DOI:
10.1039/D0CS01150A
(Tutorial Review)
Chem. Soc. Rev., 2021,
50, 87-101
Phosphorus recovery and recycling – closing the loop†
Received
4th September 2020
First published on 19th November 2020
Abstract
There is a clear and pressing need to better manage our planet's resources. Phosphorus is a crucial element for life, but the natural phosphorus cycle has been perturbed to such an extent that humanity faces two dovetailing problems: the dwindling supply of phosphate rock as a resource, and the overabundance of phosphate in water systems leading to eutrophication. This Tutorial Review will explore the current routes to industrial phosphorus compounds, and innovative academic routes towards accessing these same products in a more sustainable manner. It will then describe the many ways that useful phosphate can be recovered from waste streams, and how it can be recycled and used as a resource for new products. Finally, we will briefly discuss the barriers that have thus far prevented the widespread adoption of these technologies, and how we can close the loop to establish a modern phosphorus cycle.
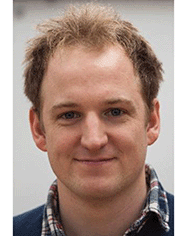
Andrew R. Jupp
| Andrew Jupp obtained his PhD from the University of Oxford (2012–2016) under the supervision of Prof. Jose Goicoechea. He worked on phosphorus analogues of the cyanate anion and urea, for which he was awarded the Reaxys PhD Prize in Hong Kong in 2015. He subsequently carried out a Banting Postdoctoral Fellowship with Prof. Doug Stephan at the University of Toronto (2016–2018), working on the synthesis and reactivity of main-group Lewis acids and bases, and the functionalisation of carbon dioxide. In 2018, he became an NWO VENI laureate at the University of Amsterdam, working with Assoc. Prof. Chris Slootweg on the formation of main-group radicals. In 2020, he launched his independent career as a Birmingham Fellow at the University of Birmingham (UK), working on small-molecule activation and molecular photo-switches. |
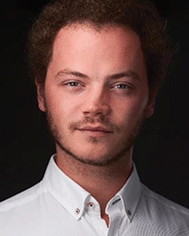
Steven Beijer
| Steven Beijer obtained his BSc in chemistry at Utrecht University and his MSc at the University of Amsterdam with an emphasis on energy and sustainability. His master's thesis on valorizing phosphate from waste streams was awarded the Unilever Research Prize 2019. He is currently a PhD candidate under the supervision of Assoc. Prof. Chris Slootweg. His research focuses on the sustainable use of phosphorus, positioning him at the intersection of developing novel recycling methodologies and exploring their potential for large-scale implementation. |
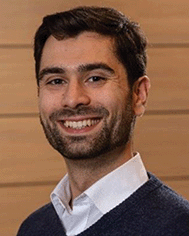
Ganesha C. Narain
| Ganesha (Nesha) Narain obtained his Master's degree in Chemistry from the University of Amsterdam and the Vrije Universiteit Amsterdam. He worked on phosphorus recovery and recycling and molecular sensing. His focus has been sustainability, circular economy and renewable energy. He obtained his Bachelor's degree in Chemical Engineering from the University of Twente focusing on material engineering and a research into photocatalytic water splitting. Nesha is currently pursuing a career as a strategy consultant. He remains a sustainability enthusiast and keeps aiming for societal impact. |
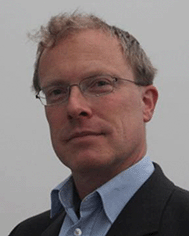
Willem Schipper
| Willem Schipper received his MSc in 1989 and his PhD in 1993, at Utrecht University in physical chemistry under the supervision of Prof. G. Blasse. He subsequently joined Hoechst Holland, which later became Thermphos International, as chemist supporting the industrial production of white phosphorus and phosphates. As Senior Chemist, he pioneered the use of secondary raw materials, such as sewage sludge ash and meat and bone meal ash, for the production of white phosphorus. As Innovation Manager he explored the direct functionalisation of white phosphorus to obtain various phosphorus containing chemicals, bypassing the existing derivatives routes, and developed the synthesis and chemistry of phosphorus trioxide. Since 2013 he works as independent consultant to the industry and the scientific world, focusing on recycling, phosphorus chemistry, and process development. |
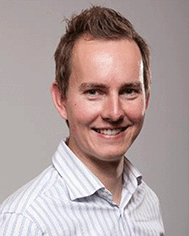
J. Chris Slootweg
| Chris Slootweg was born in Haarlem (The Netherlands) in 1978 and received his undergraduate education from Vrije Universiteit Amsterdam in 2001. After earning his PhD in 2005 under the supervision of Prof. Koop Lammertsma, he pursued postdoctoral studies at the ETH Zürich with Peter Chen. In 2006, he returned to VU to initiate his independent career. He was promoted to Associate Professor in 2014, and moved to the University of Amsterdam in 2016. The mission of his laboratory is to educate students at the intersection of fundamental physical organic chemistry, main-group chemistry, and circular chemistry. |
Key learning points
(1) Why is phosphorus important and how has the natural phosphorus cycle been disrupted?
(2) How are phosphorus-containing compounds currently synthesized?
(3) Can these same products be accessed in more environmentally friendly and sustainable ways?
(4) How can phosphorus be recovered and recycled?
(5) What barriers need to be overcome to re-establish the phosphorus cycle?
|
Introduction
2019 marked the 350th anniversary of the discovery of one of the periodic table's most beguiling and multifaceted elements. Phosphorus was first discovered in 1669 by Hennig Brand, an alchemist from Hamburg, who heated the malodorous residues of urine with sand and coal in a quest for the “philosopher's stone”.1,2 The substance he isolated gave off a pale green glow, and he thus named it phosphorus, from the Greek for light-bearer. We now know that Brand had isolated white phosphorus, an elemental allotrope that consists of discrete P4 tetrahedra. White phosphorus was originally (and misguidedly) sold as a medicine for a wide range of ailments, but the pyrophoric nature of P4 resulted in its subsequent use as a weapon in warfare, both as an incendiary agent and for creating smoke screens. Despite these nefarious uses, phosphorus is also an element that is essential to all known forms of life. Phosphorus is most commonly found in nature in its highest oxidation state (+5). Phosphorus is required for the formation of nucleotides, which comprise DNA and RNA molecules, and is a crucial component of ATP (adenosine triphosphate), which is the primary energy carrier in cells. Furthermore, phospholipid bilayers are found in the membranes of cells, and calcium phosphate is the primary component of mammalian bones and teeth. The essential nature of phosphorus combined with its relatively low abundance compared to other essential nutrients has led to the element being dubbed “life's bottleneck” by Isaac Asimov.3
The natural phosphorus cycle, which has been established over geological timescales, first involves the weathering of phosphorus-containing minerals into soil and streams. From here it is taken up by microbes and plants, and subsequently by animals that eat the plants, and used to promote growth. The phosphorus returns to the soil via excretion from animals during their lifetime, and by decomposition of plants and animals after death, where it can be taken up again by living creatures. This part of the process, the phosphate being taken up by animal and plant life and subsequently returned to the soil, can be repeated numerous times. Over time, some phosphorus is lost to waterways and eventually the sea, where over long periods of time it is reincorporated in sedimentary rock. Like most natural cycles, the phosphorus cycle has not necessarily remained completely constant over time; studies have shown historical ebbs and flows,4 but given the long timelines involved nature has always managed to redress this balance.
This natural equilibrium has been severely impacted by human activity.5 Deforestation, and the associated soil loss, has led to more rapid loss of phosphate from the ground into surface waters. Furthermore, phosphate-based fertilizers have been required to feed an ever-growing global population, and this has required extensive mining of phosphate rock (PR); approximately 20 Mt P is annually extracted from the earth.6 This is problematic because PR is a finite and dwindling resource. Furthermore, it is not equally distributed around the globe, with three quarters of the supply found in Morocco and Western Sahara, and other significant quantities in China, the USA and Russia. This asymmetric distribution of such an essential resource, combined with its economic volatility (the price of phosphorus briefly increased eightfold in 2008) could lead to sensitive food security situations and future political tensions.7 An accurate value of total useful reserves of PR is hard to predict, since this is highly dependent on the price. When the price increases, currently uneconomic reserves may become economically viable. Furthermore, when there is scarcity, the incentive to search for new reserves is higher. Due to this uncertainty, the estimates for depletion of PR reserves range from 40 to 400 years.8
The increased amount of phosphate in the soil from fertilizers and animal excrement also leads to an inevitable increase in the loss of phosphates into waterways. This is not merely wasteful; it has led to anthropogenic eutrophication, which is the process where the increased phosphate content leads to enormous blooms of algae, which depletes the water of oxygen and decimates marine ecosystems.9 It is apparent that the geological formation of PR from this run-off is negligible compared to the last century's intensive use of fertilizers and phosphate-based feed and food additives, and the phosphorus cycle is no longer a cycle; it is instead an unequivocally linear process.
There is therefore a problem of not enough phosphorus, with the dwindling supply of accessible PR, and of too much phosphorus in our surface waters leading to environmental concerns. These dovetailing problems necessitate the need for efficient phosphorus recovery and recycling schemes, which are essentially replacing the geological regeneration of PR on a timescale relevant to the human-disrupted phosphorus cycle (Fig. 1).
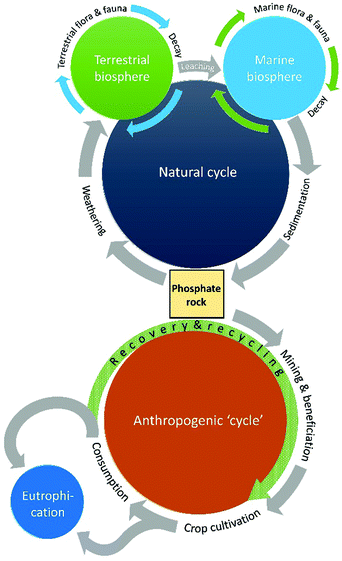 |
| Fig. 1 Schematic representation of the natural and anthropogenic phosphorus cycles. | |
This Tutorial Review will first look at current industrial routes to phosphorus-containing products, followed by relevant and sustainable routes to similar compounds that have been demonstrated on a laboratory, pilot or industrial scale. We will then explore the various ways phosphate can be recovered and recycled, followed by the barriers and obstacles to fulfilling this goal of closing the loop on the modern phosphorus cycle.10
Traditional routes to phosphorus and phosphates
The phosphorus required for industrial processes is traditionally obtained by mining PR. This consists mainly of apatite: Ca5(PO4)3X, (X = OH, F, Cl), with fluoroapatite (Ca5(PO4)3F) the most common. There is often a high degree of isomorphic substitution of carbonate ions for phosphate ions in naturally occurring PR as well, depending on geographical location. Over 80% of mined phosphorus comes from sedimentary PR, which has a relatively high phosphate content (reported by convention as phosphorus pentoxide content) of up to 40% P2O5.11,12 However, the sedimentary deposits can contain a wide range of pollutants and heavy metal contaminants, including radioactive metals such as uranium and thorium. Depending on the requirements of subsequent steps and the final product, these impurities can be removed by subsequent treatment, often by precipitation or extraction. Igneous rock deposits typically have lower levels of radioactive impurities, but also much lower phosphorus concentrations (0.005–2% P2O5). Fortunately, beneficiation methods (mechanical separation of minerals, usually involving grinding and flotation) can yield PR with 35–40% P2O5 content.11 There are some metamorphic rock deposits that contain 0.01–1.3% P2O5, but these are often deep-lying and not very porous, which makes subsequent treatment more difficult, and as such these deposits are not yet economically viable. Seabed phosphate deposits are currently being explored in Namibia and Mexico, illustrating the ongoing quest for finding new exploitable PR reserves. After mining, the PR is ground and treated via the wet or thermal processes.
Wet process
The vast majority of PR, about 98% of it, is processed via the wet process to yield wet phosphoric acid.6 This process consists of acidulation followed by concentration and purification (precipitation and extraction). The acidulation is generally performed with sulfuric acid (H2SO4) to afford phosphoric acid (H3PO4) and the by-products phosphogypsum (various hydrates of CaSO4) and hydrogen fluoride (HF) according to eqn (1): | Ca5(PO4)3F + 5H2SO4 + 5nH2O → 3H3PO4 + 5CaSO4·nH2O + HF (n = 0, 0.5 or 2) | (1) |
Roughly five tons of phosphogypsum are produced per ton of phosphoric acid.13 Phosphogypsum is mostly landfilled (88%) or disposed at sea (10%) and requires careful deposit management such as containment and treatment of drainage water to avoid environmental problems; only a small amount of gypsum is used as raw material (<2%). Recent work has shown that valuable rare earth elements can be recovered from the phosphogypsum waste, allowing the remaining material to be used as an alternative to natural gypsum in cements or plasterboards.14
To prevent the formation of phosphogypsum, the acidulation can be carried out using nitric acid, which yields marketable calcium nitrate as the by-product. However, the cost of nitric acid is higher than sulfuric acid, so only 10% of the wet process carried out worldwide is performed with nitric acid.15 Hydrochloric acid can also be used to obtain a relatively pure product, but this poses a separation challenge to remove calcium chloride, hence it is chiefly operated in parallel with a solvent extraction setup to obtain purified phosphoric acid.
About 92% of the phosphoric acid is converted into fertilizers,6 representing a market valued at several tens of billion USD y−1. Many of the impurities from the PR, including the HF by-product, are still present in the phosphoric acid (and/or the phosphogypsum by-product) depending on the type of digestion process and the origin of the PR. The impurities can be removed by several methods, most commonly extraction and precipitation, which are chiefly used to produce feed- (5%), or technical- and food grade phosphates (3%);6 fertilizer grade acid is rarely treated to remove impurities. Extraction involves the transfer of the phosphoric acid from the aqueous phase to an organic phase (such as methyl isobutyl ketone or tributyl phosphate), while the majority of the impurities remain in the aqueous phase.15 Cationic impurities can be removed by adding sulfuric acid to form sulfate salts, which remain in the aqueous phase. The extraction methods do not work for arsenic and cadmium impurities, so precipitation methods are required. Arsenic can be separated by adding sodium sulfide, which leads to precipitation of arsenic sulfide, while cadmium can be removed using complexing agents such as dialkyldithiophosphoric acid esters (S = P(SH)(OR)2).16 If desired, cationic impurities (Fe, Al, Mg and Ca) can be removed by neutralizing the acid with sodium hydroxide, resulting in a mixed mono/disodium phosphate solution and the precipitation of metal hydroxides. However, this method is limited by the fact that the phosphoric acid is transformed to phosphate salts only usable for products such as detergents.
Thermal process
The thermal (or Wöhler) process is an energy intensive process that produces elemental white phosphorus (P4). The remaining 2% of mined PR is processed this way. The chemical reaction is actually remarkably similar to that in Hennig Brand's urine-boiling quest for the philosopher's stone, and involves the heating of PR with coke (carbon) and silica (SiO2; for CaSiO3 slag formation) to temperatures of 1500–1600 °C according to eqn (2). | 4Ca5(PO4)3F + 18SiO2 + 30C → 3P4 + 30CO + 18CaSiO3 + 2CaF2 | (2) |
The coke acts as a reducing agent to give diphosphorus gas (P2 – the heavier congener of dinitrogen), which dimerises and condenses to white phosphorus. The process has a large energy consumption of 12.5–14 MW h per tonne of P4 produced, but gives a relatively pure product that can be functionalised to a large range of phosphorus-containing chemicals (see Fig. 2).11 The market value of P4 and its primary derivatives is estimated at several billion USD y−1.6 A significant part of the global supply of white phosphorus is oxidised to phosphorus pentoxide (P4O10) and subsequently hydrolysed to afford high-purity thermal phosphoric acid, which is (also as its sodium, calcium and potassium salt) suitable for use in foodstuffs, beverages, toothpaste and detergents. The latter use is declining, due to a ban of phosphates in detergents in several parts of the world to help combat increasing levels of phosphates in water bodies. The only significant impurity in this thermal phosphoric acid is arsenic (5–30 ppm), which could arise from small amounts of AsP3 in the white phosphorus.17 The arsenic can be removed via addition of sodium sulfide and precipitation of arsenic sulfide (vide supra).
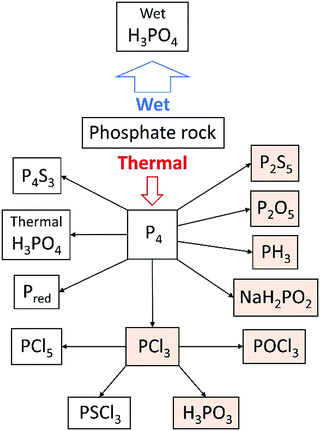 |
| Fig. 2 Use of phosphorus in the chemical industry, with precursors to organophosphorus compounds highlighted. | |
Organophosphorus compounds, i.e. molecules featuring a P–C bond, or mixed P–C and P–O–C bonds, are found in many bulk and specialty chemicals, such as flame-retardants, metal extractants and pesticides. Phosphines, PR3, are a widely used class of ligand to support homogeneous transition metal catalysts, as a key component of Wittig reactions (see below), and over the last decade have been explored as part of frustrated Lewis pairs for small molecule activation and metal-free hydrogenations.18 The vast majority of organophosphorus compounds are industrially synthesised using phosphorus trichloride (PCl3) as a precursor, which is generated in turn by the direct chlorination of white phosphorus. This process can also afford other chlorinated derivatives, such as PCl5, PSCl3 and POCl3. Phosphorus trichloride can be functionalised using Grignard or organolithium reagents, or by reaction with halogenated organic compounds under forcing reducing conditions.19 They can also be converted to phosphonates (including glyphosate) in a modified Mannich reaction, or to a range of alkyl and aryl phosphites by treatment with alcohols in the presence of an amine to capture HCl.
Alternative routes to organophosphorus compounds
PCl3 is a very useful chemical synthon for the synthesis of organophosphorus compounds, but is not an ideal reagent in terms of sustainability and safety due to its inherent corrosiveness, high toxicity and moisture sensitivity.20 It is a precursor on the list of Schedule 3 substances of the Chemical Weapons Convention, and thus its manufacture is carefully regulated. Moreover, very few of the final products contain a P–Cl bond, so the use of the toxic chlorine gas to functionalise white phosphorus can be regarded as a necessary yet wasteful step. Therefore, there has been a lot of research into finding alternatives to PCl3 for the synthesis of organophosphorus compounds. Phosphine gas, PH3, is used on industrial scales to synthesise organophosphorus compounds, although it is itself highly toxic and pyrophoric (the latter on account of trace P2H4 impurities). Phosphinate esters, also called hypophosphites (H2P(O)(OR)), and sodium hypophosphite (NaH2PO2), have also been explored in the formation of organophosphorus compounds via P–C bond formation by hydrophosphinylation of alkenes and alkynes.21 In particular, sodium hypophosphite is used in the synthesis of aluminium diethyl phosphinate, which is produced industrially as a flame-retardant (Fig. 3A).22
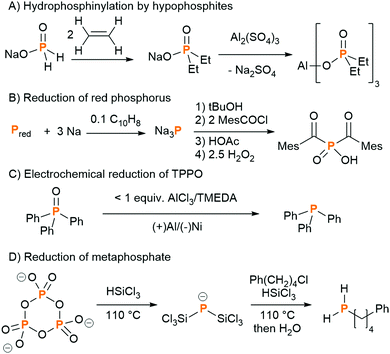 |
| Fig. 3 Selected examples of methods to synthesise organophosphorus compounds avoiding the use of PCl3. Mes = mesityl; OAc = acetate; TPPO = triphenylphosphine oxide; TMEDA = tetramethylethylenediamine. | |
To circumvent the use of a PCl3 surrogate at all, the direct functionalisation of white phosphorus has been researched in earnest since the 1970s. The reactivity of the P4 tetrahedron, usually attributed to the inherent ring strain, is difficult to control, with nucleophilic, electrophilic, and radical pathways sometimes operating concurrently. A large range of phosphorus-containing products have been synthesised from P4; this topic has been reviewed extensively, with reviews focusing on P4 activation by transition metals, main-group systems and via electrocatalytic functionalization, and will not be further discussed here.23 White phosphorus is the most reactive allotrope of phosphorus, so efforts to use more benign red phosphorus as an alternative to PCl3 necessitate more forcing conditions. Red phosphorus, which is formed by heating white phosphorus to 250 °C, has a chain-like polymeric structure, and is used as-is in matchboxes and flame-retardants, but is not relevant as a separate raw material for follow-up chemistry. In fine chemicals synthesis on lab or small industrial scale, red phosphorus is reduced using alkali metals to form highly reactive metal phosphides (M3P; M = Li, Na, K). These are usually generated and functionalised in situ, often via the protonated analogues (M2PH and MPH2), affording a diverse array of phosphines and phosphine oxides. As a representative example, bis(mesitoyl)phosphinic acid, a water-soluble and stable analogue of the commercially available photo-initiators known as bis(acyl)phosphine oxides (BAPOs), was recently synthesised by Grützmacher and co-workers in a one-pot procedure from red phosphorus (Fig. 3B).24 Note, further processing of white or red phosphorus can lead to other allotropes: violet phosphorus, which consists of a polymer of alternating [P8] and [P9] units linked by pairs of P atoms, and black phosphorus, which is a layered structure of puckered six-membered rings.25
There are examples of recycling of certain phosphorus compounds in small and self-contained cycles. The Wittig reaction, named after the chemist who shared the 1979 Nobel Prize for Chemistry for its discovery, is used on an industrial scale in the synthesis of vitamins and pharmaceuticals. In this process, a phosphine (often PPh3, TPP) is first converted to a phosphonium ylide, which reacts with an aldehyde or ketone to generate the desired alkene and an equivalent of the by-product phosphine oxide (OPPh3, TPPO). The phosphine oxide is typically treated as waste. The chemical company BASF have developed a large-scale recycling process for TPPO, which involves chlorination with phosgene and reduction with aluminium powder.26 Although these hazardous reagents and conditions do not comply with all the tenets of green chemistry, the process is still more environmentally sustainable and cost effective than disposing of the TPPO and having to synthesise new TPP from PCl3, sodium and chlorobenzene. Catalytic variants of the Wittig reaction have also been developed on a laboratory scale, using milder reducing agents such as diphenylsilane (Ph2SiH2), to convert the TPPO to TPP in situ,27 and electrochemical methods have very recently shown great promise (Fig. 3C).28,29
All of the above sustainable methods for the generation of phosphorus-containing fine chemicals still rely on the thermal process for the initial conversion of PR to P4. Recently, Geeson and Cummins found a way to circumnavigate this prerequisite, and showed that such organophosphorus compounds could be prepared from phosphoric acid derivatives. They showed that the trimetaphosphate salt, prepared from the dehydration of phosphoric acid using sodium chloride, could be reduced to the novel bis(trichlorosilyl)phosphide anion, [P(SiCl3)2]−, using trichlorosilane (Fig. 3D).30 This anion offered access to compounds including primary and secondary alkyl phosphines, which can be converted to organophosphinates and other building blocks for pharmaceuticals. In a subsequent report, the dehydration step was eliminated, and the same [P(SiCl3)2]− anion could be accessed directly from phosphoric acid and trichlorosilane.31 In this case, isolation of the pure [P(SiCl3)2]− anion was not possible, but it could be generated in situ and reacted further to an organophosphorus derivative. These studies show that it really is possible to completely bypass the use of white phosphorus in the synthesis of useful organophosphorus compounds,32 and it will be interesting to see if these can be developed into useful industrial processes. It is worth noting that the industrial process to produce trichlorosilane involves the use of elemental silicon, and elemental silicon requires similar amounts of energy to produce as white phosphorus, although recent studies have shown that SiCl4 (which can be converted to HSiCl3) can be formed directly from orthosilicates.33
Phosphorus recovery and recycling
The examples of sustainable phosphorus chemistry mentioned thus far are all innovative but are limited to linear processes (or small self-contained cycles such as the TPP/TPPO recycling), and do not contribute significantly to closing the phosphorus loop on a global scale. Awareness of the need to better manage the planet's dwindling resources has increased exponentially over the recent decades, and there has been a growing effort towards developing a circular economy.34 This is particularly relevant in the chemical sector and the principles of circular chemistry were recently outlined.35 The overarching idea is that all waste should be collected and used, and this notion is gaining traction in the phosphorus industry.
There are many phosphorus-containing waste streams that, if properly exploited, have the potential to replace a significant portion of the phosphorus produced by traditional mining. Van Dijk et al. mapped out the phosphorus-containing streams in the EU-27 for the year of 2005.36 Of the total amount of imported phosphorus in 2005, 51% was lost as waste, 39% accumulated in the soil and the remainder was exported. The largest share (54%) of losses is from human consumption, of which 55% was lost via wastewater. This loss of phosphorus into wastewater therefore amounts to 15% of the total imported phosphorus, making wastewater and its accompanying solid fractions prime candidates for nutrient recovery, especially as a lot of the necessary infrastructure is already in place.
Wastewater is generally treated in wastewater treatment plants (WWTPs), where phosphate can be recovered from multiple sources: aqueous streams (untreated wastewater, urine, effluent of treatment), sewage sludge, or sewage sludge ash (SSA). Furthermore, there are alternative sources of phosphate, including manure, slaughter waste and steelmaking slag. All of these various sources will be examined in more detail, and the nature of the recovered products for further use will be highlighted where appropriate. We have included a selection of real-world industrial processes to showcase what is already being carried out and the challenges that remain in ensuring a fully circular phosphorus industry.
Phosphorus removal at wastewater treatment plants (WWTPs)
There are several different forms in which phosphorus can occur in wastewater. For example, wastewater from the animal and livestock sector generally contains large amounts of organic phosphates like phytic acid, a predominant form of phosphate in monogastric animal manure. When looking at domestic wastewater, however, orthophosphate (PO43−) is the most abundant. As this represents a far larger stream in terms of volume, most phosphate removal and recovery techniques have focused on removing orthophosphate over other phosphate derivatives. It is worth noting that phosphine gas (PH3) has been observed in sewage sludge, where microorganisms are able to produce this gas under anaerobic conditions.37 This is especially pertinent given the recent use of phosphine gas as a potential biomarker in the atmosphere of Venus.38
Since the issue of eutrophication has been known for a long time, the development of technologies aimed at reducing the concentration of phosphorus in WWTP effluent started as early as the 1950s. Two predominant technologies emerged which have since seen global implementation: the chemical precipitation of metal phosphates and enhanced biological phosphorus removal.39
Chemical precipitation.
Metal phosphate precipitation is a straightforward technology based on the addition of iron or aluminium salts in the form of chlorides or sulfates, forming insoluble phosphate salts which can be removed after sedimentation (for the solubility constants of relevant phosphate salts, see Table S1 in the ESI†).39 As a simple technique, it can be applied at various stages in the wastewater treatment process. Primary precipitation entails the addition of chemicals before sedimentation, and the precipitate ends up in the primary sludge. Secondary precipitation is where the iron or aluminium salts are directly added into the aeration tank of an activated sludge process, with the phosphorus consequently ending up in the secondary sludge. The third option is adding the flocculant post-sludge treatment, producing a relatively pure tertiary metal phosphate sludge. Due to the costs of handling an additional stream however, this has historically not been the method of choice.
Enhanced biological phosphorus removal (EBPR).
As the name implies, EBPR does not involve chemical dosing but instead relies on microorganisms to remove P from wastewater and is also widely used in WWTPs. The workhorses of this technology are a specific type of microbe, collectively named polyphosphate accumulating organisms (PAOs). They can sequester phosphate as intracellular polyphosphate in excess of their biological need under aerobic conditions, also dubbed “luxury uptake”. More than 90% of the phosphorus present in the wastewater can be removed this way, and where normal sludge biomass usually contains about 2–3% of phosphorus, reports have been made of P-contents ranging from 4% up to 12% post-EBPR.40 By subsequent removal of the sludge, net P removal from the wastewater is achieved. Part of the sludge is recirculated back to the aerobic tank where the process starts over. When applying subsequent anaerobic conditions however, the polyphosphates are released back into the solution as orthophosphate allowing for an effective concentration of P in the liquid phase. This is imperative for phosphorus recovery from the aqueous phase to be viable.
Both chemical precipitation and EBPR are effective in the removal of phosphorus from wastewater. However, the P removed is generally contained in the waste sludge and not valorised other than incidentally being applied to agricultural land, or otherwise incinerated. The latter sees more and more implementation in a number of European countries, following concerns over spreading of drug residues, heavy metals and pathogens onto arable land via the sludge. Furthermore, FePO4 and AlPO4 lack proper bioavailability and consequently have lower or little nutritional value depending on soil type.
Phosphorus recovery at WWTPs
As the paradigm regarding wastewater handling is slowly but surely shifting from nutrient removal towards resource recovery and recycling, other more bioavailable precipitates from the aqueous phase based on magnesium and calcium have started to gain interest. This section will discuss recovery of various phosphates from the aqueous phase, sewage sludge, and the incinerated form of the latter, sewage sludge ash. All discussed technologies are represented in Fig. 4.
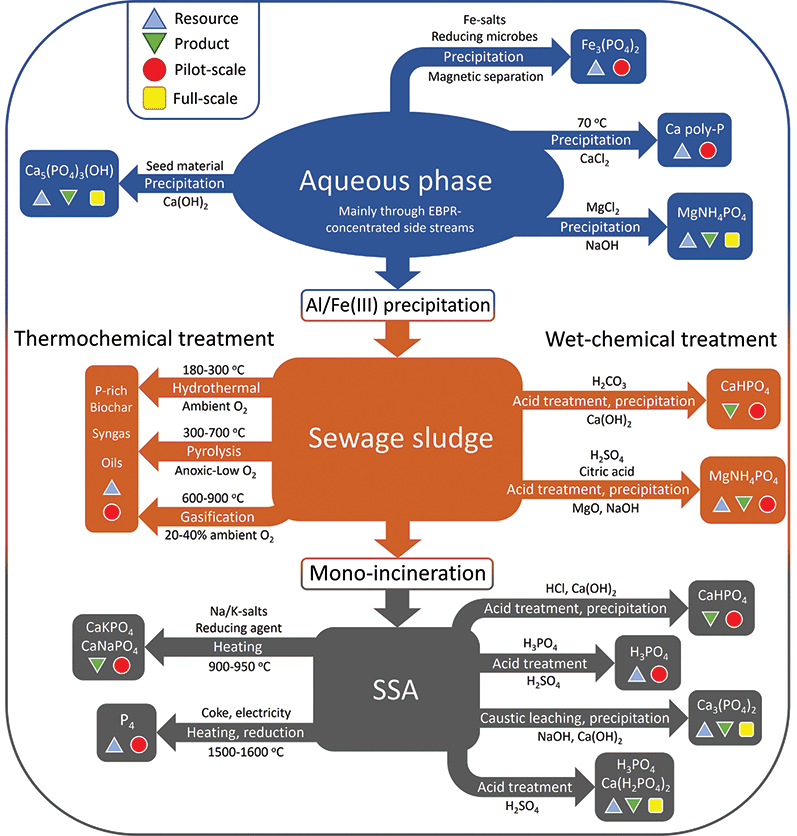 |
| Fig. 4 Schematic overview of P recovery processes. | |
Aqueous phase.
Without prior concentration of P using EBPR, only about 10–40% P can be recovered from the aqueous phase, depending on P already being bound to other metals or biomass. Furthermore, the liquid phase should have a phosphate concentration of at least 50–60 mg L−1 for recovery to be economically viable, depending on the method employed.41 More importantly, however, constituent ions need to be present in sufficiently high concentrations for precipitation to occur at all, and P concentrations of 100 mg L−1 are mentioned for effective precipitation of specific magnesium phosphate salts.42 As these numbers are exceptionally high for untreated wastewater, the P-rich reject liquid phase of EBPR anaerobic treatment is generally used for the precipitation processes from the aqueous phase discussed herein.
Struvite.
Struvite is a salt consisting of equimolar amounts of magnesium, ammonium and phosphate (MgNH4PO4·6H2O). Nowadays, struvite is hailed as a promising way to recover phosphorus from wastewater, but initial reports were not as positive; it was found as mineral encrustations inside digester pipelines, reducing flow throughput and necessitating costly maintenance and downtime to remove. Indeed, when constituent ions are present in sufficiently high concentrations and conditions are alkaline, struvite can spontaneously precipitate.43 As such, the controlled precipitation of struvite has gained interest, serving two purposes: the omittance of costly maintenance and the recovery of phosphate. A significant added benefit is the simultaneous removal of ammonium from the wastewater.
Struvite precipitation processes are the most widely implemented of the commercialised P recovery techniques, partly due to their simplicity. A representative example is the Belgian NuReSys (Nutrient Recovery Systems) process. The P-rich effluent from EBPR anaerobic treatment is fed into a stirred tank reactor, where magnesium is added in the form of soluble MgCl2 to achieve an equimolar Mg
:
P ratio. The pH is subsequently elevated to 8–9 using NaOH, at which point struvite crystals start to form. The process is capable of reaching 90% recovery of the P fed into the reactor.44 However only up to 40% of the P in the WWTP can be harvested this way; the remaining >60% is lost to the sludge, where its reduced concentration hampers attempts to recycle this remaining part. Struvite finds application as slow-release fertilizer, but as produced amounts increase, large scale applications are still missing.
Calcium phosphate.
Calcium phosphate recovery is also a straightforward process, although it has been less widely implemented than the analogous magnesium process. The most prominent example is the original DHV Crystalactor process.45 It uses a fluidized bed reactor with suitable seed material, generally quartz. Ca(OH)2 is added as precipitating agent, after which calcium phosphate precipitates at a pH of 9. Calcium phosphate can be used as slow-release fertilizer or as feedstock for regular fertilizer production. However, Crystalactor units for phosphate recovery have not seen large implementation beyond the food industry due to economic reasons. Interestingly, calcium polyphosphates can also be the target recovered material, which is the case in the Heatphos process. Here, instead of applying anaerobic conditions to release orthophosphate during EBPR treatment, polyphosphates are released by heating the sludge at 70 °C for 1 hour. The poly-P is easily precipitated by subsequent CaCl2 addition. A marked advantage over other precipitation processes is that elevated pH is not necessary, reducing necessary chemicals. After calcination, the calcium poly-P can be mixed into the traditional wet process for phosphoric acid production.11
Iron phosphate.
Another promising metal phosphate for P recovery is vivianite (iron(II) phosphate). Ferric iron (Fe(III)) is much more common under ambient conditions due to the presence of oxygen, however, vivianite forms readily under reducing conditions. This can be induced under anaerobic digestion conditions, where microorganisms also responsible for biogas formation can reduce Fe3+ to Fe2+. Other key factors are the pH and sulfate concentrations, both due to competing reactions. Neutral pH (6.0–8.0) is preferred, as more alkaline conditions will start to favour the formation of Fe(OH)2, whereas acidic conditions will simply dissolve the salt.
The vivianite can be separated from the sludge using a magnetic separation process. Although there is a recent increase in research into vivianite, it remains an iron phosphate which is not directly compatible with the mainstream fertilizer industry; more research is required for this to become a widespread strategy in industry.46 Kemira's Vivimag process aims to leach phosphate from vivianite, to yield a fertilizer precursor and iron salts for re-use in wastewater treatment.
Sewage sludge.
More than 30 million tons of sewage sludge is produced per annum in developed countries alone. As stated earlier, about 90% of influent P at WWTPs can be incorporated in digested sludge. It is one the most concentrated forms of phosphorus in organic waste streams, second only to bone meal. There are currently a number of ways to dispose of the sludge: co-incineration in the cement industry, landfill, agricultural use, or (mono)incineration, which provide opportunities for more sustainable P recovery and recycling. The cement industry uses sewage sludge as fuel in clinker production, which leads the P contained therein to become locked and diluted in cement. Actually, its presence is undesired for cement quality. Landfill is practised in all European countries to varying degrees. The disadvantages to this method are the evolution of methane, a powerful greenhouse gas, and the increasing associated costs as available land becomes scarcer. Agricultural use utilizes the large amounts of nitrogen, phosphorus and potassium nutrients as well as the organic matter present in the sludge. However, contaminants like heavy metals, pharmaceuticals, and pathogens are also prevalent, and these latter considerations have been the main argument for countries which have implemented legislation limiting or outright banning the use of sewage sludge in agriculture. Some countries where such legislation is in place, like the Netherlands, Switzerland, and increasingly Germany, have opted for the alternative option of incineration. This exothermic process yields highly P-rich ashes, which are discussed in a subsequent section.47 For the direct recovery of P from sewage sludge, a multitude of technologies have been proposed, some of which have seen real-world implementation. We can divide these in wet-chemical leaching and thermal technologies, related to the beneficiation of PR.
Wet-chemical treatment of sewage sludge.
This process involves the release of P into solution by first treating the sludge with a mineral acid. Wet-chemical leaching technologies build on the previously discussed precipitation technologies, however the acidic leaching inevitably co-solubilizes heavy metals also present in the sludge and potentially large amounts of iron and/or aluminium depending on whether metal phosphate precipitation was initially employed to remove P from the liquid phase. The omittance of metal incorporation is imperative for recovered materials to meet legislative and quality requirements, and can be achieved in various ways. A good example is the Stuttgart process,11 which has been operational as a large-scale test plant over the previous decade at the WWTP Offenburg, Germany. P is leached from the sludge using sulfuric acid (pH ≈ 3). To safeguard the quality of the precipitated struvite, citric acid is added as complexing agent prior to MgO and NaOH addition, and the resulting metal citrate salts stay in solution during struvite precipitation. A process developed by Budenheim in Germany uses leaching of phosphate from sludge by carbonic acid under pressure, followed by precipitation as calcium phosphate.
The P recovery potential for these processes depends strongly on process conditions like leaching pH and P speciation in the sludge. In the case of the Stuttgart process, recovery values of 50 and 80% are reported for sludge with prior phosphate precipitation using Al or Fe salts, respectively. An overall recovery rate of 65% is reported by Egle and co-workers.48
Thermochemical treatment of sewage sludge.
Many processes for sewage sludge treatment make use of elevated temperatures, and a range of different processes are employed. These can be generally classified as hydrothermal treatment, pyrolysis and gasification. The processes are typically differentiated by the temperature used and the amount of oxygen present, as represented in Fig. 4 (see also Table S2 in the ESI†).
Harsher temperature and higher oxygen profiles tend to achieve satisfactory depollution in terms of pathogens and organics, but inorganic metal- and metalloid contaminants generally persist throughout these types of treatment, and exceed limits set for fertilizers and soil improvers as stated in the EU Fertilizing Products Regulation.49 Based on these considerations, materials obtained from pyrolyzed and gasified sewage sludge appear to have limited potential on the fertiliser market, and require further treatment. The described thermal treatments are, however, suitable to process a range of less contaminated materials like manure, bones, and food waste. None of these (hydro)thermal processes have seen widespread implementation yet.
Sewage sludge ash (SSA).
Incineration of sewage sludge to afford SSA reduces the volume by more than 90%, and the ashes have a P-content between 9–13.1%.50 Furthermore, organic pathogens and pharmaceutical contaminants are destroyed in the process, and the fact that SSA is a dry and free-flowing powder makes subsequent handling relatively easy. The main downside of sewage sludge incineration is the fact that large infrastructural investments for incineration facilities are needed, even though benefits of economy of scale and centralization can mitigate this. It is highly unlikely countries will invest in these practices for the sake of P recovery alone, especially in areas where the application of sewage sludge to arable land is still practised. However, the process becomes more attractive when the direct use of sewage sludge has been banned altogether, like in Switzerland and the Netherlands, where all the sewage sludge is mono-incinerated. Currently, these ashes are often incorporated in cement or asphalt or simply sent to landfill, effectively wasting valuable resources, as about 87% of the P in WWTP influent persists in SSA.48
Many of the metals present are non-volatile at the employed temperatures and conditions. As such, the large reduction in volume also means that heavy metals become more concentrated. This, in combination with low reported bioavailability of the present P-species exclude the option of using SSA directly as fertilizer. Consequently, valorisation of the ashes is needed to recover P in a usable form, and various technologies have been developed to this end. By direct analogy with the treatment of sewage sludge, the methodologies for treating SSA can be divided into wet-chemical processes and thermochemical processes.
Wet-chemical treatment of SSA.
The majority of SSA valorisation technologies employ wet-chemical treatment, which are either based on acidic or alkaline leaching. Acidic leaching is quite similar to previously discussed processes involving acid digestion, and is the most frequent technique seen for SSA treatment. Different acids can be employed in this process, including sulfuric acid, hydrochloric acid, phosphoric acid, and carbonic acid, and some of these processes will be highlighted below.
The traditional wet phosphoric acid process discussed earlier is in essence an acidic wet-chemical treatment. As SSA has such a high P-content, both ICL in the Netherlands and Nippon Phosphoric Acid Co. Ltd (NPA) in Japan started trials mixing in ashes with PR to feed their production facilities in the hopes of integrating this secondary feedstock into existing infrastructure and this has been reasonably successful. NPA was able to use a blend ratio of up to 2.5% SSA without significantly affecting plant performance, product quality and pollution levels. After acquiring the necessary industrial waste disposal business licence, mixing practices were implemented commercially in 2013, accepting about 1300 t y−1 of SSA from local sludge incineration facilities. The main issue with this practice is the fact that the relatively large amounts of heavy metals present in SSA are incorporated in the fertilizer product. ICL blends SSA into its TSP (solid fertilizer) production, with the advantage that the ash does not pass through a truly liquid phase preventing excessive metal solubilisation. Metal contamination starts to become a real issue for blends above 2.5% SSA, and technologies to remove metals from phosphoric acid solutions have not been implemented due to economic considerations. As such, SSA originating from WWTPs employing chemical precipitation with iron and/or aluminium is less suited for blending than that of WWTPs employing biological P removal.11
Straight acid digestion of the ash with mineral acids is also possible. The TetraPhos process (Remondis) leaches phosphate from the ash by means of phosphoric acid, which is then treated with sulfuric acid to give phosphoric acid, and acting as the ultimate source of the acid. The EcoPhos process, sold to Prayon after EcoPhos went bankrupt, uses HCl digestion on the ash followed by precipitation of the phosphate with lime to give dicalcium phosphate. The remaining calcium chloride can be disposed of at sea, while heavy metals remain with the insoluble residue or are removed by ion exchange.
Another method of acidic treatment is the use of CO2 instead of mineral acids. Blowing CO2 into an aqueous suspension of the ash forms carbonic acid, which can react with the phosphate in the ash to form phosphoric acid. A less widely utilised alternative to acidic wet-chemical treatment is the alkaline counterpart. The alkaline leaching is practised on an industrial scale in Gifu, Japan, by treating SSA with hydroxide solutions. The leached ashes are washed and subsequently treated with a dilute H2SO4 solution, which removes the toxic heavy metals. The resulting solids can be used in cement, asphalt, as roadbed material or as soil improver. The P extraction rate is strongly dependent on the calcium (CaO) content in the ashes, and overall a P recovery of 30–40% is reported, much lower than acidic treatment processes. Chemical costs are also significant due to the large amounts of chemicals needed. On the other hand, the method does remove a lot of the heavy metal contaminants, and while it is not the most efficient process in terms of P recovery, it is fully operational and has been supplying local farmers with over 300 t y−1 of recovered fertilizer since 2009.11 Economics depend on the local availability of caustic soda waste, which is relatively expensive when bought at the market.
Thermochemical treatment of SSA.
The thermochemical treatment of SSA involves reacting the ash with dosed chemicals at elevated temperatures. The main goals of this are to remove heavy metal contaminants and increase the bioavailability of P. A key example of this is Outotec's AshDec process, on which research started back in 2004 in Germany and Switzerland.11 In this process, ashes are fed into a rotary kiln dosed with sodium, magnesium or potassium salts and a reducing agent, preferably dry sewage sludge, and heated to 900–950 °C for 15–20 minutes. Ideally, the ash is taken directly from an adjacent incineration reactor, reducing energy requirements as the ashes are still hot. The system ensures heavy metal removal by volatilization in a reducing atmosphere, giving bioavailable CaKPO4 and CaNaPO4 compounds as products. Copper and zinc, the most common heavy metals in SSA, are removed to a large extent and the product meets fertilizer regulations.
For more extensive metal removal, for example in the case of high copper concentrations, inorganic chlorides like MgCl2 or CaCl2 can be added to generate volatile metal chlorides, in this case CuCl2.11 The downside of this practice is that the formed chlorapatite (Ca5(PO4)3Cl1−x(OH)x) has low overall bioavailability, and farringtonite (Mg3(PO4)2) and stanfieldite (Ca4Mg5(PO4)6) only show agronomic efficiency in acidic soils. Interestingly, the AshDec technology was initially based on the use of chlorides due to more stringent regulations on recycled fertilizers in place in Germany (until 2008) and Switzerland (until 2017). As more accessible limit values became the norm, the process switched to additives allowing for more bioavailable products. One downside of the technology is its large energy consumption, but a significant benefit is the fact that it is not limited to the use of SSA as feedstock, but is compatible with other P-rich ashes as well, as are described in the following section.
White phosphorus can be produced from SSA in a classical P4 furnace, however Fe levels need to be limited to prevent the formation of excessive amounts of a less valuable by-product, ferrophosphorus. This limits suitable ashes to WWTP sludges with Al precipitation and EBPR as P removal technology. A new process indicating to bypass the iron issue was developed by the RecoPhos consortium, a technology now acquired by Italmatch, which uses an inductively heated coke bed to reduce SSA.
Alternative phosphorus sources
While we have so far focused mostly on P recovery from wastewater, there are a number of alternative waste streams from which phosphate can be recovered and utilised. These are mostly animal-derived waste streams. First and foremost, livestock manure represents a large waste stream rich in P. In fact, with 1.6 Mt P per year, it is the largest source of recyclable P in Europe.36 It is a much more localized stream compared to municipal wastewater. In most countries, manure is simply applied to land as organic fertilizer. However, countries with dense livestock industries like the Netherlands and Belgium generate large volumes of manure, but arable land to apply it to is limited. As such, the risk of manure overapplication in these livestock-intensive regions is a real problem, both in terms of nutrient loading as well as the accumulation of contaminants present in the manure. Consequently, other ways of disposal are warranted, provided this type of livestock-intensive agriculture persists. The transportation of excess manure to other areas presents a logistical challenge, as it is a bulky (water-rich) substance and therefore highly unfavourable to move around from an economic perspective, especially over longer distances.51 Local treatment is therefore warranted, and there are numerous options to this end. Oftentimes, the first manure treatment step is anaerobic digestion, producing valuable biogas (methane). This can be applied to wet manure, but more generally its dewatered counterpart. This is also the case for thermal treatments like wet oxidation (hydrothermal), pyrolysis and gasification. However, these are merely volume reductions to make transport less expensive, and do not address the local oversupply of P, which remains in the digestate. Alternatively, follow-up treatments of the resulting biochar as discussed in the section on sewage sludge thermal treatment can also be applied. Similarly, the incineration of manure is also an option, recovering its energy content at the expense of C- and N-based nutrients. The ashes can be treated by analogous methods to the SSA, although this practice would also require significant infrastructural investments. Furthermore, the resulting liquid fraction from dewatering can be used for the precipitation of calcium phosphate or struvite. This only contains up to 30% of the total P however, and may not be economically viable in all cases.52
Another large P-containing waste stream is Category I meat and bone meal (MBM). MBM is a by-product of the rendering of animals and is rich in a range of nutrients. Historically, MBM was generally processed towards animal feed, but due to the BSE (bovine spongiform encephalopathy, or “mad cow disease”) crisis this practice was partially banned in the EU. While legislation was softened somewhat a number of years later, large amounts of MBM still need to be disposed of, with MBM production exceeding 3.5 million tons per year in the EU.53 Since then, MBM is often incinerated to valorise its energy content in cement works, and destroy any pathogens. By using MBM in cement works, the P ends up diluted and locked in concrete. When mono-incinerated, still a rare practice in Europe, the ashes (MBMA) mostly comprise Ca5(PO4)3OH and Ca3(PO4)2, and have a composition closely reminiscent to that of PR, exhibiting P contents as high as 15–19% with similar or lower heavy metal content.54 As such, MBMA is a prime candidate for P recycling, and there are numerous ways to valorize the P content in these ashes. The most straightforward is acidic leaching, similar to the wet-chemical methods handled in previous sections. Extensive pilots have been conducted by ICL mixing MBMA into existing (wet) processes based on PR, yielding good results.11 Furthermore, P valorization technologies applicable to SSA can be extended to MBMA, with the latter exhibiting better properties in terms of P content and heavy metal contaminants.
Another waste stream of interest is steelmaking slag.11 The raw materials of steelmaking (iron ore, CaO, and coal) do not contain much phosphorus (0.05–0.06 wt%), but the impurities are concentrated in the steel production process and end up unevenly distributed in the slag. Phosphorus has a negative impact on the quality of steel and is therefore actively removed. Since steel production is one of the larger industrial processes worldwide, this results in vast amounts of slag with concentrated levels of phosphorus compared to its parent raw material (0.3–1.7 wt%). Multiple techniques have been proposed for P recovery from steelmaking slag. These include magnetic separation, capillary action separation, aqueous dissolution and carbothermic reduction, as well as reductive melting. While the latter has been run at pilot-scale,11 currently it is not economically attractive to actively use steelmaking slag as a source of phosphorus. However, with the projected increase of PR pricing accompanying its depletion, this could become feasible in the future.
Closing the loop: drivers and barriers for recycling
The principal initial driver for P recovery was not so much focused on the recovery of P in a usable form, but rather its removal from wastewater to prevent pollution. Legislation has therefore been put into place in many developed countries, spurring the widespread implementation of chemical precipitation and biological treatment systems to treat wastewater and counter the harmful phenomenon of eutrophication. Nowadays, many WWTPs still employ either, or even both, but the resulting P-rich materials have seen only little reintroduction in the P cycle. Over the last couple of decades, however, P-rich streams like wastewater are increasingly seen as promising secondary resources of P, emphasizing the need for its recovery in usable, bioavailable form; the focus is shifting from P removal to P recovery and recycling. This shift is exacerbated by the increasingly pronounced public opinion on sustainability and circularity, and the need for it is underlined by the inclusion of PR (as well as P4) on the list of critical raw materials as formulated by the European Commission.
The uneven distribution of PR reserves has led to a lot of countries being highly dependent on imports and consequently subject to price fluctuations of the resource. In 2008, an extreme fluctuation occurred, driving up PR prices from about $50 t−1 a year earlier to $430 t−1,55 which in turn was largely responsible for sharp increases in food prices worldwide. Having a local, sustainable, and virtually inexhaustible source of renewable fertilizer would alleviate these issues to a large extent by decoupling food production from foreign and fossil resources. Much effort has gone into the development of P recovery technologies and significant progress has been made, as highlighted in this review. Still, commercially operating processes are not abundant, and most of them are based on struvite precipitation. The relative success of struvite can be explained by the fact that its removal addresses another issue: that of unwanted precipitant scaling in biological wastewater treatment piping. By removing the struvite in a controlled manner, costly maintenance can be prevented, which provides an additional economic incentive for targeted struvite precipitation.
There is however a distinction to be made between P recovery and P recycling, and their associated drivers. The reasons for the relative success of struvite are very much based on incentives for P recovery: the mandatory P removal from wastewater under the Wastewater Directive from a legislative perspective, and the prevention of maintenance costs from an economic standpoint. A good example of driving P recovery is the relatively stringent legislation in place in Switzerland since 2016 and Germany since 2017. Germany and Switzerland have since taken a leading role in the speed and degree of implementation of P recovery technologies compared to other European nations. Indeed, environmental regulations have always been a key driver for sustainable innovation. However, while this progress is to be applauded, it only solves part of the puzzle; P recycling, by using recovered P as resource for the production of marketable products, is needed to truly close the loop. To this end, recovery and recycling need to be aligned to make sure the recovered material is suitable for subsequent recycling, and value chains are needed to bridge the gap between them.11
Legislation
Legislation is a key factor in the promotion of P recycling. One issue from a legislative standpoint is that recovered materials are designated as waste. A waste material may only be moved across borders following a lengthy process involving written notifications and authorization in accordance with the Shipment of Waste Regulation,56 which has further hampered the development of a European sustainable P market. The EU has undertaken action to facilitate the use of recovered materials, by revising the Waste Framework Directive in 2018 and Fertilizers Regulation in 2019 as part of the Circular Economy Package launched in 2015.57 The new EU Fertilizing Product Regulation introduces product function categories (PFC) regarding materials to be used as fertilizer, and component material categories (CMC) which encompass raw materials for fertilizer production. The latter is expanded to now include compost, digestates, animal by-products, struvite, biochar, sewage sludge and its derivative ashes. When quality requirements are fulfilled, outputs have product status and are no longer considered waste, removing legal hurdles and promoting marketing of these materials. While these requirements will still be stringent, legislation on waste status has finally been harmonized, facilitating more facile free trade of CMC materials. Another important change is the introduction of a Cd limit on fertilizer. A maximum of 60 mg Cd per kg P2O5 is now mandatory. As virgin mineral fertilizers generally contain significantly higher Cd concentrations than recovered materials, this gives recycled material an advantage.57 However, having a facilitating legal framework in place is not enough. As noted for struvite, an economic driver is needed for P recovery technologies to become more widely implemented, which naturally extends to P recycling practices.
A paramount driving force towards P recycling would be to ban recovered P from going anywhere but a true recycling operation. As is clear from the technology review, the economics of P recovery are often weak, due to a number of factors (economy of scale, technological complications), hence blocking routes leading to P wastage in e.g. landfill, asphalt fillers, etc. is seen as the most effective way to create a true P cycle. The ban on agricultural spreading of sewage sludge in the Netherlands, Switzerland and parts of Germany has already helped in creating an infrastructure which makes large amounts of SSA available; the legislation enforcing P recycling until 2026 as now seen in Germany will make many technologies with marginal economics more viable.
Value chains
We cannot escape the need for economic viability. The P recycling sector will never truly thrive if there is no prospect of financial gain; something that cannot sustain itself will never truly contribute to sustainability, irrespective of how well the people and planet have been integrated into the concept. A value chain is needed to close the loop, where recovery serves as supply and recycling as demand (Fig. 5).
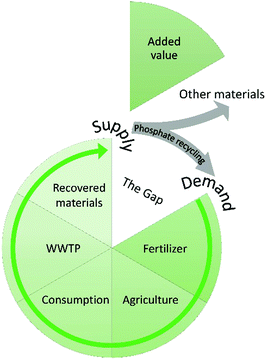 |
| Fig. 5 Necessity of a value chain to close the gap between supply and demand. | |
There are many stakeholders involved in the secondary fertilizer industry, including governmental bodies, WWTPs, P valorisation companies, farmers and the general public. Effective sales of a product rely on criteria on which stakeholders should be in alignment. This way, the (prospective) added value of a particular recovered material can be determined, which is necessary to close the gap. Examples are nutrient content, homogeneity of the material, purity, proof of agronomic efficiency and guarantees for sufficient quantities. Without them, a secondary fertilizer will intrinsically be of lower value or even lose all interest, and a value chain might not be established.11 Achieving such alignment is challenging, but there are inter-organizational platforms and international studies whose main goals are to facilitate this. A prime example is the European Sustainable Phosphorus Platform (ESPP), which comprises over 150 members stemming from various stakeholder groups in industry, science and policy. Its main goal is to promote P sustainability in Europe by identifying and addressing obstacles and facilitate opportunities like collaborative actions, business cases and the establishment of value chains. The Dutch Nutrient Platform in the Netherlands strives towards the same goals on a national level. In 2011, they initiated the Phosphorus Value Chain Agreement, bringing together twenty parties to further a sustainable market for P recycling.11 The recently published STRUBIAS report explored the technical and market conditions for using products derived from biogenic waste or other secondary raw materials as fertilizers, and showed that the agronomic efficiency of secondary fertilizers is generally in line with their PR-derived counterparts.49
Another important facet of stakeholder alignment in which these facilitating bodies play a role is educating the customer segment and raising general awareness; primarily, the way as to how recovered fertilizing materials are perceived by the market.11 In regions where there is some supply of recovered fertilizer like struvite or calcium phosphates, farmers have to date been reluctant to switch from traditional mineral fertilizers to its recovered counterpart. This is understandable, as the latter are predominantly slow-release fertilizers in contrast to the highly soluble ammonium- and potassium phosphates commonly used. Therefore, it is most desirable to produce known products, but using renewable resources. An example of the importance of customer education is the calcium phosphate product produced by alkaline leaching of SSA in Gifu, Japan. Upon full-scale operation of the process, no channels were in place for the sales of the material. To alleviate this issue and establish a value chain, a marketing campaign was launched including advertisements, briefing sessions for farmers, free sample offerings and leaflet distribution. Now, it is sold to local farmers and enjoys a good reputation, with sales volumes increasing annually and serves as a good example of how P recycling can be achieved on a local level.11 The challenge now is to translate these relatively small-scale examples to an international market.
Outlook
The interplay between stakeholders, recovery and recycling strategies and associated legislation is paramount for enabling secondary fertilizers to penetrate the market. Still, even in a hypothetical perfect case where all stakeholders are aligned, all legislation is adhered to and the value chain is in place, the market may still not favour secondary fertilizers over virgin ones simply due to competition. It has been postulated that for P recovery and recycling from wastewater to become truly self-sufficient, PR prices need to be at least 100 $US t−1.58 As of March 2020, prices were just above 70 $US t−1 (for Moroccan PR) and have been steadily declining over the last few years.55 Indeed, the low price of PR has to date been a key barrier for the implementation of P recovery systems. Still, despite this recent decline, prices are significantly higher now than they were before the price spike of 2008 and are expected to rise in the future as increasingly contaminated PR pushes mining and beneficiation costs. In time, this economic barrier is expected to become a driver, and value chains should be in place to capitalize on it when that happens. Analogously, production of more specialized, higher value P compounds from secondary sources could also start to become more viable. This may well prove to be a very interesting emerging market when recovered P materials start taking precedence over PR. It is expected that anywhere between 17 to 31% of P currently derived from fossil resources can be substituted in Europe by use of recycled materials in a fully-fledged market.49 A promising prospect indeed!
Conclusions
This review is an introductory text into the complex and wide-ranging topic of phosphorus recovery and recycling. It is important to change the way we deal with phosphorus and close the loop. The requirement for cheap chemical conversions from renewable phosphate resources to highly pure phosphorus-containing building blocks is imperative, and recycling schemes should be implemented rapidly. Chemical pathways should be assessed over the full cycle for effective recovery and effective recycling to usable products. Other factors that make a process industrially viable are often overlooked, and phosphate rock is currently still a relatively cheap resource. The factors that need to be considered for new recycling schemes to kick-start this process are politics, legislation, societal pressure, and of course economics. Sustainability goals set by the government can be a political driver, but for fertiliser producers and farmers, the ill-defined properties of recovery products such as struvite in comparison to established fertilisers form a barrier to adopting them. Social drivers are the popularity of the circular economy and the green image for marketing. Water boards are economically hindered by high investment costs and uncertainty of the return on investment for the implementation of struvite recovery processes. The reduction in maintenance costs however (prevention of blocking of pipes) is a strong driver. Scientists, policymakers and companies are on the right track to effectively recover and recycle phosphorus. They are learning to deal with new raw materials from waste, a myriad of recovery and recycling schemes and a great deal of uncertainty. By aligning such stakeholders, barriers can be mitigated and drivers exploited/amplified, allowing the establishment of value chains to become easier and thus more likely. These will prove imperative in moving away from fossil feedstocks, and towards renewable ones, and help to close the loop of the phosphorus cycle.
Conflicts of interest
J. C. S. is co-founder, shareholder and scientific advisor of SusPhos BV. W. S. is CTO and shareholder of SusPhos BV.
Acknowledgements
Renske Verhulst (Dutch Nutrient Platform) and Aalke Lida de Jong (AquaMinerals) are thanked for their feedback on a draft of this manuscript. This work was supported by the Council for Chemical Sciences of The Netherlands Organization for Scientific Research (NWO/CW) by a VIDI grant (J. C. S.), and a VENI grant (A. R. J.), a NWO KIEM GoChem grant with SusPhos BV (J. C. S.) and a CE-RUR-08-2018-2019-2020 grant LEX4BIO (J. C. S., grant agreement no. 818309).
References
- M. E. Weeks, J. Chem. Educ., 1933, 10, 302–306 CrossRef CAS.
-
J. Emsley, The 13th Element: The Sordid Tale of Murder, Fire, and Phosphorus, Wiley, New York, 2000 Search PubMed.
-
I. Asimov, The Magazine of Fantasy and Science Fiction, Mercury Press, Oklahoma City, 1959 Search PubMed.
- G. M. Filippelli, Elements, 2008, 4, 89–95 CrossRef CAS.
- P. J. A. Withers, J. J. Elser, J. Hilton, H. Ohtake, W. J. Schipper and K. C. van Dijk, Green Chem., 2015, 17, 2087–2099 RSC.
-
O. Gantner, W. Schipper and J. J. Weigand, in Sustainable Phosphorus Management, ed. R. W. Scholz, A. H. Roy, F. S. Brand, D. T. Hellums and A. E. Ulrich, Springer, Dordrecht, 2014, pp. 237–242 Search PubMed.
- D. Cordell and S. White, Sustainability, 2011, 3, 2027–2049 CrossRef.
-
S. J. van Kauwenbergh, World Phosphate Rock Reserves and Resources, 2010.
- M. Wang, C. Hu, B. B. Barnes, G. Mitchum, B. Lapointe and J. P. Montoya, Science, 2019, 365, 83–87 CrossRef CAS.
- J. Elser and E. Bennett, Nature, 2011, 478, 29–31 CrossRef CAS.
-
H. Ohtake and S. Tsuneda, Phosphorus Recovery and Recycling, Springer, 2018 and references within Search PubMed.
- J. C. Slootweg, Angew. Chem., Int. Ed., 2018, 57, 6386–6388 CrossRef CAS.
- H. Tayibi, M. Choura, F. A. Lopez, F. J. Alguacil and A. Lopez-Delgado, J. Environ. Manage., 2009, 90, 2377–2386 CrossRef CAS.
- V. N. Rychkov, E. V. Kirillov, S. V. Kirillov, V. S. Semenishchev, G. M. Bunkov, M. S. Botalov, D. V. Smyshlyaev and A. S. Malyshev, J. Clean. Prod., 2018, 196, 674–681 CrossRef CAS.
-
K. Schrödter, G. Bettermann, T. Staffel, F. Wahl, T. Klein and T. Hofmann, Ullmann's Encyclopedia of Industrial Chemistry, Wiley, Weinheim, Germany, 2008 Search PubMed.
- H. Von Plessen and G. Schimmel, Chem. Ing. Tech., 1987, 59, 772–778 CrossRef CAS.
- B. M. Cossairt, M. C. Diawara and C. C. Cummins, Science, 2009, 323, 602 CrossRef CAS.
- A. R. Jupp and D. W. Stephan, Trends Chem., 2019, 1, 35–48 CrossRef CAS and references within.
-
R. Engel, Synthesis of Carbon-Phosphorus Bonds, CRC Press, 2nd edn, 2003 Search PubMed.
- N. Weferling, S. M. Zhang and C. H. Chiang, Procedia Eng., 2016, 138, 291–301 CrossRef CAS.
- J. L. Montchamp, Acc. Chem. Res., 2014, 47, 77–87 CrossRef CAS.
-
E. D. Weil and S. V. Levchik, Flame Retardants for Plastics and Textiles: Practical Applications, Carl Hanser Verlag, Munich, 2009, p. 97 Search PubMed.
- J. E. Borger, A. W. Ehlers, J. C. Slootweg and K. Lammertsma, Chem. – Eur. J., 2017, 23, 11738–11746 CrossRef CAS and references within.
- G. Müller, M. Zalibera, G. Gescheidt, A. Rosenthal, G. Santiso-Quinones, K. Dietliker and H. Grützmacher, Macromol. Rapid Commun., 2015, 36, 553–557 CrossRef.
-
N. N. Greenwood and A. Earnshaw, Chemistry of the Elements, Butterworth-Heinemann, Boston, Mass., 2nd edn, 1997 Search PubMed.
-
J. H. Clark and D. J. Macquarrie, Handbook of Green Chemistry and Technology, John Wiley & Sons, 2002 Search PubMed.
- C. J. O'Brien, J. L. Tellez, Z. S. Nixon, L. J. Kang, A. L. Carter, S. R. Kunkel, K. C. Przeworski and G. A. Chass, Angew. Chem., Int. Ed., 2009, 48, 6836–6839 CrossRef.
- J. S. Elias, C. Costentin and D. G. Nocera, J. Am. Chem. Soc., 2018, 140, 13711–13718 CrossRef CAS.
- S. Manabe, C. M. Wong and C. S. Sevov, J. Am. Chem. Soc., 2020, 142, 3024–3031 CrossRef CAS.
- M. B. Geeson and C. C. Cummins, Science, 2018, 359, 1383–1385 CrossRef CAS.
- M. B. Geeson, P. Ríos, W. J. Transue and C. C. Cummins, J. Am. Chem. Soc., 2019, 141, 6375–6384 CrossRef CAS.
- M. B. Geeson and C. C. Cummins, ACS Cent. Sci., 2020, 6, 848–860 CrossRef CAS.
- J. M. Roberts, J. L. Placke, D. V. Eldred and D. E. Katsoulis, Ind. Eng. Chem. Res., 2017, 56, 11652–11655 CrossRef CAS.
- J. C. Slootweg, Curr. Opin. Green Sustainable Chem., 2020, 23, 61–66 CrossRef.
- T. Keijer, V. Bakker and J. C. Slootweg, Nat. Chem., 2019, 11, 190–195 CrossRef CAS.
- K. C. van Dijk, J. P. Lesschen and O. Oenema, Sci. Total Environ., 2016, 542, 1078–1093 CrossRef CAS.
- I. Dévai, L. Felföldy, I. Wittner and S. Plósz, Nature, 1988, 333, 343–345 CrossRef.
- J. S. Greaves, A. M. S. Richards, W. Bains, P. B. Rimmer, H. Sagawa, D. L. Clements, S. Seager, J. J. Petkowski, C. Sousa-Silva, S. Ranjan, E. Drabek-Maunder, H. J. Fraser, A. Cartwright, I. Mueller-Wodarg, Z. Zhan, P. Friberg, I. Coulson, E. L. Lee and J. Hoge, Nat. Astronomy, 2020 DOI:10.1038/s41550-020-1174-4.
- G. Morse, S. Brett, J. Guy and J. Lester, Sci. Total Environ, 1998, 212, 69–81 CrossRef CAS.
- P. H. Liao, W. T. Wong and K. V. Lo, J. Environ. Eng. Sci., 2005, 4, 77–81 CrossRef CAS.
- P. Cornel and C. Schaum, Water Sci. Technol., 2009, 59, 1069–1076 CrossRef CAS.
- P. Kehrein, M. van Loosdrecht, P. Osseweijer, M. Garfí, J. Dewulf and J. Posada, Environ. Sci.: Water Res. Technol., 2020, 6, 877–910 RSC.
- J. D. Doyle and S. A. Parsons, Water Res., 2002, 36, 3925–3940 CrossRef CAS.
- E. Desmidt, K. Ghyselbrecht, Y. Zhang, L. Pinoy, B. Van der Bruggen, W. Verstraete, K. Rabaey and B. Meesschaert, Crit. Rev. Environ. Sci. Technol., 2014, 45, 336–384 CrossRef.
- Crystalactor Water Technology by Royal HaskoningDHV, http://www.royalhaskoningdhv.com/en/crystalactor, (accessed 4 July 2020).
- Y. Wu, J. Luo, Q. Zhang, M. Aleem, F. Fang, Z. Xue and J. Cao, Chemosphere, 2019, 226, 246–258 CrossRef CAS.
- H. Herzel, O. Krüger, L. Hermann and C. Adam, Sci. Total Environ, 2016, 542, 1136–1143 CrossRef CAS.
- L. Egle, H. Rechberger, J. Krampe and M. Zessner, Sci. Total Environ, 2016, 571, 522–542 CrossRef CAS.
-
D. Huygens, H. Saveyn, D. Tonini, P. Eder and L. Delgado Sancho, Technical proposals for selected new fertilising materials under the Fertilising Products Regulation (Regulation (EU) 2019/1009): process and quality criteria, and assessment of environmental and market impacts for precipitated phosphate salts & derivates, thermal oxidation materials & derivates and pyrolysis & gasification materials, Publications Office of the European Union, 2019.
- O. Krüger, A. Grabner and C. Adam, Environ. Sci. Technol., 2014, 48, 11811–11818 CrossRef.
- P. J. A. Withers, K. C. van Dijk, T. S. S. Neset, T. Nesme, O. Oenema, G. H. Rubæk, O. F. Schoumans, B. Smit and S. Pellerin, Ambio, 2015, 44, S193–S206 CrossRef.
- O. F. Schoumans, F. Bouraoui, C. Kabbe, O. Oenema and K. C. van Dijk, Ambio, 2015, 44, S180–S192 CrossRef.
- E. Cascarosa, G. Gea and J. Arauzo, Renewable Sustainable Energy Rev., 2012, 16, 942–957 CrossRef CAS.
- M. Coutand, M. Cyr, E. Deydier, R. Guilet and P. Clastres, J. Hazard. Mater., 2008, 150, 522–532 CrossRef CAS.
- Rock Phosphate - Monthly Price - Commodity Prices - Price Charts, Data, and News - IndexMundi, http://www.indexmundi.com/commodities/?commodity=rock-phosphate&months=180, accessed 4 July 2020.
- S. Hukari, L. Hermann and A. Nättorp, Sci. Total Environ, 2016, 542, 1127–1135 CrossRef CAS.
- B. Garske, J. Stubenrauch and F. Ekardt, Review of European, Comparative & International Environmental Law, 2020, 29, 107–117 Search PubMed.
-
J. Von Horn and C. Sartorius, International Conference on Nutrient Recovery from Wastewater Streams Vancouver, IWA Publishing, London, 2009 Search PubMed.
Footnotes |
† Electronic supplementary information (ESI) available. See DOI: 10.1039/d0cs01150a |
‡ These two authors contributed equally. |
|
This journal is © The Royal Society of Chemistry 2021 |
Click here to see how this site uses Cookies. View our privacy policy here.