DOI:
10.1039/C9SM01584A
(Paper)
Soft Matter, 2020,
16, 132-141
Preparation of electrospray ALG/PDA–PVP nanocomposites and their application in cancer therapy†
Received
6th August 2019
, Accepted 3rd November 2019
First published on 4th November 2019
Abstract
In this study, sodium alginate (ALG)/poly dopamine (PDA)–polyvinylpyrrolidone (PVP) nanocomposites was synthesized via a one-step electrostatic spraying method. The spinning solution of ALG and dopamine was electrostatically sprayed into an alkaline solution of PVP, calcium chloride and tris buffer (pH = 8.5), in which the gelation of ALG and the polymerization of dopamine could be simultaneously triggered. PDA hence produced possesses a high photothermal conversion efficiency, while the PVP that was facilely conjugated onto the surface of nanocomposites improves the colloidal stability and compatibility of the material. Moreover, the ALG renders the nanocomposite excellent drug (doxorubicine, DOX) loading capacity. Promisingly, the temperature increment during the PTT process could promote the DOX release, thus enhancing its therapeutic effect. The in vitro/in vivo biosafety and tumor treatment experiments further corroborate that the ALG/PDA–PVP nanocomposites have remarkable biocompatibility and synergism for tumor hyperthermia and chemotherapy. Consequently, such a one-step electrospray strategy provides a new way for designing nanomaterials and is expected to significantly promote the development of organic photothermal therapeutic agents with excellent bio-compatibility.
Introduction
Cancer claims myriads of human life every year, so the exploration for its cure never ceases.1 However, conventional treatments such as surgery and chemotherapy still could not be disentangled from side effects, such as normal tissue lesions and drug resistance.2–4 Compared with other treatments, photothermal therapy (PTT) of tumor has emerged as a new focal point with the advantages of lower toxicity, non-invasiveness, specific targeting and less drug resistance.5,6 In PTT, materials could convert the photon energy of near infrared (NIR) laser into heat, so as to cause ablation and apoptosis of tumor cells.7 For decades, researchers have devoted to making suitable and efficient photothermal agents (PTAs).8–10 Presently, inorganic materials, including gold nanostructures, carbon-based nanomaterials, and two-dimensional transition metal sulfide nanomaterials, have been sought owing to their excellent photothermal conversion properties.11–14 Nonetheless, the long-term accumulation of these materials in organisms would lead to potential biotoxicity because of their poor biodegradability, which fundamentally constricts their translational applications.15 Moreover, organic photothermal materials such as polypyrrole,16 indocyanine green,17 polydopamine (PDA)18 and polythiophene,19 are becoming burgeoning stars as PTAs due to their excellent biocompatibility. However, the over-complicated synthesis process and poor water-solubility remain unresolved and restrict their application in tumor therapy.20,21 Therefore, novel PTAs with convenient synthesis, admirable colloidal stability, higher photothermal conversion efficiency and photothermal durability are yet to be explored.
Electrospinning has attracted increasing attention due to its characteristics such as user-friendly, controllable process and low cost, and has become one of the primary methods to obtain organic/inorganic nanomaterials.22–24 The aforementioned characteristics endow the electrospinning process with broad range of applications in fields of energy storage,25 sensor26 and drug delivery.27 In principle, electrospinning employs the high voltage electrostatic field between the ejecting unit and the receiving device to evaporate and tangle the spinning solution, after which the target nanomaterial is formed on the collecting device.28,29 In addition, by altering the electrospinning parameters (applied voltage, collecting distance between the solution and collecting device, etc.) and adjusting the type and topological structure to the receiving device, different formulations of material, for instance, electrospun fibers, bead fibers, and nanospheres, could be obtained.30–32
Sodium alginate (ALG) is a water-soluble linear polysaccharide, which is composed of α-1,4-L-guluronic acid and β-1,4-D-mannuronic acid.32 As a natural macromolecular material, ALG exhibits excellent biocompatibility and biodegradability.33,34 Besides, the enormous carboxyl groups in alginate enable it to easily cross-link with metallic cations (Ca2+, Fe3+, Ba2+, etc.) to form a hydrogel with an “egg box” structure.35,36 Furthermore, its adequate availability and low immunogenicity further promise a bright prospect in biomedical utility.37 As an organic PTA, PDA displays a remarkable near-infrared absorption, and possesses higher photothermal conversion efficiency than common inorganic metal photothermal materials. Moreover, PDA shows noteworthy biosafety since it is the natural component of human melanin pigment, which makes it particularly desirable for tumor PTT.38–40
In this study, the ALG/PDA–polyvinylpyrrolidone (PVP) nanocomposites were synthesized via a one-step electrostatic spraying method. Spinning solution, containing ALG and dopamine, was first added into a syringe, and then sprayed at a constant speed by the injection pump into the electrostatic field to form a jet. A coagulation bath containing PVP, CaCl2·2H2O and tris(hydroxymethyl) aminomethane (Tris, as the pH conditioner, pH = 8.5) was selected as the receiving device. Hence, dopamine could be polymerized under the alkaline condition, while ALG could cross-link with Ca2+ to form a gel in the meantime. In addition, PVP molecules were simultaneously conjugated onto the surface of nanocomposites to improve the colloidal stability and compatibility of the material. More importantly, the anticancer drug doxorubicin (DOX) could also be added to the spinning solution to simultaneously form ALG/PDA/DOX–PVP nanocomposites for combined tumor PTT and chemotherapy. The feasibility of ALG/PDA/DOX–PVP nanocomposites acting as a PTA for cancer treatment was validated through multiple assays.
Experimental section
Materials
ALG, dopamine hydrochloride, calcium chloride dihydrate (CaCl2·2H2O), and tris were purchased from Aladdin Reagent Co., Ltd (China). PVP (M.W. = 40 kDa) was obtained from Sigma-Aldrich (USA). All reagents were directly used without further processing. Human colon cancer cells (HT29) were bought from the Institute of Biochemistry and Cell Biology, Chinese Academy of Sciences (Shanghai, China). Dulbecco's Modified Eagle Medium (DMEM), fetal bovine serum (FBS), penicillin, streptomycin and phosphate buffer (PBS) were obtained from Gibco (Shanghai, China). Cell counting kit-8 (CCK-8) was purchased from Dojindo Laboratories (Japan). Cell culture flasks and Petri dishes were supplied by Corning Co., Ltd (Shanghai). Balb/c nude mice and Kunming (KM) mice weighing approximately 20 g were purchased from Shanghai Slac Laboratory Animal Center (Shanghai, China). All animal breeding and experiments were conducted at Changhai Hospital, Second Military Medical University Affiliated Hospital, and were in accordance with the agreements and policies of the Ministry of Health. In this study, deionized water with a resistivity higher than 18.2 MΩ cm was purified in a Pall Cascada laboratory water system.
Preparation of ALG/PDA–PVP nanocomposites
A 1% ALG solution was prepared by dissolving 1 g ALG in 99 mL distilled water. Then, dopamine hydrochloride was added to the above solution to obtain a homogenous solution of ALG/DA (m/m = 1
:
2). Two grams of CaCl2·2H2O, 0.5 g Tris, and 0.5 g PVP were blended together in 100 mL distilled water to form a coagulation bath, which acted as the receiving unit. The ALG/dopamine hydrochloride solution was sprayed into the coagulation bath to obtain the ALG/PDA–PVP nanocomposites by electrostatic spraying. The spinning solution was packed into a 5 mL syringe with 22 G right-angled stainless steel needle. The parameters of electrostatic spraying were as follows: the voltage of the needle tip was fixed at 20 kV; the collecting distance from the needle to the coagulation bath was 20 cm; the injection velocity of electrostatic spraying was at 0.5 mL h−1, which was controlled using a home-made syringe pump. The relative humidity and temperature of ambient was 70–75% and 25 °C. The obtained ALG/PDA–PVP was then rinsed using water thrice by centrifugation.
Characterization of the nanocomposites
The morphology and size of the as-prepared ALG/PDA–PVP nanocomposites were observed and measured using a scanning electron microscope (SEM). The measurement of hydrodynamic size and stability were accomplished by dynamic light scattering (DLS, Nano ZS 90, Malvern). To further study the colloidal stability, ALG/PDA/DOX–PVP nanocomposites was dispersed in a mice serum (the blood cells were centrifugally separated (3000 rpm, 3 min)). The structure of the ALG/PDA–PVP nanocomposites was analyzed by the transmission mode of FTIR (Nicolet Nexus 670), ranging from 4000 cm−1 to 500 cm−1 at the scanning speed of 32 scans per min.
In vitro photothermal conversion performance of ALG/PDA–PVP nanocomposites
The absorbance characteristics of the as-prepared ALG/PDA–PVP nanocomposites from 500 to 1000 nm were read by UV-vis-NIR spectrophotometry (Lambda 25, PerkinElmer, USA) at a fixed scanning speed. To assess the in vitro photothermal conversion performance, 100 μL of the sample was distributed in a 96-well cell culture plate for the continuous irradiation of an NIR laser (808 nm, 5 min). The effects of material concentration (2.5, 5.0, 10, and 20 mg mL−1) and power density (0.6, 0.8, and 1.0 W cm−2) on the temperature rise (ΔT) were then studied. The ALG/PDA–PVP nanocomposites (20 mg mL−1) were repeatedly rayed (1 W cm−2, 808 nm, 5 min), then freely cooled (5 min), and the material's photo-to-heat conversion efficiency was calculated according to the method in our previous study.41,42 The photothermal stability of ALG/PDA–PVP nanocomposites was investigated by subjecting the sample to 8 cycles (irradiation: 5 min; cooling: for 5 min) of irradiation (808 nm, 1 W cm−2), with a control group using only pure water. The NIR-laser used in this test was launched from a high-power multimode pump obtained from Shanghai Connet Fiber Optics Company. The sample temperature increment and infrared thermographs were recorded using an FLIRTM E60 infrared camera (FLIR, USA).
Cyto-compatibility of ALG/PDA–PVP nanocomposites
HT29 cells were selected to model the in vitro biocompatibility of ALG/PDA–PVP nanocomposites. Cells were cultured in DMEM (containing 10.0% FBS, 100 unit per mL penicillin, and 0.1 mg mL−1 streptomycin) in a humidifying incubator (37 °C, 5% CO2). The cells prepared as above were planted in a 96-well plate (8 × 103 cells per well) before a 24 hour culture (n = 3). Then, DMEM solution of the material at different concentrations (0, 2.5, 5, and 10 mg mL−1) were dropped into the holes and cultured for another whole day. The metabolism of the treated cells was quantitatively studied using a CCK-8 kit. Further, the cells stained using a live/dead kit and rinsed with PBS solution, were qualitatively studied for morphology using a phase contrast microscope (Leica DM IL LED).
Hemo-compatibility of ALG/PDA–PVP nanocomposites
The fresh blood from KM mice was exacted for the hemo-compatibility of ALG/PDA–PVP nanocomposites. The procedure is as follows: first, a KM mouse was anesthetized before its blood was taken via cardiac puncture. Then, the fresh blood was centrifuged (3000 rpm, 3 min), rinsed three times with PBS for collecting mouse red blood cells (mRBCs). The collected cells were dispersed in PBS and stored in a refrigerator at 4 °C. 0.4 mL mRBC dispersion was mixed with 1.2 mL ALG/PDA–PVP nanocomposites at different concentrations (final concentration at 1, 2.5, 5, and 10 mg mL−1), with water and PBS respectively set as the positive and negative control (n = 3). The above solutions then hatched for 2 h under human body temperature were centrifuged and photographed after the whole culture period. The supernatant was taken out, and their absorbance under the wavelength of 570 nm was quantified using a UV-vis-NIR spectrophotometer (Lambda 25, PerkinElmer, USA). The hemolysis percentage (HP, %) of ALG/PDA–PVP nanocomposites was calculated by the following formula, in which the specific meaning of each symbol was the absorbance of supernatants from the culture vials at 570 nm (ALG/PDA–PVP: Dt, PBS: Dnc, and water: Dpc), respectively:
In vitro drug loading and release
On the pharmaceutical carrying study, a certain amount of DOX was dissolved in the spinning solution (the final concentration at 1 mg mL−1) to obtain the ALG/PDA/DOX–PVP nanocomposites using the same spraying conditions as those of the ALG/PDA–PVP preparation. The as-prepared sample was centrifuged (10
000 rpm, 10 min) and rinsed three times with water. The supernatant was collected, while the sediment was refrigerated for further use. The standard curve of DOX was measured and plotted via UV-vis-NIR spectroscopy (Lambda 25, PerkinElmer, USA). Then, the loading efficiency was calculated with the collected solutions’ absorbance at 480 nm.
The appraisal of the drug release was done as follows: 1 mL ALG/PDA/DOX–PVP (5 mg mL−1) was added inside a dialysis bag with a MWCO of 10
000 kDa, and placed in a vial of PBS (5 mL, pH = 7.4) and HAc-NaAc (5 mL, pH = 6.0). The vial was placed on a constant temperature shaker at different temperatures (37 °C, 54 °C). At the set time, 1 mL fresh buffer was substituted for the equivalent buffer that was removed out. Eventually, the extracted solution was measured using a UV-vis-NIR spectrophotometer for its absorbance at 480 nm and the cumulative release efficiency of DOX was calculated according to the standard curve.
Combined in vitro tumor therapy
For the in vitro tumor therapy test, a 96-well plate was seeded with HT29 cells (8 × 103 cells per well) in DMEM overnight in an incubator. The ALG/PDA–PVP nanocomposites (2.5, 5, and 10 mg mL−1 in DMEM) were dispersed into the culture holes, and then exposed to the NIR laser (808 nm, 5 min) before another 24 h of cultivation. As for the combined treatment, 5 mg mL−1 ALG/PDA–PVP (Group I) and ALG/PDA/DOX–PVP (Groups II, III) were dropped into each well, while pure DMEM was set as the Group IV (n = 3). After being cultured for 12 h, cells in groups I and II were given 5 min exposure to the NIR laser (808 nm, 1 W cm−2). The viability of all cells above were tested using the CCK-8 kit and the stained cells’ fluorescence (live cells would emit green fluorescence, while the dead ones would be red) were observed under a Leica DM IL LED inverted-phase contrast microscope to identify the material's in vitro photothermal and combined therapeutic effects.
Flow cytometer analysis
HT29 cells with a density of 1 × 105 cells per well were seeded in a 6-well tissue culture plate. After 24 h culture, the medium was replaced with medium containing ALG/PDA/DOX–PVP (5 mg mL−1, n = 3) and cultured for predetermined time periods (2 h and 4 h). Then, the medium was removed and cells were washed using PBS (3 times). Finally, cells were digested and dispersed in 1 mL of PBS. The DOX fluorescence was measured using an ISXmkII flow cytometer (Millipore), which is equipped with a 488/561 nm band-pass filter. For the test, 1 × 104 cells in each sample were counted.
In vivo biocompatibility of ALG/PDA–PVP nanocomposites
In this experiment, each KM mouse was intravenously (I.V.) injected with 0.2 mL (3 mg mL−1) of the nanocomposites, with a control group given PBS by the same means. The mice were recorded periodically for their body weight and then euthanized on the 1st, 7th and 14th day for blood collection via cardiac puncture. The routine blood parameters were determined using a Sysmex XS-800i blood analyzer, which concerned the red blood cells (RBCs), white blood cells (WBCs), hemoglobin (HgB), hematocrit (HCT), mean red blood cell volume (MCV), mean erythrocyte hemoglobin content (MCH), mean red blood cell hemoglobin concentration (MCHC), platelet (PLT), and red blood cell distribution width (RDW). Moreover, the serum biochemical parameters, including total bilirubin (TB), alanine aminotransferase (ALT), aspartate aminotransferase (AST), blood urea (Urea), and serum creatinine (Crea) were obtained by a DxC 800 automated biochemical analyzer. For the histocompatibility test of ALG/PDA–PVP, the main organs of KM mice at different time points were stained by hematoxylin-eosin (H&E) and imaged with a Leica DM IL LED inverted phase contrast microscope.
In vivo distribution of DOX
For the biodistribution of DOX, tumor bearing mice were divided into two groups (n = 3). Mice I.V. injected of 100 μL saline containing ALG/PDA/DOX–PVP nanocomposites were anesthetized on day 1 and 7 and organs (tumor, heart, liver, spleen, lung, and kidney) were obtained. Then, 0.3 g of each organ was grinded with lysis solution (500 μL) using a tissue grinder. The formed solution (200 μL) was mixed with Triton (100 μL) and homogenized in isopropanol (1 mL, containing 0.75 mM HCl) at 37 °C for 12 h. The supernatant was centrifuged (12
000 rpm, 10 min) and analyzed using a Microplate reader (SpectraMax i3, Molecular Devices) to record the DOX fluorescence (λex = 485 nm, λem = 528 nm). The DOX concentration was then calculated according to the standard curve.
In vivo tumor therapy of ALG/PDA–PVP nanocomposites
For the in vivo therapeutic experiment, 100 μL of serum-free DMEM containing about 107 HT29 cells were subcutaneously embedded on the back of each mouse to model a typical tumor. After two weeks’ feeding, tumor nodules of about 1 cm width emerged. Then, these mice were randomly distributed into five groups (n = 4). All injecting materials used in this section were prepared into 5 mg mL−1 solutions. Group I injected with 200 μL PBS (I.V.) was settled as the control. Both groups II and III were dosed with the ALG/PDA/DOX–PVP composite (I.V., 200 μL). The nude mice injected with 200 μL ALG/PDA–PVP nanocomposites (I.V.) were classified as Group IV. As for group V, 20 μL of ALG/PDA/DOX–PVP nanocomposites was injected (I.T.) into the mice. After 12 h, mice in Group I, II, IV, and V were subjected to irradiation of the infrared laser (808 nm, 1 W cm−2, 5 min) on their tumor site. The FLIRTM E60 camera (FLIR, USA) was simultaneously placed aside to monitor the thermal changes and collect photothermal images. As the feeding proceeded, the documentation of the tumor volume and appearance of each mouse were recorded with the help of a vernier caliper and camera to assess the comprehensive effect of the in vivo tumor therapy.
Results and discussions
Synthesis and characterization of ALG/PDA–PVP nanocomposites
The spinning solution was an aqueous solution composed of ALG and dopamine at a certain proportion, while the coagulation bath liquid, which served as the collecting unit, was consisted of CaCl2·2H2O, Tris, and PVP. During the material synthesis, the spinning solution was sprayed into the coagulation bath liquid and the solvent (i.e., water) was evaporated during its travel between the positively charged syringe needle and the negatively charged collecting liquid. When contacting Ca2+, the ALG could be crosslinked to form the hydrogel, and the dopamine simultaneously polymerized under an alkaline coagulation bath solution to form PDA nanoparticles, which was then encapsulated by the ALG hydrogel. PVP, as an amphoteric surfactant, could form a polymer micelle to support the ALG hydrogel and PDA formation. Therefore, PVP was simultaneously conjugated onto the ALG/PDA–PVP nanocomposite surface (Fig. 1a) probably owing to the hydrophobic interactions. Moreover, the ALG endows the nanocomposite with excellent drug loading capacity. DOX could be facilely loaded by simply dissolving DOX into the spinning solution. These DOX molecules are uniformly dispersed in the formed ALG/PDA/DOX–PVP nanocomposites upon its formation.
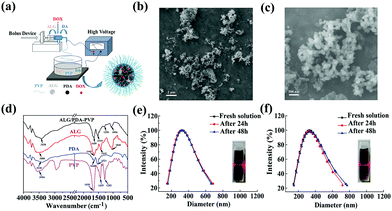 |
| Fig. 1 (a) Schematic of the synthesis process; (b) SEM micrograph of ALG/PDA–PVP nanocomposites; (c) enlarged SEM micrograph of ALG/PDA–PVP nanocomposites; (d) FTIR spectrum of ALG/PDA–PVP, ALG, PDA and PVP; (e and f) DLS and typical Tyndall effect image of ALG/PDA–PVP nanocomposites dispersed in (e) water and (f) saline. | |
As shown in Fig. 1b and c, the formed ALG/PDA–PVP nanocomposites are characterized with regular spherical morphology with an average particle size of about 200 nm. Moreover, it was found that the solution feeding rate exerts a great influence on the morphology. As shown in Fig. S1 (ESI†), irregular fragments were formed when the feeding rate was set as 0.8 mL h−1. Although spherical nanoparticles can also be produced at the lower feeding rate (0.3 mL h−1), its low yield restricts further applications. In order to verify that the as-prepared nanocomposites are composed of ALG, PDA and PVP, all the components above were characterized by FTIR spectroscopy. As shown in Fig. 1d, the peak at about 3500 cm−1 is attributed to the N–H/O–H stretching vibration, which was also found in the spectrum of ALG, PDA, and PVP. The peak at around 1650 cm−1 is the C
O stretching vibration of both ALG and PVP, while the signal at around 1520 cm−1 is the N–H bending vibration of PDA. The peak at around 1290 cm−1 is ascribed to the C–N stretching vibration, while the absorption at about 1050 cm−1 can be attributed to the C–O–C stretching vibration of ALG, indicating the existence of ALG, PDA and PVP in ALG/PDA–PVP nanocomposites. Notably, the ALG/PDA–PVP nanocomposites show a good colloidal stability owing to the modification of PVP. DLS study manifested that the hydrodynamic size of ALG/PDA–PVP scarcely varied after 48 h in water and saline. The colloidal stability of ALG/PDA/DOX–PVP nanocomposites was further studied in mice serum, which showed no detectable precipitate after standing for 24 h (Fig. S2, ESI†). Besides, it also exhibited the typical Tyndall effect in the above solutions (Fig. 1e and f), clearly confirming the long-term stability and laying the foundation for subsequent tumor treatments of the electrospray ALG/PDA–PVP nanocomposites.
Photothermal conversion performance of ALG/PDA–PVP nanocomposites
Ahead of the photothermal conversion experiment, UV-vis-NIR spectroscopy was used to test the absorbance of the ALG/PDA–PVP nanocomposites at the wavelength range from 500 nm to 1000 nm, which clearly confirmed its concentration-dependent light absorption in the NIR region (Fig. S3, ESI†). The 808 nm NIR laser irradiation was then utilized to study the photothermal transition of the material. As shown in Fig. 2a, when irradiated the solution (with concentrations of 20, 10, 5.0, and 2.5 mg mL−1), the temperature increments (ΔT) were 42 °C, 30 °C, 18 °C, and 11 °C, respectively. However, water was only heated for 2 °C. When laser with different power densities (1.0, 0.8, and 0.6 W cm−2) were beamed onto the material, the ΔTs were calculated as 18.6 °C, 12.5 °C, and 10.5 °C, respectively (Fig. 2c). Except for the heating curve, the material concentration and laser density dependent temperature increase was further demonstrated by the infrared thermal imaging (Fig. 2b and d).
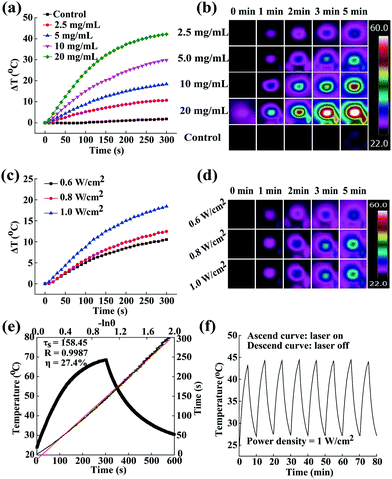 |
| Fig. 2 (a) Concentration-dependent temperature curve of ALG/PDA–PVP nanocomposites under 808 nm laser irradiation at a power density of 1.0 W cm−2; (b) corresponding thermal images of (a); (c) temperature curves with different power densities (concentration: 5 mg mL−1); (d) corresponding thermal images of (c); (e) η value and time constant for heat transfer of ALG/PDA–PVP nanocomposites; (f) temperature rise curve under eight laser irradiation cycles. | |
The time constant of the photothermal conversion process was calculated at 158.45 (Fig. 2e). Therefore, the photothermal conversion efficiency of the ALG/PDA–PVP nanocomposites is reckoned as 27.4%. Besides, the ALG/PDA–PVP nanocomposites display good photothermal stability with no significant ΔT retrogress during 8 irradiation cycles (Fig. 2f, laser on: 5 min, laser off: 5 min), verifying that the ALG/PDA–PVP nanocomposites could be used as a highly efficient photothermal reagent for cancer hyperthermia.
In vitro cyto-compatibility
Previous to cancer treating applications, it is essential to validate whether the ALG/PDA–PVP nanocomposites as a foreign species would bring biological toxicity to the organism. Therefore, its bio-compatibility was studied in vitro and in vivo. HT29 cells were used for the detailed in vitro judgement of the biocompatibility of the material on the basis of cellular viability and morphology. After being cultured with ALG/PDA–PVP nanocomposites for 24 h, the cellular survival rates of all three groups were above 95% (concentration of 2.5 mg mL−1: 99.3%, and 5 mg mL−1: 98.6%, Fig. 3a) and showed paltry cell death even after treated with high concentration of material (10 mg mL−1, 99.5%) as compared to cells incubated with PBS (control, the survival rate of which was set as 100%). The live/dead staining morphology observation of cells further suggests that the cell membrane remained intact and the treated cells could be hardly stained into red (Fig. 3c), which obviously corroborates that the ALG/PDA–PVP nanocomposites have unparalleled in vitro biocompatibility at experimental concentrations.
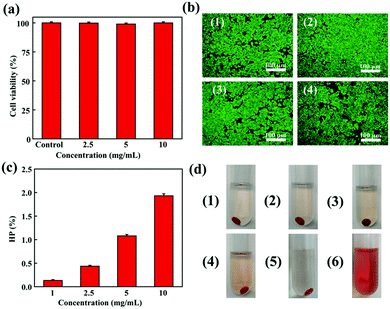 |
| Fig. 3 (a and b) Survival rates and corresponding live/dead morphology of HT29 cells cultured with ALG/PDA–PVP nanocomposites at different concentrations (b: (1): control, (2): 2.5 mg mL−1, (3): 5 mg mL−1, (4): 10 mg mL−1); (c and d) HP and photos of centrifuged mRBCs incubated with ALG/PDA–PVP nanocomposites with concentrations of (1) 1 mg mL−1, (2) 2.5 mg mL−1, (3) 5 mg mL−1, (4) 10 mg mL−1, (5) PBS, and (6) distilled water (n = 3). | |
In vitro hemo-compatibility examination
As another prerequisite of clinical application, the hemo-compatibility of the ALG/PDA–PVP nanocomposites was assessed by conducting a hemolysis experiment. In the in vitro hemolytic assay, blood cells cultured with water and PBS were set as positive and negative controls, whose hemolysis rates were 100% and 0% by default. The mRBCs were treated with ALG/PDA–PVP nanocomposites at different concentrations for 2 h at a constant temperature, of which the HPs could be calculated as 0.13%, 0.43%, 1.08% and 1.93% (material concentrations at 1.0, 2.5, 5.0, and 10 mg mL−1, respectively, Fig. 3c). The HPs were all less than 5%, which authenticated the excellent hemo-compatibility of the ALG/PDA–PVP nanocomposites. It was potentially accepted that because of the non-specific adsorption of plasma proteins onto the surface of the guest material, a small number of mRBC cellular membranes were impaired (HP: 1.08% and 1.93%, material concentrations at 5.0 and 10 mg mL−1, respectively, Fig. 3b). Moreover, the mRBCs dissolved in distilled water were completely destroyed, forming a red solution of their contents, while those cultured with PBS and ALG/PDA–PVP nanocomposites still maintained their structural integrity with trifling damage (Fig. 3d), further affirming that the ALG/PDA–PVP nanocomposites have perfect blood compatibility within the experimental dosages. Besides, no aggregation was found during the 3 h culture of nanocomposites and mRBCs (data not shown), clearly indicating its blood cell stability.
Drug loading and release
Typically, chemotherapeutic drugs encapsulated in nanomaterials take endocytosis as an entry pathway to give out their targeted therapeutic efficacy.43 Hence, the feasibility of utilizing ALG/PDA–PVP nanocomposites as nano-carriers for drug was investigated. The water-soluble anticancer drug DOX was dissolved in the spinning solution (1 mg mL−1) and selected as a drug model, which was encapsulated in the cross-linked ALG/PDA–PVP to simultaneously form ALG/PDA/DOX–PVP nanocomposites. The loading efficiency of the obtained DOX-loaded material was approximately 84.3% (Fig. S4, ESI†). This high drug loading efficiency was further qualitatively confirmed by observing the red color (the color of DOX solution) of centrifuged coagulation bath solution (Fig. S5, ESI†), which clearly indicating the dramatic color fading after the drug loading. Such a high drug loading efficiency can be explained as the sufficient drug package during the Ca2+ induced swift formation of ALG nanocomposites. The cumulative release rates of DOX were 23.5%, 27.7%, and 29.9% at the corresponding conditions of pH = 7.4/T = 37 °C, pH = 6.0/T = 54 °C and pH = 6.0/T = 54 °C (Fig. 4a). The drug release may be attributed to the diffusion of DOX from the composite to the surroundings. Notably, high temperature and low pH could accelerate the drug release, which might be attributed to the increased solubility of DOX under rising temperature and falling pH. Considering the acidic tumor microenvironment as well as the PTT of the as-prepared nanocomposites, the designed nanocomposites with sustainable drug release are anticipated to facilitate the drug release in the tumor tissue, thus improving the outcome of tumor chemotherapy.
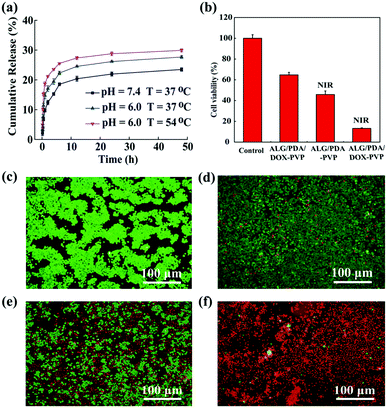 |
| Fig. 4 (a) In vitro drug cumulative release profiles of DOX from ALG/PDA/DOX–PVP nanocomposites; (b) combined tumor therapy of ALG/PDA–PVP and ALG/PDA/DOX–PVP nanocomposites (5 mg mL−1); (c–f) morphology of live/dead staining of HT29 cells corresponding to (b): ((c): control, (d) ALG/PDA/DOX–PVP, (e) ALG/PDA–PVP + NIR, (f) ALG/PDA/DOX–PVP + NIR). | |
In vitro PTT and combined tumor therapy
Previous examinations had demonstrated that ALG/PDA–PVP nanocomposites possess excellent photothermal conversion property and controllable drug release capability; thereafter, the tumor treatment study was performed in vitro and in vivo. Live/dead staining and CCK-8 were used to assess the cell viability to estimate the in vitro tumor PTT effect. After being cultured with different concentrations of ALG/PDA–PVP nanocomposites, HT29 cells were subjected to constant irradiation of NIR laser simultaneously, which show a decrease in the cellular activity as the concentration increased (2.5 mg mL−1: 93.3%; 5.0 mg mL−1: 46.4%, Fig. S6, ESI†). Moreover, when the material concentration reached 10 mg mL−1, the cell survival rate dropped to approximately 20% (10 mg mL−1: 23.8%, Fig. S6, ESI†). In addition, according to the live/dead staining, the amount of red cells was proportional to the material concentration, further ascertaining that ALG/PDA–PVP nanocomposites possess desirable inhibitory effect on cellular proliferation (Fig. S7, ESI†).
Nanocarriers loaded with chemotherapeutic drug can enter the cells by endocytosis of the cells, and then release the loaded drug upon the nanocarrier decomposition and dissolution.41 To further explore the therapeutic efficiency, the combined in vitro photothermal/chemotherapy efficacy of ALG/PDA/DOX–PVP nanocomposites was investigated. As shown in Fig. 4b, compared to the PBS-cultured cells, those cultured with ALG/PDA/DOX–PVP resulted in a significant decrease in viability (64.7%) due to the chemotherapeutic effect of DOX. The proliferation of cells cultured with ALG/PDA–PVP nanocomposites was suppressed by laser irradiation, and only 45.8% cells survived. The cell activity decreased to only 13.2% in the combined therapy group owing to the hyperthermia and the released DOX-caused cell killing. It was also anticipated that the photothermal conversion of ALG/PDA–PVP nanocomposites could elevate the efficacy of chemotherapy via promoting the DOX release (Fig. 4a). In addition, qualitative calcein-AM/PI live/dead staining pictures clearly demonstrate the tumor PTT, chemotherapy, and combined tumor therapy of ALG/PDA–PVP, ALG/PDA/DOX–PVP and ALG/PDA/DOX–PVP (with NIR irradiation), respectively (Fig. 4c–f).
Cellular uptake of ALG/PDA/DOX–PVP nanocomposites
To quantify the cellular uptake of ALG/PDA/DOX–PVP, flow cytometry of HT29 cells was performed after incubation with nanocomposites for 2 or 4 h (Fig. 5). Apparently, the treatment of ALG/PDA/DOX–PVP nanocomposites showed an apparent increase in the fluorescence signal of cells than control (Fig. 5a–c), proving an efficient cellular uptake of ALG/PDA/DOX–PVP nanocomposites. Moreover, the fluorescence intensity at 4 h was higher than that at 2 h (Fig. 5d), indicating the incubation time-dependent cellular uptake of ALG/PDA/DOX–PVP. The flow cytometry data quantitatively verify that ALG/PDA/DOX–PVP nanocomposites can efficiently deliver DOX to cancer cells, which will then significantly improve its tumor therapeutic efficacy.
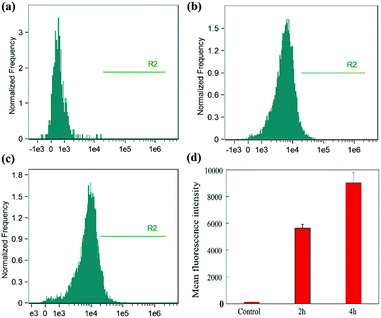 |
| Fig. 5 Flow cytometer analysis of the in vitro cellular uptake of (a) PBS and (b and c) ALG/PDA/DOX–PVP in HT29 cells after (b) 2 h and (c) 4 h incubation; (d) the comparison of the mean fluorescence intensity of HT29 cells after 2 h and 4 h treatment with ALG/PDA/DOX–PVP. | |
In vivo biocompatibility assay
The in vivo biocompatibility of the ALG/PDA–PVP nanocomposites was verified via blood routine detection, serum biochemical analysis, and tissue section staining, before the in vivo tumor therapy studies. The long-term biosafety of the material was first assessed by weighing the mice at due time points. During continuous feeding, the KM mice exhibited a minimal body weight fluctuation in 14 days (Fig. 6a). The routine blood test and serum biochemical analysis on mice blood further suggest that neither the blood routine tests (RBC, WBC, HGB, HCT, MCV, MCH, MCHC, PLT, RDW) nor the biochemical parameters (TB, ALT, AST, Urea, Crea) showed meaningful distinctions when compared with the PBS-treated mice, evidencing the excellent in vivo blood compatibility of the ALG/PDA–PVP nanocomposites (Fig. S8 (ESI†) and Fig. 6b, c). Further in vivo biocompatibility investigation was performed with H&E staining on the sliced organ tissues to determine the lesions on main organs (Fig. 6d). The pictures show that ALG/PDA–PVP nanocomposites barely have any negative effect or pathological abnormality on the organisms in the 14 day feeding, promising the enormous in vivo tumor therapy potential of ALG/PDA–PVP nanocomposites.
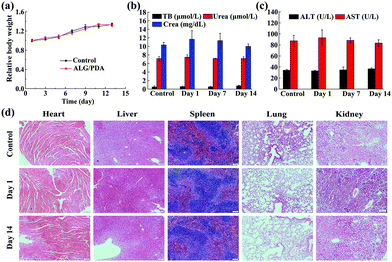 |
| Fig. 6 (a) Time-dependent body weight evolution of KM mice; (b and c) blood biochemistry parameters of KM mice, and (d) H&E staining images of the major organs heart, liver, spleen, lung, and kidney of KM mice treated with PBS and ALG/PDA–PVP. | |
In vivo distribution of DOX
The in vivo biodistribution of DOX was confirmed by in vivo biodistribution analysis on day 1 and day 7. As shown in Fig. S9 (ESI†), the amount of DOX showes no obvious change in major organs and tumor during the first 7 days. On day 1, the distribution ratios of DOX were 2.3 ± 1.4% in heart, 15.9 ± 0.9% in liver, 6.6 ± 0.2% in spleen, 4.3 ± 1.1% in lung, 2.9 ± 0.9% in kidney and 0.9 ± 0.4% in tumor. Their values changed to 1.7 ± 0.9%, 12.3 ± 1.4%, 4.9 ± 0.7%, 5.6 ± 0.5%, 2.6 ± 1.9% and 1.2 ± 0.7%, respectively on day 7. It is worth noting that the enhanced permeability and retention effect induced DOX accumulation in tumor was low in the experimental time periods; however, it could be enhanced by linking the tumor targeting ligands in future studies.
Combined in vivo tumor therapy
After qualifying the excellency of ALG/PDA–PVP nanocomposites in photothermal conversion efficiency, biosafety and in vitro tumor cell treatment efficiency, the superiority of ALG/PDA–PVP nanocomposites in in vivo tumor treatment was studied. The results of in vivo photothermal efficiency indicate that the I.V. injected tumor temperature increased by 10.8 °C (ALG/PDA–PVP) and 10.5 °C (ALG/PDA/DOX–PVP) after 5 min of NIR irradiation. The I.T. injected mice with NIR irradiation were observed with a temperature increment of 17.3 °C after 5 min laser irradiation, significantly higher than other groups (Fig. 7a). Infrared images of these mice further confirm the variation in tumor temperature (Fig. 7b). The changes in tumor volume and appearance under different modes of administration were recorded (Fig. 7c). Compared with the control group whose tumors expanded to nearly 9.9 times of their original sizes, tumor volumes of other groups showed a difference in growth after 28 days of feeding. Mere photothermal therapy or chemotherapy could not completely inhibit the growth of the malignant lumps, which are 6.5 and 4.8 times larger than the initial states. Owing to the promotion of drug release via temperature increase, the combination-effect of PTT and chemotherapy manifests an appreciable inhibition of tumor growth. Obviously, the therapeutic effect of the I.V. injection was significantly lower than that of the I.T. injection due to the limited accumulation and blood dilution of drugs and the tumor volume of the former group is 2.1 times higher than that of the initial state, while the latter group was evidently suppressed. The pictures of the tumor appearance further confirm the effect of the material in cancer treatment (Fig. 7d).
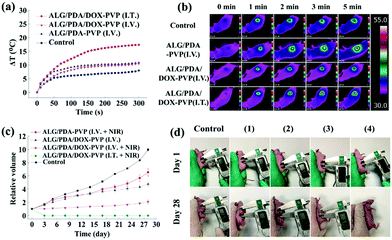 |
| Fig. 7 (a and b) The tumor heating curves and in vivo thermal images of mice received various treatments (as noted) and NIR laser irradiation, respectively; (c) tumor growth profile of mice after various treatments as noted; (d) representative pictures of tumor bearing mice corresponding to (c): (1) ALG/PDA–PVP (I.V. + NIR), (2) ALG/PDA/DOX–PVP (I.V.), (3) ALG/PDA/DOX–PVP (I.V. + NIR), (4) ALG/PDA/DOX–PVP (I.T. + NIR). | |
Conclusions
In summary, PVP-modified and DOX-loaded nanocomposites of ALG and PDA were synthesized via a one-step electrostatic spraying method. After being electrostatically sprayed into the tris buffer solution containing PVP and CaCl2, ALG/dopamine solution underwent a simultaneous gelation and polymerization. It was found that the as-prepared nanocomposites possess excellent photothermal conversion capability with the photothermal conversion efficiency of 27.4%. Moreover, via directly dissolving DOX into the ALG/dopamine solution, ALG/PDA/DOX–PVP nanocomposites with temperature and pH dual modal controllable drug release capability could also be facilely prepared. The in vitro spectroscopic characteristic, controlled drug release pattern and anti-tumor therapeutic performance of the as-prepared ALG/PDA–PVP nanocomposites were investigated. It was concluded that the ALG/PDA–PVP nanocomposites suggest its superiority in oncotherapy as a nano-platform for both hyperthermia therapy and chemotherapy. Findings in this study provide new thoughts for the synthesis of promising multi-modal tumor therapeutic agents.
Conflicts of interest
There are no conflicts to declare.
Acknowledgements
This work was financially supported by the National Science Foundation of China (Grant No. 51702214) and Youth Foundation of Shanghai Municipal Commission of Health and Family Planning (Grant No. 20184Y0214).
Notes and references
- X. Gong, Y. Zheng, G. He, K. Chen, X. Zeng and Z. Chen, Multifunctional nanoplatform based on star-shaped copolymer for liver cancer targeting therapy, Drug Delivery, 2019, 26, 595–603 CrossRef CAS.
- Y. Y. Liu, X. F. Meng and W. B. Bu, Upconversion-based photodynamic cancer therapy, Coord. Chem. Rev., 2019, 379, 82–98 CrossRef CAS.
- L. S. Lin, T. Huang, J. Song, X. Ou, Z. Wang and H. Deng,
et al., Synthesis of copper peroxide nanodots for H2O2 self-supplying chemodynamic therapy, J. Am. Chem. Soc., 2019, 141, 9937–9945 CrossRef CAS.
- T. Feng, J. Wan, P. Li, H. Ran, H. Chen and Z. Wang,
et al., A novel NIR-controlled NO release of sodium nitroprusside-doped Prussian blue nanoparticle for synergistic tumor treatment, Biomaterials, 2019, 214, 119213 CrossRef CAS.
- C. E. Shi, C. Q. You and L. Pan, Facile formulation of near-infrared light-triggered hollow mesoporous silica nanoparticles based on mitochondria targeting for on-demand chemo/photothermal/photodynamic therapy, Nanotechnology, 2019, 30, 325102 CrossRef CAS.
- Z. Guo, S. Zhu, Y. Yong, X. Zhang, X. H. Dong and J. F. Du,
et al., Synthesis of BSA-coated BiOI@Bi2S3 semiconductor heterojunction nanoparticles and their applications for radio/photodynamic/photothermal synergistic therapy of tumor, Adv. Mater., 2017, 29, 1704136 CrossRef.
- L. Zhang, Y. Zhang, Y. N. Xue, Y. Wu, Q. Q. Wang and L. J. Xue,
et al., Transforming weakness into strength: photothermal-therapy-induced inflammation enhanced cytopharmaceutical chemotherapy as a combination anticancer treatment, Adv. Mater., 2019, 31, 1805936 Search PubMed.
- J. Zhao, A. H. Wang, T. Y. Si, O. D. Hong and J. B. Li, Gold nanorods based multicompartment mesoporous silica composites as bioagents for highly efficient photothermal therapy, J. Colloid Interface Sci., 2019, 549, 9–15 CrossRef CAS.
- Q. Q. Yu, Y. M. Han, X. C. Wang, C. Qin, D. Zhai and Z. F. Yi,
et al., Copper silicate hollow microspheres-incorporated scaffolds for chemo-photothermal therapy of melanoma and tissue healing, ACS Nano, 2018, 12, 12941 CrossRef CAS.
- C. Scialabba, A. Sciortino, F. Messina, G. Buscarino, M. Cannas and G. Roscigno,
et al., Highly homogeneous biotinylated carbon nanodots: red-emitting nanoheaters as theranostic agents toward precision cancer medicine, ACS Appl. Mater. Interfaces, 2019, 11, 19854–19866 CrossRef CAS.
- Y. Y. Yu, M. Zhou, W. Zhang, L. Huang, D. D. Miao and H. Y. Zhu,
et al., Rattle-type gold nanorods/porous-SiO2 nanocomposites as near-infrared light-activated drug delivery systems for cancer combined chemo-photothermal therapy, Mol. Pharmaceutics, 2019, 16, 1929–1938 CrossRef CAS.
- M. Khafaji, M. Zamani, M. Golizadeh and O. Bavi, Inorganic nanomaterials for chemo/photothermal therapy: a promising horizon on effective cancer treatment, Biophys. Rev., 2019, 11, 335–352 CrossRef CAS.
- C. Wu, S. Wang, J. Zhao, Y. Liu, Y. Zheng and Y. Luo,
et al., Biodegradable Fe(III)@WS2-PVP nanocapsules for redox reaction and time-enhanced nanocatalytic, photothermal, and chemotherapy, Adv. Funct. Mater., 2019, 1901722 CrossRef.
- Y. Zheng, W. Wang, J. Zhao, C. Wu, C. Ye and M. Huang,
et al., Preparation of injectable temperature-sensitive chitosan-based hydrogel for combined hyperthermia and chemotherapy of colon cancer, Carbohydr. Polym., 2019, 222, 115039 CrossRef CAS.
- P. Fan, Z. Fan, F. L. Huang, J. T. Yang, F. Chen and Z. D. Fei,
et al., GO@polyaniline nanorod array hierarchical structure: a photothermal agent with high photothermal conversion efficiency for fast near-infrared responsive hydrogels, Ind. Eng. Chem. Res., 2019, 58, 3893–3901 CrossRef CAS.
- Y. X. Chen, S. Y. Xiang, L. Wang, M. Y. Wang, C. C. Wang and S. W. Liu,
et al., Hollow polypyrrole nanospindles for highly effective cancer therapy, ChemPlusChem, 2018, 83, 1127–1134 CrossRef CAS.
- X. R. Liu, C. Wang, H. S. Ma, F. Y. Yu, F. Q. Hu and H. Yuan, Water-responsive hybrid nanoparticles codelivering ICG and DOX effectively treat breast cancer via hyperthermia-aided DOX functionality and drug penetration, Adv. Healthcare Mater., 2019, 8, 1801486 CrossRef.
- Q. L. Lu, S. Qi, P. S. Li, L. F. Yang, S. Yang and Y. D. Wang,
et al., Photothermally activatable PDA immune nanomedicine combined with PD-L1 checkpoint blockade for antimetastatic cancer photoimmunotherapy, J. Mater. Chem. B, 2019, 7, 2499–2511 RSC.
- L. Cheng, K. Yang, Q. Chen and Z. Liu, Organic stealth nanoparticles for highly effective in vivo near-infrared photothermal therapy of cancer, ACS Nano, 2012, 6, 5605–5613 CrossRef CAS.
- J. J. Hu, Y. J. Cheng and X. Z. Zhang, Recent advances in nanomaterials for enhanced photothermal therapy of tumors, Nanoscale, 2018, 10, 22657–22672 RSC.
- K. Q. Qiu, J. Q. Wang, T. W. Rees, L. N. A. Ji, Q. L. Zhang and H. Chao, A mitochondria-targeting photothermogenic nanozyme for MRI-guided mild photothermal therapy, Chem. Commun., 2018, 54, 14108–14111 RSC.
- J. Li, Q. Zhang, S. S. Peng, D. S. Zhang, X. M. Yan and X. M. Wu,
et al., Electrospinning fiberization of carbon nanotube hybrid sulfonated poly(ether ether ketone) ion conductive membranes for a vanadium redox flow battery, J. Membr. Sci., 2019, 583, 93–102 CrossRef CAS.
- D. Li and Y. N. Xia, Electrospinning of nanofibers: reinventing the wheel?, Adv. Mater., 2004, 16, 1151–1170 CrossRef CAS.
- J. Y. Lyu, S. Chen, W. He, X. X. Zhang, D. Y. Tang and P. J. Liu,
et al., Fabrication of high-performance graphene oxide doped PVDF/CuO/Al nanocomposites via electrospinning, Chem. Eng. J., 2019, 368, 129–137 CrossRef CAS.
- S. Wang, D. L. Zhang, Z. Shao and S. Y. Liu, Cellulosic materials-enhanced sandwich structure-like separator via electrospinning towards safer lithium-ion battery, Carbohydr. Polym., 2019, 214, 328–336 CrossRef CAS.
- L. B. Zhao, Y. H. Zhao, Y. Xia, Z. K. Li, J. Li and J. W. Zhang,
et al., A novel cmut-based resonant biochemical sensor using electrospinning technology, IEEE Trans. Ind. Electron., 2019, 66, 7356–7365 Search PubMed.
- E. M. Cagil, O. Hameed and F. Ozcan, Production of a new platform based calixarene nanofiber for controlled release of the drugs, Mater. Sci. Eng., C, 2019, 100, 466–474 CrossRef CAS.
- M. Koosha, M. Raoufi and H. Moravvej, One-pot reactive electrospinning of chitosan/PVA hydrogel nanofibers reinforced by halloysite nanotubes with enhanced fibroblast cell attachment for skin tissue regeneration, Colloids Surf., B, 2019, 179, 270–279 CrossRef CAS.
- P. Aghasiloo, M. Yousefzadeh, M. Latifi and R. Jose, Highly porous TiO2 nanofibers by humid-electrospinning with enhanced photocatalytic properties, J. Alloys Compd., 2019, 790, 257–265 CrossRef CAS.
- M. T. Huang, H. Tu, J. J. Chen, R. Liu, Z. Y. Liang and L. B. Jiang,
et al., Chitosan-rectorite nanospheres embedded aminated polyacrylonitrile nanofibers via shoulder-to-shoulder electrospinning and electrospraying for enhanced heavy metal removal, Appl. Surf. Sci., 2018, 437, 294–303 CrossRef CAS.
- R. Zhang, R. R. Xie, D. P. Liu, X. L. Jia, Q. Cai and X. P. Yang, Nanoporous fibers built with carbon-bound SiO2 nanospheres via electrospinning and calcination, Mater. Des., 2017, 130, 231–238 CrossRef CAS.
- D. Jing and S. Yi, Electroosmotic flow in treelike branching microchannel network, Fractals, 2019, 27, 1950095 CrossRef.
- L. Wang, R. M. Shelton, P. R. Cooper, M. Lawson, J. T. Triffitt and J. E. Barralet, Evaluation of sodium alginate for bone marrow cell tissue engineering, Biomaterials, 2003, 24, 3475–3481 CrossRef CAS.
- M. Xie, F. Zhang, H. P. Peng, Y. N. Zhang, Y. P. Li and Y. G. Xu,
et al., Layer-by-layer modification of magnetic graphene oxide by chitosan and sodium alginate with enhanced dispersibility for targeted drug delivery and photothermal therapy, Colloids Surf., B, 2019, 176, 462–470 CrossRef CAS.
- J. Wu, H. Zheng, F. Zhang, R. J. Zeng and B. S. Xing, Iron-carbon composite from carbonization of iron-crosslinked sodium alginate for Cr(VI) removal, Chem. Eng. J., 2019, 362, 21–29 CrossRef CAS.
- G. R. Mahdavinia, Z. Rahmani, S. Karami and A. Pourjavadi, Magnetic/pH-sensitive kappa-carrageenan/sodium alginate hydrogel nanocomposite beads: preparation, swelling behavior, and drug delivery, J. Biomater. Sci., Polym. Ed., 2014, 25, 1891–1906 CrossRef CAS.
- A. D. Ayub, H. I. Chiu, S. Yusuf, E. Abd Kadir, S. H. Ngalim and V. Lim, Biocompatible disulphide cross-linked sodium alginate derivative nanoparticles for oral colon-targeted drug delivery, Artif. Cells, Nanomed., Biotechnol., 2019, 47, 353–369 CrossRef CAS.
- C. Chen, W. Tang, D. W. Jiang, G. L. Yang, X. L. Wang and L. N. Zhou,
et al., Hyaluronic acid conjugated polydopamine functionalized mesoporous silica nanoparticles for synergistic targeted chemo-photothermal therapy, Nanoscale, 2019, 11, 11012–11024 RSC.
- Y. F. Tian and M. Lei, Polydopamine-based composite nanoparticles with redox-labile polymer shells for controlled drug release and enhanced chemo-photothermal therapy, Nanoscale Res. Lett., 2019, 14, 186 CrossRef.
- Y. X. Xing, T. Ding, Z. Q. Wang, L. C. Wang, H. D. Guan and J. Tang,
et al., Temporally controlled photothermal/photodynamic and combined therapy for overcoming multidrug resistance of cancer by polydopamine nanoclustered micelles, ACS Appl. Mater. Interfaces, 2019, 11, 13945–13953 CrossRef CAS.
- S. Wang, L. Zhou, Y. Zheng, L. Li, C. Wu and H. Yang,
et al., Synthesis and bio-compatibility of two-dimensional biomaterials, Colloids Surf., A, 2019, 583, 124004 CrossRef CAS.
- Y. Liu, Y. Xi, J. Zhao, J. Zhao, J. Li and G. Huang,
et al., Preparation of therapeutic-laden konjac hydrogel for tumor combination therapy, Chem. Eng. J., 2019, 375, 122048 CrossRef CAS.
- V. Francia, C. Reker-Smit, G. Boel and A. Salvati, Limits and challenges in using transport inhibitors to characterize how nano-sized drug carriers enter cells, Nanomedicine, 2019, 14, 1533–1549 CrossRef CAS.
Footnotes |
† Electronic supplementary information (ESI) available. See DOI: 10.1039/c9sm01584a |
‡ Y. X. and J. Z. contributed equally to this work. |
|
This journal is © The Royal Society of Chemistry 2020 |
Click here to see how this site uses Cookies. View our privacy policy here.