.
- Ø. Hatlevik, W. E. Buschmann, J. Zhang, J. L. Manson and J. S. Miller, Adv. Mater., 1999, 11, 914–918 CrossRef.
- W. R. Entley and G. S. Girolami, Science, 1995, 268, 397–400 CrossRef CAS PubMed.
- S. Margadonna, K. Prassides and A. N. Fitch, J. Am. Chem. Soc., 2004, 126, 15390–15391 CrossRef CAS PubMed.
- E. Coronado, M. C. Giménez-López, G. Levchenko, F. M. Romero, V. García-Baonza, A. Milner and M. J. Paz-Pasternak, J. Am. Chem. Soc., 2005, 127, 4580–4581 CrossRef CAS PubMed.
- R. Garde, C. Desplanches, A. Bleuzen, P. Veillet and M. Verdaguer, Mol. Cryst. Liq. Cryst., 1999, 334, 587–595 CrossRef CAS.
- Z. J. Zhong, H. Seino, Y. Mizobe, M. Hidai, A. Fujishima, S. Ohkoshi and K. Hashimoto, J. Am. Chem. Soc., 2000, 122, 2952–2953 CrossRef CAS.
- D. Pinkowicz, R. Podgajny, M. Bałanda, M. Makarewicz, B. Gaweł, W. Łasocha and B. Sieklucka, Inorg. Chem., 2008, 47, 9745–9747 CrossRef CAS PubMed.
- S. Ohkoshi, K. Nakagawa, K. Imoto, H. Tokoro, Y. Shibata, K. Okamoto, Y. Miyamoto, M. Komine, M. Yoshikiyo and A. Namai, Nat. Chem., 2020, 12, 338–344 CrossRef CAS PubMed.
- C. Train, R. Gheorghe, V. Krstic, L.-M. Chamoreau, N. S. Ovanesyan, G. L. J. A. Rikken, M. Gruselle and M. Verdaguer, Nat. Mater., 2008, 7, 729–734 CrossRef CAS PubMed.
- K. Wang and D. M. Mittleman, Nature, 2004, 432, 376–379 CrossRef CAS PubMed.
- M. Tonouchi, Nat. Photonics, 2007, 1, 97–105 CrossRef CAS.
- A. Shalit, S. Ahmed, J. Savolainen and P. Hamm, Nat. Chem., 2017, 9, 273–278 CrossRef CAS PubMed.
- D. Zhao, J. M. Skelton, H. Hu, C. La-o-vorakiat, J.-X. Zhu, R. A. Marcus, M.-E. Michel-Beyerle, Y. M. Lam, A. Walsh and E. E. M. Chia, Appl. Phys. Lett., 2017, 111, 201903 CrossRef.
- M. A. Pérez-Osorio, R. L. Milot, M. R. Filip, J. B. Patel, L. M. Herz, M. B. Johnston and F. Giustino, J. Phys. Chem. C, 2015, 119, 25703–25718 CrossRef.
- S. Koenig, D. Lopez-Diaz, J. Antes, F. Boes, R. Henneberger, A. Leuther, A. Tessmann, R. Schmogrow, D. Hillerkuss, R. Palmer, T. Zwick, C. Koos, W. Freude, O. Ambacher, J. Leuthold and I. Kallfass, Nat. Photonics, 2013, 7, 977–981 CrossRef CAS.
- A. Namai, M. Yoshikiyo, K. Yamada, S. Sakurai, T. Goto, T. Yoshida, T. Miyazaki, M. Nakajima, T. Suemoto, H. Tokoro and S. Ohkoshi, Nat. Commun., 2012, 3, 1035 CrossRef PubMed.
- J. Ma, R. Shrestha, J. Adelberg, C.-Y. Yeh, Z. Hossain, E. Knightly, J. M. Jornet and D. M. Mittleman, Nature, 2018, 563, 89–93 CrossRef CAS PubMed.
- S. Dang, O. Amin, B. Shihada and M.-S. Aluini, Nat. Electron., 2020, 3, 20–29 CrossRef.
- L. Atzori, A. Iera and G. Morabito, Computer Networks, 2010, 54, 2787–2805 CrossRef.
- X. Wu, X. Zhu, G.-Q. Wu and W. Ding, IEEE Trans. Knowl. Data Eng., 2014, 26, 97–107 Search PubMed.
- D. Maurya, A. Charkhesht, S. K. Nayak, F.-C. Sun, D. George, A. Pramanick, M.-G. Kang, H.-C. Song, M. M. Alexander, D. Lou, G. A. Khodaparast, S. P. Alpay, N. Q. Vinh and S. Priya, Phys. Rev. B: Condens. Matter Mater. Phys., 2014, 96, 134114 CrossRef.
- S. Yue, H. T. Chorsi, M. Goyal, T. Schumann, R. Yang, T. Xu, B. Deng, S. Stemmer, J. A. Schuller and B. Liao, Phys. Rev. Res., 2019, 1, 033101 CrossRef.
- V. Keppens, D. Mandrus, B. C. Sales, B. C. Chakoumakos, P. Dai, R. Coldea, M. B. Maple, D. A. Gajewski, E. J. Freeman and S. Bennington, Nature, 1998, 395, 876–878 CrossRef CAS.
- M. M. Koza, M. R. Johnson, R. Viennois, H. Mutka, L. Girard and D. Ravot, Nat. Mater., 2008, 7, 805–810 CrossRef CAS PubMed.
- C. P. Berlinguette, A. Dragulescu-Andrasi, A. Sieber, J. R. Galán-Mascarós, H.-U. Güdel, C. Achim and K. R. Dunbar, J. Am. Chem. Soc., 2004, 126, 6222–6223 CrossRef CAS PubMed.
- O. Sato, T. Iyoda, A. Fujishima and K. Hashimoto, Science, 1996, 272, 704–705 CrossRef CAS PubMed.
- D. Li, R. Clérac, O. Roubeau, E. Harté, C. Mathonière, R. Le Bris and S. M. Holmes, J. Am. Chem. Soc., 2008, 130, 252–258 CrossRef CAS PubMed.
- O. Kahn and C. J. Martinez, Science, 1998, 279, 44–48 CrossRef CAS.
- P. Gütlich, A. Hauser and H. Spiering, Angew. Chem., Int. Ed. Engl., 1994, 33, 2024–2054 CrossRef.
- J. A. Real, E. Andrés, M. C. Muñoz, M. Julve, T. Granier, A. Bousseksou and F. Varret, Science, 2002, 268, 265–267 CrossRef PubMed.
- P. Gütlich, Y. Garcia and H. A. Goodwin, Chem. Soc. Rev., 2000, 29, 419–427 RSC.
- S. Ohkoshi, K. Imoto, Y. Tsunobuchi, S. Takano and H. Tokoro, Nat. Chem., 2011, 3, 564–569 CrossRef CAS PubMed.
- M. A. Halcrow, Chem. Soc. Rev., 2011, 40, 4119–4142 RSC.
- R. M. van der Veen, O.-H. Kwon, A. Tissot, A. Hauser and A. H. Zewail, Nat. Chem., 2013, 5, 395–402 CrossRef CAS PubMed.
- R. Bertoni, M. Lorenc, H. Cailleau, A. Tissot, J. Laisney, M.-L. Boillot, L. Stoleriu, A. Stancu, C. Enachescu and E. Collet, Nat. Mater., 2016, 15, 606–610 CrossRef CAS PubMed.
- M. Nihei, H. Tahira, N. Takahashi, Y. Otake, Y. Yamamura, K. Saito and H. Oshio, J. Am. Chem. Soc., 2010, 132, 3553–3560 CrossRef CAS PubMed.
- E. Collet, M.-H. Lemée-Cailleau, M. B.-L. Cointe, H. Cailleau, M. Wulff, T. Luty, S. Koshihara, M. Meyer, L. Toupet, P. Rabille and S. Techert, Science, 2003, 300, 612–615 CrossRef CAS PubMed.
- S. Ohkoshi, H. Tokoro, T. Hozumi, Y. Zhang, K. Hashimoto, C. Mathonière, I. Bord, G. Rombaut, M. Verelst, C. Cartier dit Moulin and F. Villain, J. Am. Chem. Soc., 2006, 128, 270–277 CrossRef CAS PubMed.
- A. C. Felts, A. Slimani, J. M. Cain, M. J. Andrus, A. R. Ahir, K. A. Abboud, M. W. Meisel, K. Boukheddaden and D. R. Talham, J. Am. Chem. Soc., 2018, 140, 5814–5824 CrossRef CAS PubMed.
- S. Ohkoshi, Y. Tsunobuchi, T. Matsuda, K. Hashimoto, A. Namai, F. Hakoe and H. Tokoro, Nat. Chem., 2010, 2, 539–545 CrossRef CAS PubMed.
- E. S. Koumousi, I.-R. Jeon, Q. Gao, P. Dechambenoit, D. N. Woodruff, P. Merzeau, L. Buisson, X. Jia, D. Li, F. Volatron, C. Mathoniere and R. Clerac, J. Am. Chem. Soc., 2014, 136, 15461–15464 CrossRef CAS PubMed.
- M. Arczyński, J. Stanek, B. Sieklucka, K. R. Dunbar and D. Pinkowicz, J. Am. Chem. Soc., 2019, 141, 19067–19077 CrossRef PubMed.
- L. Egan, K. Kamenev, D. Papanikolaou, Y. Takabayashi and S. Margadonna, J. Am. Chem. Soc., 2006, 128, 6034–6035 CrossRef CAS PubMed.
- D. M. Pajerowski, M. J. Andrus, J. E. Gardner, E. S. Knowles, M. W. Meisel and D. R. Talham, J. Am. Chem. Soc., 2010, 132, 4058–4059 CrossRef CAS PubMed.
- Y.-Z. Zhang, P. Ferko, D. Siretanu, R. Ababei, N. P. Rath, M. J. Shaw, R. Clérac, C. Mathonière and S. M. Holmes, J. Am. Chem. Soc., 2014, 136, 16854–16864 CrossRef CAS PubMed.
- M. Irie, S. Kobatake and M. Horichi, Science, 2001, 291, 1769–1772 CrossRef CAS PubMed.
- P. G. Lacroix, R. Clément, K. Nakatani, J. Zyss and I. Ledoux, Science, 1994, 263, 658–660 CrossRef CAS PubMed.
- S. Venkataramani, U. Jana, M. Dommaschk, F. D. Sönnichsen, F. Tuczek and R. Herges, Science, 2011, 331, 445–448 CrossRef CAS PubMed.
- E. Heintze, F. E. Hallak, C. Clauß, A. Rettori, M. G. Pini, F. Totti, M. Dressel and L. Bogani, Nat. Mater., 2013, 12, 202–206 CrossRef CAS PubMed.
- K. Parlinski, Z. Q. Li and Y. Kawazoe, Phys. Rev. Lett., 1997, 78, 4063–4066 CrossRef CAS.
- G. Kresse and J. Hafner, Phys. Rev. B: Condens. Matter Mater. Phys., 1993, 48, 13115–13118 CrossRef CAS PubMed.
- G. Kresse and J. Furthmüller, Phys. Rev. B: Condens. Matter Mater. Phys., 1996, 54, 11169–11186 CrossRef CAS PubMed.
Footnote |
† Electronic supplementary information (ESI) available. CCDC 1945288 and 1945289. For ESI and crystallographic data in CIF or other electronic format see DOI: 10.1039/d0sc02605k |
|
This journal is © The Royal Society of Chemistry 2020 |
Click here to see how this site uses Cookies. View our privacy policy here.