DOI:
10.1039/D1MA00329A
(Review Article)
Mater. Adv., 2021,
2, 6569-6588
The framework of nanopesticides: a paradigm in biodiversity
Received
10th April 2021
, Accepted 15th August 2021
First published on 28th September 2021
Abstract
Nanotechnology has imparted excellent substitutes in the area of agriculture for the management of insect pests without deteriorating the surrounding biota and thereby allows a more verdant environment. Particular idiosyncrasies have arisen in innovative nanoagrochemicals due to the viable applications of nanotechnology in a myriad of agricultural settings. Concerns have been raised about the need for novel products, therefore they have been predicted to have considerable potential to underpin the obligatory increase in worldwide food production in a sustainable manner. Conventional strategies like assimilated pest management employed in agriculture are inadequate and the application of chemical pesticides has pernicious impacts on the environment and mankind. There is a dire need to develop a repository of safe and propitious formulations to implement a regulatory paradigm for nanopesticides. Nanopesticides are well known in agriculture to reduce costs, improve the outcomes of agricultural products and enhance shelf life and nutrition. Much research has presented the innovation in a range of industrial domains that allows the improvement in the effective use of nanopesticides in water, nanocapsules for the delivery of biocides, nanosensors for the detection of pests, etc. Furthermore, the current consumption pattern of nanopesticides and their health repercussions has been elucidated. This much needed discussion will address the gap between the need for adequate control, environmental efficacy, associated benefits and the detrimental impacts of nanoagrochemicals.
The use of nanotechnology in agriculture
Sustainability requires high agricultural yields with the resulting environmental repercussions in terms of water utilization, the contamination of ecosystems and land treatment by agrochemicals. Nanotechnology is on the horizon to transform present industries, including food, pharmaceuticals, agriculture, medical and other related areas. Research on the applications of nanotechnology in agriculture, particularly in the production of crops, has latterly received much attention, with the fundamental aim of attaining a more reasonable utilization of resources via the development of sensors or delivery systems for agrochemicals.1,2 There is a fundamental belief that agriculture has the ability to address these issues, yet there is scant agreement on how this can be attained in a sustainable manner. World food distribution and production result in environmental contamination, changing populations and the extortionate use of energy and water. Nanotechnology has currently been suggested to improve the protection of crops in agriculture by taking corrective measures to mitigate the use of hazardous pesticides and to fabricate novel pesticide formulations to reduce their employment, with controlled delivery, to grains and plants. Nanomaterials can facilitate the destruction and detection of environmental contaminants by nanoremediation and nanosensor methods.3 The current nanotechnology tools and strategies have the potential to address the several “Gordian knots” of traditional pest management techniques which could revolutionize the agricultural domain. The lion's share of nanopesticides and nanofertilizers produced up to now encompass reformulations of currently known active ingredients to attain enhanced performances compared to those of existing active ingredients, which will address the major stumbling block of present agrochemical products.4,5 Enhanced fertilizer efficacy and the targeted delivery of active ingredients to pests are among the approaches which will help or allow enhanced yields which much reduced rates of application, which will concomitantly address the damaging implications of agriculture on human health and ecosystems. Nanoparticles are well regarded for the control and management of insect pests. They allow the management of insect pests via formulations of nanomaterials based on insecticides and pesticides. The use of nanoparticle-mediated DNA and gene editing in plants to improve the heterogeneity in insect pest resistance and agricultural efficiency improvement by utilizing encapsulated nanoparticles for the steady exposure of plants to nutrients. Inadequate conventional techniques employed in agriculture for the integrated management of pests, such as the implementation of synthetic pesticides such as DDT (dichlorodiphenyltrichloroethane) and malathion, etc., have had unpropitious impacts on mankind and animals and have resulted in a decrease in soil fertility.
It has been demonstrated that nanoagrochemicals may be excellent replacements for traditional products and much hope has been placed in the applications of nanotechnology. Nonetheless, no systematic comparison has yet been carried out on the framework of literature which has evolved over more than a decade. The increase in the global population has been estimated to reach 9.7 billion by 2050, which requires the gross production of agriculture to be increased by 60%, compared to the levels in 2005, to address the need for food, specifically in developing countries.6 Furthermore, there are ∼815 million people which are presently undernourished and it is anticipated that an additional 2 billion people will be included in this classification by 2050. These circumstances call for significant changes in the nanotechnology domain to ameliorate the needs of agriculture by providing solutions to agriculture, enhancing the efficiency of agricultural inputs and addressing environmental challenges to food safety and productivity. Pesticides are essential to fortify our crops and thereby they enhance crop yields. However, the potency of chemical pesticides is deficient because of their vulnerability and substantial binding with organic matter in soil which manifests them ineffective or impedes the accumulation of residual pesticides at the root level where several pests reside.7 Prodigious amounts are required, which results in the accumulation of pesticides in the water, soil and agricultural products.8 Continuous exposure to these chemicals is damaging to human health and threatens the biodiversity of already endangered ecosystems. 9 Therefore, changes in farming practices are required to distribute pesticides in a more effective manner.
The main emphasis of this exploration is to thoroughly analyze the properties of nanoagrochemicals compared with those of existing products, with a specific aim to focus on such properties which play a vital role in the environmental impacts and benefits associated with agrochemicals. This research aims to present the future of nanopesticides, to cast light on the impact of nanomaterials on soil systems and their translocation and uptake mechanisms.
Nanopesticides: state of the art
The understanding of nanopesticides has been thriving amidst researchers in commercial, scientific and public fields. There is a clear link between climate change and agriculture. In a pernicious negative cycle, the prevailing current agricultural practices exacerbate factors that negatively affect climate change, while the increases in environmental pressures and the influence of biophysical conditions affect both the quantity and quality of production of food, thereby causing an escalation in food instability and impeding ample nutrition and the equitable distribution of food. The use of nanotechnology has led to the development of efficient medical imaging tools and drug delivery systems, and similar revolutions are now being implemented in intelligent agrochemical delivery systems designated as “nanopesticides”. Nanopesticides represent an emerging technological development that utilizes nanotechnology for the nano-based smart formulation of pesticides to realize applications in the agricultural field. The shape and peculiar properties of nanoscale materials are engineered to explore pesticide activities in innovative nanocarrier formulations based on several materials, such as metals, ceramics, carbon, copolymers, polymers, lipids, silica, etc. The benefits of the nanoformulation of pesticides are illustrated in Fig. 1. These comprise the utilization of nanomaterials for the conjugation, encapsulation, and adsorption of pesticides to meliorate the dispersion, permeability, stability and biodegradability of the active ingredients. The encapsulation of pesticides in nanoparticles obstructs their premature degradation and the dangers of the direct release of the active ingredients to mankind. Nanopesticides have a large surface area, contrary to conventional pesticides, which improves their potential for interaction with the target pests at lower doses. It is clear that conventional pesticides and nanopesticides vary in their environmental behaviour, so the extensive investigation of the fate of nanopesticides is required to corroborate that they comply with regulatory legislation and guidelines.10,11 Nanopesticides play a significant role in curtailing the environmental footprint by reducing the use of conventional pesticides. Nanopesticides can be classified into two major categories: (a) pesticides whose coherent ingredients are at the nanoscale, which typically contain a nano dispersant emulsion of active pesticides, and (b) pesticides loaded, encapsulated, doped or coated by nanomaterials. The aforementioned type of pesticides generally combines the potential benefits of conventional pesticides while also protecting, transporting and regulating the exposure of pesticides.12–14
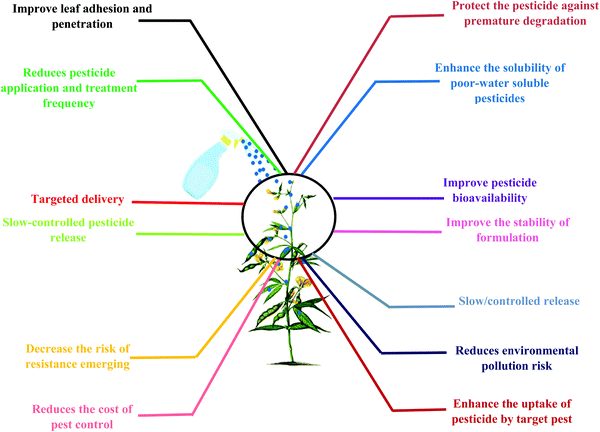 |
| Fig. 1 Why the nano-formulation of pesticides is a benefit. | |
Conventional pesticides have poor stability, lower dispersibility, coarse particles for drug carriers, lower biological activity and their employment rate is below 30%, contrary to nanopesticides.15,16 Furthermore, nanocarriers of nanopesticides can not only ameliorate the dispersibility of pesticides but also accelerate the delivery of beneficial ingredients of pesticides to the targeted organisms to enhance their bioavailability.17 These benefits reduce the shortcomings of the low efficiency and large doses of conventional pesticides and hence nanopesticides have been extensively employed. It has been shown that nanopesticides may trigger health repercussions, which have been attributed by the US-based Environmental Protection Agency to: (a) the dermal absorption of nanopesticides due to their very minuscule size and their ability to cross cell membranes, (b) they can enter the lungs and travel to the brain by crossing the blood–brain barrier, (c) the reactive potential and longevity of some specific nanomaterials, which cause environmental concerns and (d) the lack of understanding of how to measure environmental exposure to nanomaterials.18 The range of nanopesticides being fabricated comprises reformulations of well known active ingredients with fungicidal, insecticidal and herbicidal properties.19 Most active ingredients are organic molecules; apart from this, some active ingredients are inorganic, for instance copper which has been employed for its fungicidal properties. Nanocarriers are usually soft nanoparticles, such as solid lipids; polymers etc., but there are also several examples of hard nanoparticles e.g., graphene oxide, silica and carbon nanotube nanoparticles.20,21 High dosages of employed pesticides does not achieve their target and the utilization of pesticides ordinarily results in the contamination of aquatic and terrestrial environments. One of the prime impulses for the implementation of nanotechnology is to reduce the amount of pesticides required to ensure the protection of crops, which can be attained in numerous ways, for instance by enhancing the bioavailability, controlled release, apparent solubility, targeted delivery, stability, and leaf adhesion of the active ingredients in the environment. The controlled delivery of pesticides reduces the amount of pesticide utilized and prevents the development of resistant pest species that result from the contamination of water and soil and overexposure to excess chemicals. In contrary to conventional formulations, controlled delivery ensures the release of the active ingredient at a predetermined rate to attain the desired efficacy lifespan for the nanopesticides in the field. To explore the efficacy for regulatory purposes, the commercialized or registered nano-formulation is required to be compared with a conventional formulation, which is used as a reference product, to construct an appropriate investigation of its relative advantages, ideally under field conditions following the label directions for a cropping system specified by the Australian Registration Scheme. A landmark report on “diyarex gold” nanopesticides innovated using nanotechnology is prevailing in the market. It has been implemented as an antiseptic against powdery mildew and rust in several plants.22
Fate of nanopesticides
Nanopesticides can influence the photosynthetic pathway in the biochemical or photochemical phases, depending on the physiochemical properties or dosage of the nanomaterials, which therefore affects the productivity of crops. The shape and size of nanoparticles are correlated with their mechanical impact on plants, restricting the nanomaterials to some specific sites on organ surfaces or in plants. However, the effects can be manifested throughout the vegetative body, which results in the inhibition or stimulation of plant growth. In the cytoplasm of the cell, nanopesticides interact with chloroplasts and therefore influence the photosynthetic reactions at specific sites in plants by binding to the photosynthetic apparatus and obstructing its functioning. Research has shown that metallic nanoparticles may be detrimental to photosynthesis by inducing functional and structural damage.23 Therefore, comprehension of the interactions of nanopesticides with the photosynthesis mechanism could lead to an understanding of the electron transport inhibition of nanomaterials, photoinduced oxidative stress and the defense system of antioxidants in plants. For instance, 3 nm of CNTs can regulate tomato germination (Lycopersicon esculentum Mill.) owing to the effect of the water content in seeds on the entrance.24 In contrast, carbon-based nanoparticles can induce growth inhibition by mechanical damage attributed to their perforating impact on organelles or cellulose membranes, which also involves the primary mechanism of cytotoxicity.25–27 Furthermore, titanium-based nanoparticles or clay-based colloidal suspensions can affect the hydraulic conductivity of corn (Zea mays L.), which impedes the transpiration and growth of plants.28 Hence, it is imperative to analyze the role of nanoparticles in pesticide nanoformulation and the anticipated behavior resulting from this. The following conditions can be contemplated when investigating the functioning of nanoparticles:
(a) The pesticidal properties of nanoparticles, silica derived plant growth regulators and nanometals like aluminum, copper and silver fungicides or bactericides, with titanium-based oxides being prime examples.29,30
(b). The active ingredient is the major material in nanoformulation, which is bound to a nanocarrier which maintains a complex structure after spraying, followed by the release of the active ingredient in a targeted manner. Here, the pesticide fate is associated with nanoformulation that depends on the nanopesticide’s durability i.e. the duration of the association between the active ingredient and the nanocarrier. There are several nanoformulations, such as lipid-based nanomaterials (nanostructured lipid carriers and liposomes), polymer-based nanomaterials (nanogels, micelles, nanospheres and nanocapsules) and clay-based nanomaterials (layered double hydroxides and clays).31
(c). The properties of the nanoformulation are controlled to enhance the apparent dispersion/solubility of the active ingredient or preserve it from degradation. This category includes nano-dispersions and nano-emulsions. Here, the product is referred to as a conventional pesticide in the risk assessment.32,33 Consequently, the standard methodologies employed for chemicals should be adequate to examine the fate of nanopesticides.
The characterization and detection of nanomaterials in complex matrices like soil are exceedingly challenging, for instance, due to the existence of natural colloids which are almost indistinguishable from nanoparticles.34 Hence, the fate of nanopesticides in soils depends on the concentration of active ingredients. For instance, by evaluating the soil release kinetics, one may calculate the nanoformulation’s durability in water or soil solution from soil pores which are further subjected to strategies to separate free and bound pesticides. The interaction of nanomaterials with plants may be phytotoxic or positive, which also depends on the concentration and nature of the material.35–37 The dynamic interaction of nanomaterials with the surrounding environmental media and the absorption/adsorption of various moieties on particle surfaces may also lead to a change in the particle characteristics. The biotransformation of nanomaterials in the environment, resulting from their interaction with bio-films, biological organisms and plants, can also play a major role in regulating their fate, behaviour and persistence in the environment. A number of transport and fate models, including FOCUS models like TOXSWA, MACRO, PEARL, PRZM, PEARL, etc., are presently accessible for organic chemicals for different environmental compartments to aid decision-making.109,110 Additionally, it has been elucidated that the majority of environmental and physiochemical parameters that affect the fate and behaviour of nanomaterials in general are common amongst the different type of materials, for instance,
• For the soil compartment: soil type, porosity, water flow, mineral composition, pH, microbial community, temperature, amount and type of natural organic matter – in particular humic acids – and electrolytes (especially divalent cations).
• For the aqueous compartment: pH, salinity, ionic strength, dissolved (and suspended) organic matter and microorganisms.110
Readers are directed to more critical reviews for the assessment of the fate of nanopesticides that are worth considering but are beyond the scope of this study.10,19,33,49,109–111
Blueprint of next-generation nanoparticles in agriculture
Nanomaterials have potential applications in agriculture to improve the productivity of crops and to enhance soil health, which will be summarised in this segment. The ubiquitous existence of pesticides has resulted in the fabrication of pesticide resistance in insects, weeds and pathogens in the biota.38 Nanomaterials are widely employed to eradicate pollutants from contaminated soils. Due to their high aspect ratio, the smaller size of nanoparticles and their excellent reactivity, there has been an increase in the attention paid to their use to treat contaminated soil via chemical oxidation, absorption and reduction.39 Additionally, the recovery of nanomaterials for their reuse provides an opportunity to remove pollutants as it could minimize the remediation cost. Therefore, the innovation of nanomaterials that are efficient and stable and can retain their efficacy for numerous treatment cycles requires significant attention. Accordingly, the high magnetism of magnetic nanoparticles allows their easy and quick separation from the reaction medium when a magnetic field is applied, which allows their regeneration or recycling for additional treatment cycles.40–42 Several nanoparticles, such as graphene, carbon nanotubes, silver and copper, exhibit pest control functions and antibacterial properties. The proliferation in the interest into nanobiotechnology has exploited biological strategies for the fabrication of nanoparticles, utilizing algae, fungi and bacteria as well as some specific biomolecules from plants, such as lipids, proteins, carbohydrates and plant extracts, etc., by employing low cost and highly available materials and ensuring low energy consumption and minimal production of byproducts.43 The quest for green technology demonstrates the efficiency provided by the fabrication and implications of green nanomaterials in numerous fields, for instance the environment, food, agriculture, building, energy, biomedicine, etc.44–48 Nanoparticles have distinctive catalytic, physical and optoelectronic properties that facilitate improved photosynthesis, growth of plants and enhanced resistance of plants towards abiotic and biotic stress.
Nanoformulations can enhance the dispersion and solubility of lipophilic pesticides in water. Stable aqueous nanodispersions of lipophilic materials can by synthesized by employing suitable surface-active agents, thereby eradicating the utilization of noxious organic solvents and enhancing the bioavailability of pesticides on land. There are numerous types of nanopesticide based formulations, as depicted in Fig. 2. For instance, imidacloprid is an extremely efficient insecticide against various sucking insects. Furthermore, its nanoderivative is photodegradable, providing environmental benefits compared to the conventional one. Inevitably, nanoencapsulation bestows an opportunity to manage the release rate of pesticides to maintain the concentration of effective pesticides for a longer period. Also, premature degradation of pesticide protection can be overcome by using nanoencapsulation. Undoubtedly, smart nanosized delivery systems, such as multifunctional delivery systems, permit a more judicious utilization of pesticides; since the amount of pesticides needed for effective pest control is remarkably decreased, the cost associated with the production of pesticides and the waste management of pesticides is reduced and similarly the possibility of resistance developing decreases too. A perennial aim of nanoformulations is to steadily release the active ingredients, otherwise they become unstable and mobile in field applications. In similar cases, the release of active ingredients from nanocarriers is a vital approach to regulate the environmental fate of nanopesticides.49
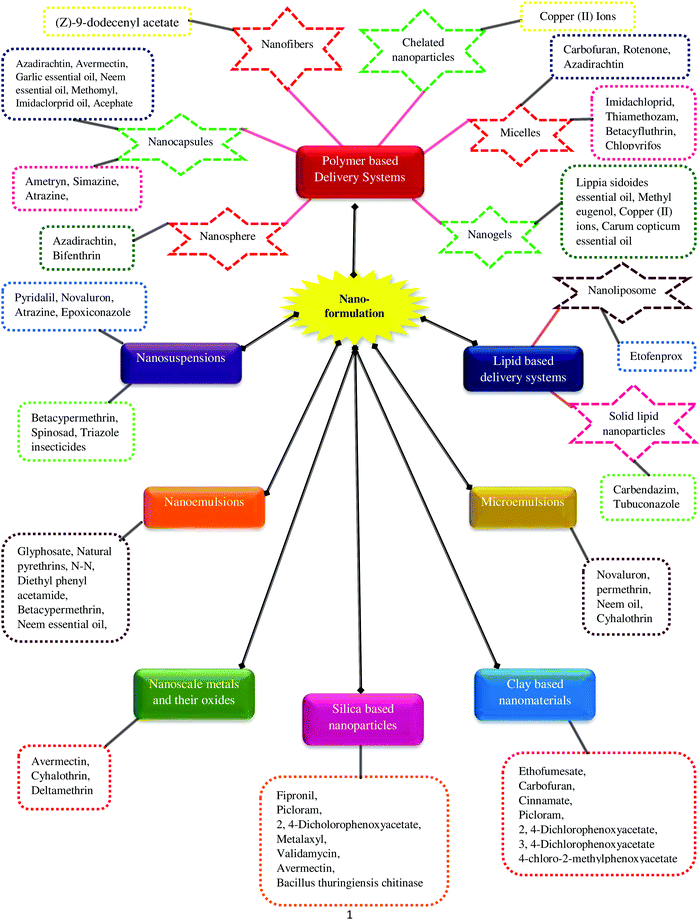 |
| Fig. 2 Delivery of synthetic and natural pesticides, outlining the different types of nanocarriers.127 | |
Carbon-based nanomaterials have various properties which make them useful in pesticides. In particular, carbon dots show self-fluorescence, which permits them to be employed for the detection of stress, in contrast to commercial biochar. Lately, significant attention has been paid to immobilization by plants as a cost-efficient and straightforward technique for the remediation of contaminated soil.50 Nanohydroxyapatite particles can immobilize metal contents in contaminated soil and segments by curtailing their exchangeable fractions, which diminishes their concentration in pore water.51,52 Nanoparticles have also been employed for the mineralization of organic pollutants and catalytic degradation in contaminated soil via an advanced oxidation process that utilized different oxidants. Iron nanoparticles, along with chelating agents, have been employed with modified Fenton oxidation for the remediation of pyrene in contaminated soil.53 The efficiency of oxidation can be varied according to the nature of the chelating agent and the maximum is attained using sodium pyrophosphate. Biotic stresses, for instance herbivore attack and pathogen infection, are crucial factors that affect crop protection. However, the utilization of pesticides has enhanced agricultural productivity and production risk. With a broad spectrum of antibacterial characteristics, silver nanoparticles have acquired conspicuous attention in the field of nanopesticides. Ocsoy and co-workers fabricated DNA directed silver nanoparticles grafted on graphene oxide which decreased the cultured activity of Xanthomonas perforans.54 This pathogenic bacterium facilitates a 10–50% reduction in yields by causing bacterial spots on tomatoes at a concentration of 16 mg l−1. Under similar conditions, Cromwell et al. fabricated silver nanoparticles that exhibited efficiency against nematodes, a common soil-borne organism. The investigated Meloidogyne spp were exposed to 30–150 mg l−1 concentrations of silver nanoparticles and it was observed that in six days 99% of the nematodes died. It was also elucidated that 150 mg l−1 of Ag nanoparticles diminished the nematodes by 82% and 92% at days 2 and 4, respectively.55 For other chemical pesticides, the production of nanoparticles with greener approaches is environmentally benign. Bacterial or plant extracts comprise numerous metabolites that play vital roles as capping and reducing agents in the synthesis of Ag nanoparticles.
Ali et al. fabricated Ag nanoparticles using Artemisia absinthium extract, which obstructed Phytophthora infection in plants and meliorates the survival of plants.56 In another study, Mishra and co-workers employed a Serratia sp, a rhizobacterium that facilitates the growth of plants, to fabricate Ag nanoparticles using a biomimetic strategy and used them against Bipolaris sorokiniana, which demonstrated excellent antifungal activity (based on a spot blotch of the wheat pathogen).57 Despite providing efficient results in agricultural settings, the potential toxicity of Ag nanoparticles in crop plants has caused apprehension. Some metal oxides, such as ZnO and TiO2 (zinc and titanium dioxide), are photochemically active. TiO2 has outstanding antimicrobial properties; when exposed to light excited electrons are developed in the presence of oxygen, which results in the formation of superoxide radicals via the direct transfer of electrons. Paret et al. investigated their antibacterial potential and remarkable photocatalytic properties against X. perforans (the pathogen which induces spot infections in tomato plants). Under a greenhouse environment, ZnO/TiO2 nanoparticles effectively decreased the bacterial spots, in contrast to copper treated and untreated controls, at a concentration of 500–800 mg l−1.58 More recently, cerium based nanoparticles have been employed by Adisa et al., who performed greenhouse analysis of the repression of Fusarium tilt in tomato plants. Tomato seedlings were exposed to cerium dioxide nanoparticles via the foliar and root pathways at concentrations of 50 and 250 mg l−1. The results demonstrated that the severity of infection was decreased by 53 and 57%, respectively, by utilizing 250 mg l−1 of CeO2 nanoparticles.59
The antifungal and antibacterial properties of copper nanoparticles are well known. Escherichia coli and Bacillus subtillis, which are bacterial pathogens, and plant fungal pathogens such as C. lunata, Fusarium oxysporum, A. alternata and P. destructiva can be treated by Cu(OH)2 nanoparticles, which act as an active ingredient in Kocide 3000 commercial pesticide.60,61 Van et al. reported the insecticidal activity of copper nanoparticles on conventional cotton and Bt-transgenic cotton. The resultant nanoparticles improved the expression of the exogenous gene in the tissues of cotton plants encoding the Bt toxin, thereby improving resistance.62 On the other hand, silica-based nanoparticles have been used for the efficient and sustained release of pesticides and the focus is on deducing the required dose to attain the desired effect. Prado et al. attempted to fabricate a nanosized efficient delivery vector for herbicide. They used picloram, in which there are abundant functional groups that act as anchor points for the attachment of pesticides. Novel silylating agents containing carboxyl moieties were developed by the reaction of chloroacetic acid with 3-amnipropyltrimethoxysilane. They proclaimed that carboxyl derived mesoporous silica nanoparticles can control the release of herbicide.63 Another study investigated the phytopathogen resistance of maize when exposed to silica nanoparticles, which facilitates the up-regulation of phenolic compounds, resulting in the higher resistance of plants towards Aspergillus spp.64 The loading capacity plays a crucial role in the performance and efficiency of nanocarriers, since high loading permits the reduction of the dose of nanocarriers required to encapsulate the number of active ingredients.
Understanding of carbon-based nanopesticides in pest management
Carbon-based nanomaterials have been contemplated by the research community mainly as active and additive compounds, but they could be employed as efficient fungicides due to their antifungal characteristics. An efficient remediation method employing carbon-based nanomaterials has the potential to be a breakthrough in the decontamination of soil. Researchers have investigated the antifungal activity of six carbon-based nanomaterials, with concentrations of 62.5, 125, 250 and 500 mg l−1, comprising activated carbon, SWCNTs (single-walled carbon nanotubes), MWCNTs (multi-walled carbon nanotubes), GO (graphene oxide), reduced GO and fullerenes, against two crucial pathogenic fungi, Fusarium graminearum and Fusarium poae, incubated for 5 and 12 h under darkness. SWCNTs, MWCNTs, GO and RGO with concentrations of 500 mg l−1 exhibited excellent antifungal properties, although activated carbon displayed no antifungal activity. The resultant carbon-based nanomaterials also showed the induction of plasmolysis and inhibition of water uptake.65 In another study, carbon dot functionalized Ag nanoparticles were fabricated via a greener approach employing lycii fructus for the development of a colorimetric sensor to regulate phoxin in fruit samples and the environment. This mechanism results in high selectivity, good recovery values (87% to 110%) and remarkable sensitivity with a low detection limit of 0.04 μM.66 Tang et al. investigated cadmium toxicity with the integration of GO in Microcystis aeruginosa by increasing the generation of reactive oxygen species.67
The utilization of fullerene nanoparticles disrupts the pathways of the transport and energy of electrons by suppressing the transcription of genes. In addition, the employment of MWCNTs is responsible for the formation of cell divisions and cell walls which affect water transport, but utilization at higher concentrations becomes toxic.68,69 A high degree of functionalized CNTs facilitates poor impacts and a considerable reduction of the growth of various plant species.70 This is per the results of Tripathi et al. on the growth of Cicer arietinum L. upon treating it with water soluble MWCNTs. The investigation resulted in a reduction in the germination rate of rye and corn plants treated with MWCNTs, but the length of the roots was increased.71 The effects of engineered carbon nanomaterials with various dimensions on the germination of rice seeds were explored, which resulted in an increase in the rate of germination with increased uptake of water for treated seeds, in contrast to those of control seeds. For instance, the exposure of zucchini plants to MWCNTs did not reveal any detrimental impacts on root elongation and the germination of seeds, although a decrease in biomass was observed during further growth in the presence of SWCNTs.72
Stipulations of polymers based nanopesticides delivery stratagems
Polymer-based nanoformulations have the prodigious potential for further growth and practical innovations have improved their colossal efficacy in contrast to conventional formulations. Polymer-based nanoformulations enable a capacious range of objectives to be attained, which makes them appropriate for protection against degradation, low solubility and the slow release of active ingredients. On entering into the soil, nanomaterials can suffer biological, chemical or physical transformations, depending on their interactions and nature, with various components of soil. Aggregation is the prime difficulty with regards to physical processes, and it occurs spontaneously when the nanoparticles enter the soil. Aggregation reduces the surface area of nanoparticles, which as a result affects their reactivity. Mineral and soil colloids, specifically iron and clay-based minerals, are viewed as efficient sinks for nanomaterials. Table 1 shows various polymer-based nanocarriers and explains the properties and applications of the resulting nanopesticides. In addition, biopolymers are excellent materials derived from natural resources and can be broadly employed as nanocarriers. They have beneficial properties such as excellent stability, biocompatibility, biodegradability nontoxicity and semi-crystallinity.73,74 The essential biopolymer is chitosan, a natural polysaccharide originating from the exoskeletons of insects or the cell walls of fungi. Chitosan-based nanocarriers have a broad range of applications in agriculture, for instance in crop protection, the growth of plants, etc. It is well understood that weeds are a significant threat to modern agriculture. Therefore, the formation of nanoherbicides using biopolymeric materials could enhance the efficiency of herbicides.
Table 1 Comparison of a range of nanoformulations as delivery systems of nanopesticides, delineating the characteristics and outcomes
Polymer |
Material |
Characteristics |
Outcome |
Ref. |
Chitosan |
Zinc nanoparticles |
Inhibitory activity against pathogenic bacteria |
Bio-reduction of silver nitrate by Pleurotus ostreatus |
75
|
Chitosan |
Copper nanoparticles |
Increased antioxidant activity, enhanced growth parameters, enzyme defense |
A biodegradable nanocomposite has been fabricated against Curvularia leaf spots in maize |
76
|
Chitosan |
Copper(II) ion nanogel |
Nanogels were produced to adsorb Cu(II) ions, which were found to be more stable |
Appropriate substrate for inhibiting the growth of Fusarium graminearum, suitable for applications in biopesticides and a pH-sensitive delivery system. |
77
|
Chitosan/Cashew gum |
Lippia sidoides
|
Nanogel loaded with Lippia sidoides oil |
In vitro release profiles exhibit sustained and slower release |
78
|
Chitosan |
Copper and zinc nanoparticles |
Nanoparticles have been tested against amylase and protease enzymes correlated with the immobilization of food |
Defense enzymes of the plant which protects them for diseases |
79
|
Polyamide 6 cellulose acetate |
Nanofibers |
Pheromones were incorporated in high concentration via electrospinning |
Release of pheromones from polymer carriers |
80
|
Chitosan-co-poly(lactic acid) |
Imidacloprid |
Imidacloprid loaded particles exhibited a sustained release process |
Lipophilic pesticide delivery for imidacloprid systems |
81
|
Chitosan |
Spinosad and permethrin |
Agrochemical loaded chitosan nanoparticles analyzed on Drosophila melanogaster under several conditions |
An excellent residual effect, used for insect pest management |
82
|
Chitosan |
Gum arabic nanoparticles |
Encapsulated geraniol was fabricated and tested for biological activity against Bemisisa tabaci. Protection against UV degradation was observed |
Potential system for utilization in insect pest management |
83
|
Polyethylene glycol |
Essential oil (peppermint and palmarosa) |
Enhanced lethal and sub-lethal impacts of essential oil against Blatella germanica L. |
Developed to control German cockroaches |
84
|
Poly(N-isopropyl acrylamide-co-methacrylic acid)methacrylic acid |
Hollow mesoporous silica nanoparticles |
Sustained protection in rice plants against Nilaparvata lugens, Effectively protects the model pesticide thiamethoxam against UV irradiation and displayed strong adhesion. |
Temperature coefficient of pesticides |
85
|
|
Zein nanoparticles/neem oil |
No phytotoxicity toward Phaseolus vulgaris. Nanoencapsulated neem oil was effective against Tetranychus urticae and Bemisia tabaci |
The nanobiopesticide has the potential to regulate agricultural pests |
86
|
Poly(lactic-co-glycolic acid) |
Nanoparticles |
The encapsulated nanoparticles had thermodynamically favorable characteristics to control disease by Phytophthora infestans employing cyzofamid. |
Suppression of infection in tomato leaves and useful in breakthrough load reduction techniques |
87
|
Starch with disulfide bridged bond |
Mesoporous silica nanoparticles |
Fabricated nano vehicles could prevent the premature release of avermectin and the degradation of active ingredients |
Superior insecticidal activity and non-toxic organic solvent employed in stimuli-responsive nanocarriers |
88
|
Poly ε-caprolactone |
Chitosan nanoparticles |
Encapsulation of thiamethoxam in polymeric nanoparticles by the solvent evaporation technique. Protection from premature degradation. The microalgae and microcrustaceans were examined to evaluate the toxicity |
Low ecotoxicity and can prevent huanglongbing disease on citrus plants |
89
|
Chitosan |
β-Cyclodextrin containing linalool and carvacrol |
The decrease in toxicity is observed upon nanoencapsulation and demonstrated insecticidal activity against Tetranychus urticae and Helicoverpa armigera |
Repellant activity and decrease in oviposition was demonstrated for mites |
90
|
Current biomimetic routes for the mitigation of soil contamination
Nano based formulations for utilization in agriculture have been synthesized using physical, biological, and chemical pathways, which lead to enhancements in the environmental toxicity, cost, cytotoxicity, etc. Therefore, concerns have been raised about the need to fabricate such formulations without using noxious compounds during synthesis. Fig. 3 illustrates the balance between concentration, size and biodegradability which affect the toxicity of nanopesticides. Green chemistry, a magic bullet in the present scenario, has resulted in many innovations and has been used in the agricultural sector. Lately, microwave irradiation and sonochemistry have gained attention as clean, green and environmentally benign strategies for the synthesis of greener nanoparticles. Anastas et al. designed a Green ChemisTREE which outlines the principles of green chemistry: atom economy, utilization of benign solvents, economic viability, waste prevention, time-saving approaches and simple instrumentation, in contrary to other techniques highlighted as improving energy efficiency.91 The production of nanomaterials using microorganisms and plants has achieved the consideration of researchers worldwide due to their environmentally friendly properties. Several plants and bacteria have been employed to develop various nanoparticles for the protection of crops. Several natural substances are well known to display pesticidal characteristics, but most of them usually require protection against premature degradation and are unstable. Several polymer-based formulations using greener strategies have already been discussed in Table 1 in various forms such as nanoparticles, nanofibers, nanogels, nanoemulsions, nanospheres, etc.
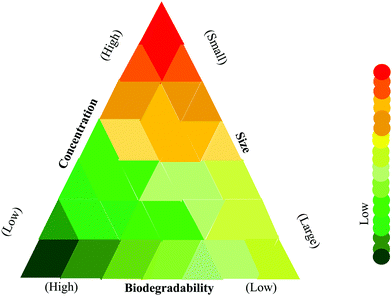 |
| Fig. 3 Balance between concentration, size and biodegradability affecting the toxicity of nanopesticides. | |
In a study on the synthesis of Ag nanoparticles, cell-free extracts of Trichoderma viride were utilized for in vivo and in vitro screening against a causative agent i.e., Alternaria solani to treat infection with blight disease in tomato plants. The antifungal results demonstrated that there was a 100% reduction in the spore count after treatment for three days. In addition, a 74% reduction in the fungal biomass was found after treatment for seven days. The effects on the foliage of the prepared nanoparticles were to facilitate a 48.57% reduction in the fungal spore count, while the pristine weight and content of the total chlorophyll of the plants increased by 32.58% and 23.52%, respectively, in comparison with those of the untreated infected plants.92 Nevertheless, although micro-organisms have been considerably studied to generate biogenic nanoparticles, there are still some gaps which need to be addressed. The reduction mechanisms that micro-organisms employ to fabricate nanoparticles are not completely understood, which makes it difficult to regulate the processes. Additionally, it is important to maintain the stability of the culture media because the reaction processes in the media can be influenced by the salinity, pH and temperature, which impede the scaling up of this to the industrial level. Moreover, the employment of plants to fabricate biogenic nanoparticles has difficulties, for instance, changes in the shape and size and less recovery results in the reduced synthetic rate of the nanoparticles. Therefore, it is required to refine the understanding of the appropriate selection of plant extracts to be used as reducing agents with a knowledge of the reduction mechanisms. To recap, it is necessary to achieve a total comprehension of the mechanisms regulating the fabrication of nanoparticles through live organisms to develop economically viable strategies for the innovation of industrially fabricated nanoparticles with suitable physicochemical characteristics, low toxicity and high biological activity.93 The aforementioned nanoformulations display inherent potential for agricultural applications and can be used to examine the maturity of crops and health conditions, discern and tune the amount of fertilizers and pesticides and sense the humidity of the soil to tailor irrigation, which eradicates the misuse of water.
Additional benefits provided by the computational modeling of nanopesticides
There has been a proliferation in endeavours to understand the release mechanisms or to evaluate whether they are regulated by desorption from the surface, erosion of the polymer structure or diffusion via the polymer matrix through computational modeling. Computational methods are in the foreground of the investigation of developed nanopesticides, which is attributed to their colossal potential to improve the understanding and innovation in environmental and (eco)toxicological analyses. Computational tools aid in reducing the time and cost required to attain authentic information to perform risk assessments and can be used alongside experimental data by delivering the necessary evidence to avoid futile experimental studies. Fig. 4 depicts how experimental data is utilized in conjunction with the outputs of computational models to assess nanopesticides. Xiang and co-workers conducted a series of experiments employing nanofibers focussed on differentiating between the degradation and diffusion controlled release mechanisms by utilizing the Higuchi equation, which has been applied in cases where the swelling of polymers and dissolution is negligible. For the in-active ingredients, the diffusion-controlled release was remarkably controlled by the nanocomposites’ hydrophobicity, which followed Fickian kinetics, although the consequent mechanism of degradation followed time-independent, zero-order kinetics. The study revealed that increasing the content of cellulose nanocrystals also increases the rate of the degradation of the fibers and the release of the active ingredients.94 Other landmark research has employed the strategy of fitting release curves utilizing mathematical models developed for the pharmaceutical industry.95 In another study, Fickian and non Fickian models have been employed for the release profiles of nanopesticides in water,96,97 which have been also employed for the estimation of genotoxicity. Ritger and Peppas designed the power model of semi-empirical power that has been extensively utilized to distinguish the type of mechanisms involved in the release of pharmaceuticals from nanosystems, which has been latterly applied for nanopesticides.98 When more than one mechanism is involved, this model has become inappropriate in conditions such as Fickian transport (diffusion combination) and non-Fickian transport (Type II, regulated by polymer chain relaxation). The exponent value gives the type of release mechanism: (a) n < 0.43 means that the mechanism is Fickian diffusion, (b) n > 0.85 means that release is regulated by the process of relaxation and (c) 0.43 < n < 0.85 reflects anomalous characteristics with the kinetics of non-Fickian release and an amalgamation of the relaxation and diffusion of polymeric chains.99,100 Some other linear models, such as first and second-order kinetic models, have also been employed. The aforementioned results demonstrated that the release of pesticide active ingredients is influenced by diffusion via the polymeric matrix, the surface desorption and the degradation of the polymeric structure. Therefore, a prodigious number of components are anticipated to exert a potential influence on the release profiles of nanopesticides in the agricultural field.
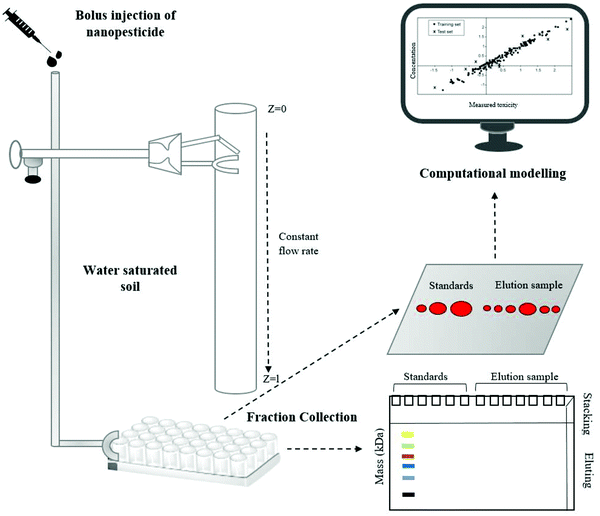 |
| Fig. 4 Computational approaches to assessing nanopesticides.116 | |
There has been a substantial upsurge of interest in quantitative structure–activity/toxicity/property relationship (QSAR/QSTR/QSPR) models lately. Viallaverde and co-workers analysed QSAR and QSPR tools for the evaluation of the biological and physiochemical effects of nanopesticides. Some of the potential modelling methods suggested for development of nano QSAR and QSPR tools include artificial neural networks, Gaussian processes, multiple linear regression and partial least squares for quantitative problems, knowledge-based expert systems, k-nearest neighbour and linear discriminant analysis for qualitative problems and principle component analysis, support vector machines and decision trees for both types of problems. The authors reported that nanopesticides have not been evaluated utilizing nano-QSAR and QSPR models so far and emphasized the inclusion of trailblazing nanodescriptors with the capacity to combine both the size-dependent and chemical attributes of nanopesticides They further suggested the correlation of biological and physiochemical end-points from theoretical and experimental nanodescriptors.112 Molecular modelling techniques, density functional theory calculations, kinetic mean field models and molecular dynamic simulations have been employed to achieve an understanding of the dynamics and interaction of nanoparticles within biological systems.113 Recently, Mishra et al. utilized molecular docking to analyse the interactions between silver nanoparticles and the digestive enzymes of termites and gut-associated microbes. The study revealed the potential prospects of fabricated nanopesticides for pest management in the agricultural and forestry sectors.114
Machine learning and artificial intelligence allow the determination of the most basic boundaries that decide and predict the behaviour of nanoparticles in soil and plant environments from enormous datasets. The utilization of mechanized information recovery from public data sets, data pre-processing and gap filling, and robotized parting of the information into test and approval sets for modelling can work with the in silico investigation of nanomaterials that can be applied to plants effectively. Nanomaterial changes in various soil conditions and distinctive rhizosphere syntheses under changing environmental conditions could likewise be predicted by incorporating prescient models, empowering the improvement of nanomaterials for horticultural applications in a range of climatic and local conditions. More extensive environment impacts and the forecasting of nanomaterials–soil–plant practices under future environmental situations can likewise be predicted utilizing, for instance, deep learning approaches and Bayesian networks. Such models are particularly significant as they can work under information shortages, yet can effectively consolidate new information as it arises. The use of these models to develop the full understanding and advancement of sustainable farming will provide significant new multifaceted bits of knowledge and ideas for ways forward.117
Toxicity: the stumbling block for nanopesticides
With the rapid expansion of nanotechnology, there is trepidation concerning their plausible entry into the food chain and the ensuing bioaccumulation of manufactured nanomaterials. However, the dangerous impacts caused by the toxicity of pesticides and nanomaterials on living organisms are still poorly understood. Therefore, we have to investigate these concerns because the inherent aftermath on humans and biota is life-changing. Toxicity is the crucial element in the efficacy of pesticides and is correlated to their action against the target organisms. Toxicity is a consequence of the stabilizers, reducing agents and organic solvents employed to circumvent the agglomeration of undesirable colloids. The employment of nanomaterials is not innately risky, for example, traditional foods harbor innumerable nanoscale materials including proteins in milk, fat globules in mayonnaise, carbohydrates, DNA, etc. However, the use of some designed nanoscale materials in agriculture, water and food may prove to be detrimental to human health and the environment.115 Hence, there is an urgent need to innovate and develop techniques for the non-hazardous, efficient and economically viable production of nanoscale compounds to accelerate their utilization in agriculture.
Some studies have reported increased pesticidal toxicity at extremely low concentrations of nanopesticides, in contrary to traditional formulations. It has been suggested that the toxicity of nanopesticides was much higher, close to nearly an order of magnitude higher, when compared to conventional formulations, although analysis could not be compared across several pests or host organisms.101,102 Sasson et al. reported that nanopesticides exhibited higher toxicity toward target organisms compared to those of to commercial products, which focussed on the need for the accurate comparison of nanopesticides with authentic existing conventional equivalents.103 The variability in toxicity among conventional formulations is not peculiar, but it ordinarily remains enigmatic, attributed to the formulations and ingredient processes being confidential and proprietary. In another study, the activity of nanopesticides was compared with those of the pristine active ingredients with commercial formulations. Such investigations are required to distinguish the effect of nanoformulations in opposition to classical formulations by considering the toxicity of fungicides and insecticides. Therefore, the products fabricated to regulate the release behaviour of the active ingredients require study over an adequate time frame as the time period of the investigation was shown to influence their activity.104Fig. 5 illustrates the life cycle of nanopesticides.
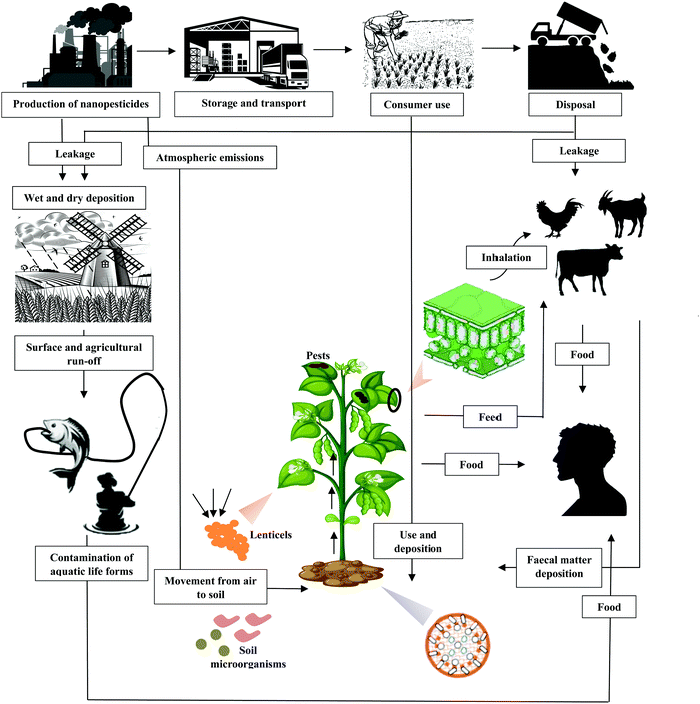 |
| Fig. 5 The life cycle of nanopesticides. | |
Environmental repercussions
The behavior and fate of nanopesticides during their application in the environment are the pivotal determinants of their impact on human health and the ecosystem. The perennial objective of nanoformulations is to steadily release the active ingredients or else they can become too unstable and mobile in the agricultural field. In the aforementioned conditions, the release of active ingredients from nanocarrier systems is an essential process that controls the environmental fate of nanopesticides. The inefficiency of the application and delivery of pesticides is also a Gordian knot. It has been observed that 10 to 75% of pesticides do not reach the selected target. The issues related to the employment of pesticides include their toxicity to non-target organisms, their persistence, the development of resistance and their accumulation in environmental spaces (Fig. 6).
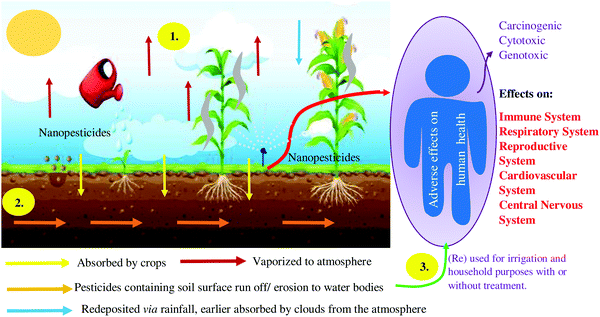 |
| Fig. 6 Nanopesticide drift and the adverse consequences that could lead to contamination of (1) the air, (2) land and (3) water. | |
If nanotechnology could enhance crop nutrition, fertilization and the efficiency of irrigation, there would be huge related benefits triggered by the reduced environmental pressure associated with the production of crops. The quantification of unbound dissolved ions of dormant noxious micronutrients is necessary to appropriately analyze the impact of nanopesticides in contrast with the effects conventional products. Such investigations these days are thought to be crucial for the environmental assessment risk of nanomaterials. Agriculture is in dire need of a revolution to meet the expanding demand for food while eradicating its impact on biota. It is paramount that essential research efforts are imparted for the innovation of new products that are highly competitive and can make future agriculture more sustainable. The responsible implementation of nanotechnology can optimistically play a pertinent role in attaining this objective.
Current consumption patterns and health impacts
The consumption of pesticides does have a socio-economic impact on the economy as a whole. They are certainly helpful in improving overall crop productivity; the demand for pesticides is continuously on the rise and currently stands at 4.19 million tonnes annually, as per the data collected by the FAO (Food and Agriculture Organisation of the United Nations). However, the associated health hazards associated with their consumption cannot be ignored. In a global comparative analysis† of the consumption of pesticides over the years vis-à-vis their gradual impact on the health of the population, it could be deduced that for the world overall (Fig. 7(g)), the growth in the consumption of pesticides has contributed to the deterioration of health, with the share of the population with cancer or the presence of neoplasms rising over the years. Country-wise, it could be deduced that in countries like China (Fig. 7(b)) and Russia (Fig. 7(c)), the consumption of pesticides has certainly taken a toll on the health of the population. In other words, the percentage of the population suffering from cancer or the presence of neoplasms in the population has been rising over the years in these regions. In such situations, the deployment of smart nanotechnology could be useful in mitigating the adverse healthcare hazards associated with the use of pesticides. In the case of advanced regions like the European Union (Fig. 7(e)) and the USA (Fig. 7(d)) as well, the rising consumption of pesticides over this time has led to a significant adverse impact on the health of the population. In the case of the former region, the share of its population with cancer has been rising consistently since 2005 onwards and has flattened since 2015. In the case of the latter country, the percentage of the population suffering with cancer shows a steadily rising trend from 2003 onwards. However, in a country like Australia (Fig. 7(a)), the growing consumption of pesticides is unlikely to have harmed the health of the population in this country. In the case of an emerging economy like the primarily agrarian India, the consumption of pesticides was the highest during the structural transformation period of the 1990s and gradually reduced during the millennium decade starting in the year 2000. Eventually, the consumption pattern has risen from the year 2013 onwards and has stabilized eventually. However, the correlation between the consumption trend and the adverse impact on health is not quite evident from the data, although the share of the population with cancer has been rising over the years in the country.
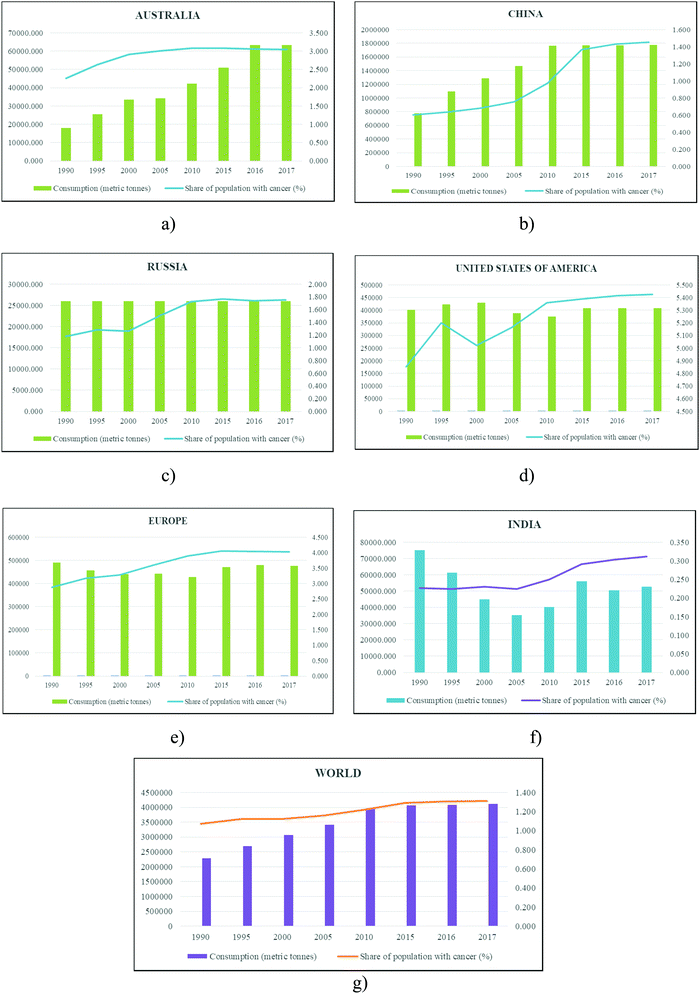 |
| Fig. 7 Country-wise and world trends (pesticide consumption and health impact). Source: authors’ analysis of data collected from ref. 105and 106. | |
The positive effects of nanoscience or nanotechnology in such countries could be a possibility. Nanotechnology has emerged as one of the most promising technologies of recent times. At nano-dimensions, the quantum confinement of electronic states results in unusually exceptional properties and thus ameliorates the issues of conventional bulk materials.105 Globally, it is estimated that the nanotechnology market is expected to exceed US$ 125 billion106 as it continues to have an impact on nearly all sectors of the globalized world, such as information and communication technology, electronics, energy, cosmetics, automotives, agriculture, etc. As an agrarian country, India, operating at a much larger agricultural scale, is set to become a country with a larger share of demand for such technologically advanced products related to farming.
In the case of India's agricultural sector specifically, productivity and food security could be directly linked to the application of nanopesticides. Fig. 8 demonstrates the various applications of nanotechnology in the agricultural sector.
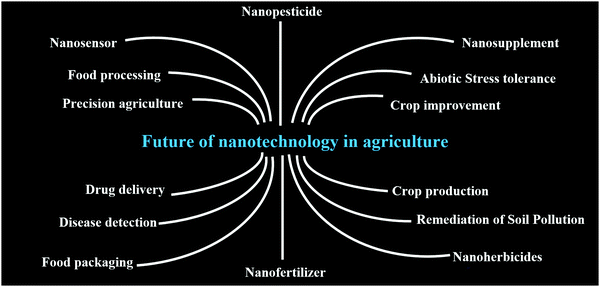 |
| Fig. 8 Application of nanotechnology in agriculture. Source: Islam (2019).107 | |
The excessive use of pesticides and their impact on health and the environment has resulted in demand for technologically advanced and safe solutions to the current agricultural formulations. In such situations, the deployment of smart nanotechnology solutions could be useful in mitigating the adverse health hazards associated with the excessive use of conventional pesticides. At present, nanopesticides are designed either as nano-sized particles of organic and inorganic constituent species as the active ingredients (AIs) in nanopesticide formulations or in the form of small engineered nanostructures having the pesticide as the active ingredient. Nanoscale formations are seen as potential candidates for bringing about a change in addressing the problems associated with bulk pesticides, especially in terms of their high efficacy and the reduction in the use of huge quantities of pesticides, however, the field is still in its infancy. Toxicity to humans and other non-target organisms, along with possible environment impacts, are the major challenges for the potential large scale applications of these nanopesticides. Thus, a word of caution is needed when it comes to the consideration of large scale applications of nanopesticides. More importantly, developing a robust regulatory framework for designing application protocols by analysing the potential risks associated with these products requires much more research effort from the scientific community because otherwise it may prove to be a major threat to food security globally. Thus, in order to overcome the challenges and major stumbling blocks in the way of large scale applications, we need various safer-by-design technologically advanced agrochemical systems with global applicability.
Natural compounds and biological systems are also viewed as interesting alternatives to conventional pesticides. Natural compounds and other biologically derived species have immense potential for use as safe and environmentally friendly agrochemicals, requiring only low doses, and they are obtainable from living systems as renewable resources, thus, they do not require much screening and have immense societal acceptability, which has attracted enormous interest. The subject has been reviewed at length in a recent study.118 Pascoli and co-workers evaluated the toxicity of neem-oil based nanopesticides towards non-target organisms, utilizing Allium cepa, soil nitrogen cycle microbiota and Caenorhabditis elegans in order to develop a safer-by-design strategy. Propitious results in the toxicity studies opened up perspectives for the use of nanobiopesticides to contribute to sustainable agriculture and improved food safety.119 Recently, Zhao and co-workers utilized carboxymethyl cellulose and rosin, two cheap and widely available biodegradable natural resources, for the synthesis of amphiphilic nanocarriers for the encapsulation of hydrophobic pesticides. It was inferred that the use of natural materials improved the long-term insecticidal effect of the pesticide, as well as reducing the harm to aquatic animals endangered by the immoderate utilization of organic solvents in conventional pesticides.120 These naturally derived agrochemical systems are, however, constrained by many limitations as compared to their synthetic counterparts in terms of their low effectiveness, complex matrix chemical structures, low persistence, higher extraction cost, inability to penetrate the applied plant forms, inherent instability and much higher biodegradability. Nanotechnology, with special reference to engineered nanoscale devices, can effectively be used to address the limitations of these natural compounds based pesticide formulations by enhancing their efficacy and stability while still maintaining their safety aspects in relation to the environment and other non-target organisms. The success of such technologies will be inherently dependent upon the safety of these designed systems in relation to the environment and other organisms.
RNA interference (RNAi) technology has been explored for the last two decades and provides another very interesting and novel mechanism for effective crop disease control and the field of pest management. The application of this technology is based on introducing double stranded RNA (dsRNA) or small interfering RNA (siRNA) with the objective of silencing target genes in plants or plant dwelling organisms by way of their direct application as pesticides. Progress in this area is expected to bring a new generation of plant protection agrochemical systems in addition to creating new plant varieties. The RNA interference mechanism is present in all eukaryotic organisms and is triggered by the cellular uptake of dsRNA molecules which are homologous to a target gene in the pest or pathogen. These dsRNAs are then processed by the RNAi pathway within the cell, which eventually leads to a depletion of messenger RNAs (mRNAs) and proteins which are encoded by that particular target gene. As in the case of other prevailing technologies, the RNAi technique requires comprehensive evaluation in relation to risk assessment frameworks and application protocols.121,122 The COST Action iPlanta, one of the largest network of European scientists, has published the outcomes of very important study aimed to discuss the risk assessment of RNAi plants and crops in relation to target and non-target organisms and the environment.123 Bioinformatics may prove to be of immense help in carrying out such assessments of these RNAi plants by way of comparing the genomes of target and non-target organisms upon exposure to interfering RNA. Although RNAi technology has enormous potential to present the next generation of agrochemical solutions in crop protection and pest management, the instability or rapid degradation of bare assayed dsRNA is a major challenge in the way of its practical utility in farming.
Nanotechnology, as a fundamental core platform, can be put to use to help design innovative nanoscale carriers for dsRNA while simultaneously preventing dsRNA degradation, especially technology that would not generate environmental damage. A number of recent studies have been dedicated to designing potentially viable nano carriers in this area.124–126 It may be noted here that, in contrast to other techniques, dsRNA technology brings specific solutions to specific problems in relation to pest management as the silencing of a particular gene is needed for a specific pest related problem.
Thus, we need to employ a complex mix of strategies in terms of nanotechnology and biotechnology for safer-by-design agrochemical solution systems, preferably based on natural products.108 In this way, we can actively contribute to transforming scientific and technological excellence into high economic returns in the form of new sustainable, eco-friendly and cost effective products, production processes and technological advancements to address the needs of the agricultural industry. Hence, it is important to maintain a concerted focus and efforts towards the development and application of technologically advanced agrochemicals through active investment and funding, coupled with government initiatives.
Conclusion and the way forward
Sustainable agriculture is a pivotal part of the move to address the proliferation in the demand for food for a swiftly growing global population. The work of researchers has increased to develop nanopesticides that are less threatening to the biota, in contrast with traditional formulations, and subsequent investigations will be needed to evaluate whether any potential developed materials can keep pace with existing formulations, in terms of their performance and cost. Therefore, innovative pathways and strategies that prevent damage to plants and ameliorate the efficiency of agrochemicals employed to attain food must proceed cautiously and sustainably. A huge amount of research will therefore be required in the near future and will entail (a) the assessment of the durability and bioavailability of nanopesticides, (b) the innovation of avant-garde protocols to foster properties for their reliable fate and (c) analysis of the current assessment of environmental risk strategies and their rectification where required. Additionally, there is an epoch-making need for a better conception of the mechanisms regulating the adverse, as well as the favourable, responses affecting the employment of nanoformulations under biotic and abiotic stressors. The behaviour of nanomaterials should also be examined in bioremediation to develop integrated remediation approaches. As an industry with a focus on profits, agricultural merchandising has intrinsic limitations that must be prudently contemplated to allow their future use, as well as public understanding and acceptance.
Conflicts of interest
The authors declare that there are no conflicts of interest.
Acknowledgements
The authors would like to pay gratitude towards the Department of Chemistry, Netaji Subhas University of Technology, Delhi, India.
References
- J. Yin, Y. Wang and L. M. Gilbertson, Opportunities to advance sustainable design of nano-enabled agriculture identified through a literature review, Environ. Sci.: Nano, 2018, 5(1), 11–26 RSC.
- S. M. Rodrigues, P. Demokritou, N. Dokoozlian, C. Ogilvie Hendren, B. Karn, M. S. Mauter and O. A. Sadik,
et al., Nanotechnology for sustainable food production: promising opportunities and scientific challenges., Environ. Sci.: Nano, 2017, 4(4), 767–781 RSC.
- I. Iavicoli, V. Leso, D. H. Beezhold and A. A. Shvedova, Nanotechnology in agriculture: Opportunities, toxicological implications, and occupational risks, Toxicol. Appl. Pharmacol., 2017, 329, 96–111 CrossRef CAS PubMed.
- M. Kah, S. Beulke, K. Tiede and T. Hofmann, Nanopesticides: state of knowledge, environmental fate, and exposure modeling, Crit. Rev. Environ. Sci. Technol., 2013, 43, 1823–1867 CrossRef CAS.
- M. Kah and T. Hofmann, Nanopesticide research: current trends and future priorities, Environ. Int., 2014, 63, 224–235 CrossRef CAS PubMed.
- N. D. Mueller, J. S. Gerber, M. Johnston, D. K. Ray, N. Ramankutty and J. A. Foley, Closing yield gaps through nutrient and water management, Nature, 2012, 490(7419), 254–257 CrossRef CAS PubMed.
- C. Lamberth, S. Jeanmart, T. Luksch and A. Plant, Current challenges and trends in the discovery of agrochemicals, Science, 2013, 341(6147), 742–746 CrossRef PubMed.
-
D. Atwood and C. Paisley-Jones, Pesticides industry sales and usage: 2008–2012 Market Estimates, US Environmental Protection Agency, Washington, DC, 2017, vol. 20460 Search PubMed.
- C. A. Damalas and I. G. Eleftherohorinos, Pesticide exposure, safety issues, and risk assessment indicators., Int. J. Environ. Res. Public Health, 2011, 8(5), 1402–1419 CrossRef CAS PubMed.
- M. Kah, R. S. Kookana, A. Gogos and T. D. Bucheli, A critical evaluation of nanopesticides and nanofertilizers against their conventional analogues, Nat. Nanotechnol., 2018, 13(8), 677–684 CrossRef CAS PubMed.
- M. Kah, H. Walch and T. Hofmann, Environmental fate of nanopesticides: durability, sorption and photodegradation of nanoformulated clothianidin, Environ. Sci.: Nano, 2018, 5(4), 882–889 RSC.
- L. C. Jiang, M. Basri, D. Omar, M. B. A. Rahman, A. B. Salleh, R. N. Z. R. A. Rahman and A. Selamat, Green nano-emulsion intervention for water-soluble glyphosate isopropylamine (IPA) formulations in controlling Eleusine indica (E. indica), Pestic. Biochem. Physiol., 2012, 102(1), 19–29 CrossRef CAS.
- A. B. Jindal, S. B. Sagar and V. D. Padma,
In situ hybrid nano drug delivery system (IHN-DDS) of antiretroviral drug for simultaneous targeting to multiple viral reservoirs: An in vivo proof of concept, Int. J. Pharm., 2017, 521(1–2), 196–203 CrossRef CAS PubMed.
- N. Chaudhry, S. Dwivedi, V. Chaudhry, A. Singh, Q. Saquib, A. Azam and J. Musarrat, Bio-inspired nanomaterials in agriculture and food: Current status, foreseen applications and challenges, Microb. Pathog., 2018, 123, 196–200 CrossRef CAS PubMed.
- Y. Tong, L. Shao, X. Li, J. Lu, H. Sun, S. Xiang, Z. Zhang, Y. Wu and X. Wu, Adhesive and stimulus-responsive polydopamine-coated graphene oxide system for pesticide-loss control, J. Agric. Food Chem., 2018, 66(11), 2616–2622 CrossRef CAS PubMed.
- Y. Sun, J. Liang, L. Tang, H. Li, Y. Zhu, D. Jiang, B. Song, M. Chen and G. Zeng, Nano-pesticides: A great challenge for biodiversity?, Nano Today, 2019, 28, 100757 CrossRef.
- S. Sharma, S. Singh, A. K. Ganguli and V. Shanmugam, Anti-drift nano-stickers made of graphene oxide
for targeted pesticide delivery and crop pest control, Carbon, 2017, 115, 781–790 CrossRef CAS.
- M. Ragaei and A-kazafy H Sabry, Nanotechnology for insect pest control., Int. J. Environ. Sci. Technol., 2014, 3(2), 528–545 Search PubMed.
- G. W. Walker, R. S. Kookana, N. E. Smith, M. Kah, C. L. Doolette, P. T. Reeves, W. Lovell, D. J. Anderson, T. W. Turney and D. A. Navarro, Ecological risk assessment of nano-enabled pesticides: a perspective on problem formulation, J. Agric. Food Chem., 2018, 66(26), 6480–6486 CrossRef CAS PubMed.
- D. Wibowo, C.-X. Zhao, B. C. Peters and A. P. J. Middelberg, Sustained release of fipronil insecticide in vitro and in perm from biocompatible silica nanocapsules, J. Agric. Food Chem., 2014, 62(52), 12504–12511 CrossRef CAS PubMed.
- L. Cao, Z. Zhou, S. Niu, C. Cao, X. Li, Y. Shan and Q. Huang, Positive-charge functionalized mesoporous silica nanoparticles as nanocarriers for controlled 2,4-dichlorophenoxy acetic acid sodium salt release, J. Agric. Food Chem., 2018, 66(26), 6594–6603 CrossRef CAS PubMed.
-
A. Bhattacharyya, P. Duraisamy, M. Govindarajan, A. A. Buhroo and R. Prasad, Nano-biofungicides: emerging trend in insect pest control, Advances and applications through fungal nanobiotechnology, Springer, Cham, 2016, pp. 307–319 Search PubMed.
- R. Tighe-Neira, E. Carmora, G. Recio, A. Nunes-Nesi, M. Reyes-Diaz, M. Alberdi, Z. Rengel and C. Inostroza-Blancheteau, Metallic nanoparticles influence the structure and function of the photosynthetic apparatus in plants, Plant Physiol. Biochem., 2018, 130, 408–417 CrossRef CAS PubMed.
- M. Khodakovskaya, E. Dervishi, M. Mahmood, Y. Xu, Z. Li, F. Watanabe and A. S. Biris, Carbon nanotubes are able to penetrate plant seed coat and dramatically affect seed germination and plant growth., ACS Nano, 2009, 3(10), 3221–3227 CrossRef CAS PubMed.
- X. Hu, K. Lu, L. Mu, J. Kang and Q. Zhou, Interactions between graphene oxide and plant cells: Regulation of cell morphology, uptake, organelle damage, oxidative effects and metabolic disorders, Carbon, 2014, 80, 665–676 CrossRef CAS.
- O. Zaytseva and G. Neumann, Carbon nanomaterials: production, impact on plant development, agricultural and environmental applications, Chem. Biol. Technol. Agric., 2016, 3(1), 17 CrossRef.
- K. L. Chen and G. D. Bothun, Nanoparticles meet cell membranes: probing nonspecific interactions using model membranes, Environ. Sci. Technol., 2014, 873–880 CrossRef CAS PubMed.
- S. Asli and M. N. Peter, Colloidal suspensions of clay or titanium dioxide nanoparticles can inhibit leaf growth and transpiration via physical effects on root water transport, Plant, Cell Environ., 2009, 32(5), 577–584 CrossRef CAS PubMed.
- M. Kah, A.-K. Weniger and T. Hofmann, Impacts of (nano) formulations on the fate of an insecticide in soil and consequences for environmental exposure assessment, Environ. Sci. Technol., 2016, 50(20), 10960–10967 CrossRef CAS PubMed.
- C. G. Athanassiou, N. G. Kavallieratos, G. Benelli, D. Losic, P. Usha Rani and N. Desneux, Nanoparticles for pest control: current status and future perspectives, J. Pest. Sci., 2018, 91(1), 1–15 CrossRef.
- M. D. Nuruzzaman, M. M. Rahman, Y. Liu and R. Naidu, Nanoencapsulation, nano-guard for pesticides: a new window for safe application, J. Agric. Food Chem., 2016, 64(7), 1447–1483 CrossRef CAS PubMed.
- M. Kah, S. Beulke, K. Tiede and T. Hofmann, Nanopesticides: state of knowledge, environmental fate, and exposure modeling, Crit. Rev. Environ. Sci. Technol., 2013, 43, 1823–1867 CrossRef CAS.
-
R. López-Cabeza, Methods for Understanding the Fate of Nanopesticides in Soil and Water, Nanopesticides, 2020111–136 Search PubMed.
- M. Hassellöv, W. R. James, J. F. Ranville and K. Tiede, Nanoparticle analysis and characterization methodologies in environmental risk assessment of engineered nanoparticles, Ecotoxicology, 2008, 17(5), 344–361 CrossRef PubMed.
-
M. H. Siddiqui, H. A.-W. Mohamed, M. Firoz and M. Y. Al-Khaishany, Role of nanoparticles in plants, Nanotechnology and Plant Sciences, Springer, Cham, 2015, pp. 19–35 Search PubMed.
- F. Aslani, S. Bagheri, N. M. Julkapli, A. S. Juraimi, F. S. G. Hashemi and A. Baghdadi, Effects of engineered nanomaterials on plants growth: an overview, Sci. World J., 2014, 641759 Search PubMed.
-
P.-H. Hermes, M.-P. Gabriela, V.-R. Ileana, C. Fusaro, L.-V. Fernando, M.-A. Mariana, C. Padilla-Rodríguez and F.-L. Fabián, Carbon Nanotubes as Plant Growth Regulators: Prospects, Green Nanoparticles, Springer, Cham, 2020, pp. 77–115 Search PubMed.
- M. Rai and A. Ingle, Role of nanotechnology in agriculture with special reference to management of insect pests, Appl. Microbiol. Biotechnol., 2012, 94(2), 287–293 CrossRef CAS PubMed.
- F. D. Guerra, F. A. Mohamed, D. C. Whitehead and F. Alexis, Nanotechnology for environmental remediation: materials and applications, Molecules, 2018, 23(7), 1760 CrossRef PubMed.
- M. Usman, S. Martin, N. Cimetiere, S. Giraudet, V. Chatain and K. Hanna, Sorption of nalidixic acid onto micrometric and nanometric magnetites: Experimental study and modeling, Appl. Surf. Sci., 2014, 299, 136–145 CrossRef CAS.
- Z. Ajmal, A. Muhmood, M. Usman, S. Kizito, J. Lu, R. Dong and S. Wu, Phosphate removal from aqueous solution using iron oxides: adsorption, desorption and regeneration characteristics, J. Colloid Interface Sci., 2018, 528, 145–155 CrossRef CAS PubMed.
- H. Fu, Y. Yang, R. Zhu, J. Liu, M. Usman, Q. Chen and H. He, Superior adsorption of phosphate by ferrihydrite-coated and lanthanum-decorated magnetite, J. Colloid Interface Sci., 2018, 530, 704–713 CrossRef CAS PubMed.
- P. Kaur, Biosynthesis of nanoparticles using eco-friendly factories and their role in plant pathogenicity: a review, Biotechnol. Res. Innov., 2018, 2(1), 63–73 CrossRef.
- Y. Lu and S. Ozcan, Green nanomaterials: On track for a sustainable future, Nano Today, 2015, 10(4), 417–420 CrossRef CAS.
- H. Barabadi, B. Tajani, M. Moradi, K. D. Kamali, R. Meena, S. Honary, M. A. Mahjoub and M. Saravanan, Penicillium family as emerging nanofactory for biosynthesis of green nanomaterials: a journey into the world of microorganisms, J. Cluster Sci., 2019, 1–14 Search PubMed.
- R. G. Saratale, I. Karuppusamy, G. Dattatraya Saratale, A. Pugazhendhi, G. Kumar, Y. Park, G. S. Ghodake, R. Naresh Bharagava, J. R. Banu and H. S. Shin, A comprehensive review on green nanomaterials using biological systems: Recent perception and their future applications, Colloids Surf., B, 2018, 170, 20–35 CrossRef CAS PubMed.
- K. W. Tan, S. K. Heo, M. L. Foo, I. M. L. Chew and C. K. Yoo, An insight into nanocellulose as soft condensed matter: Challenge and future prospective toward environmental sustainability, Sci. Total Environ., 2019, 650, 1309–1326 CrossRef CAS PubMed.
- A. Khan, Y. Wen, T. Huq and Y. Ni, Cellulosic nanomaterials in food and nutraceutical applications: a review, J. Agric. Food Chem., 2018, 66(1), 8–19 CrossRef CAS PubMed.
- R. S. Kookana, A. B. A. Boxall, P. T. Reeves, R. Ashauer, S. Beulke, Q. Chaudhry and G. Cornelis,
et al., Nanopesticides: guiding principles for regulatory evaluation of environmental risks, J. Agric. Food Chem., 2014, 62(19), 4227–4240 CrossRef CAS PubMed.
- Y. Hamid, L. Tang, M. I. Sohail, X. Cao, B. Hussain, M. Z. Aziz, M. Usman, Z.-L. He and X. Yang, An explanation of soil amendments to reduce cadmium phytoavailability and transfer to food chain, Sci. Total Environ., 2019, 660, 80–96 CrossRef CAS PubMed.
- Z. Zhang, M. Li, W. Chen, S. Zhu, N. Liu and L. Zhu, Immobilization of lead and cadmium from aqueous solution and contaminated sediment using nano-hydroxyapatite, Environ. Pollut., 2010, 158(2), 514–519 CrossRef CAS PubMed.
- A. Dong, X. Ye, H. Li, Y. Zhang and G. Wang, Micro/nanostructured hydroxyapatite structurally enhances the immobilization for Cu and Cd in contaminated soil, J. Soils Sediments, 2016, 16(8), 2030–2040 CrossRef CAS.
- S. Jorfi, A. Rezaee, G.-A. Moheb-ali and N. A. Jaafarzadeh, Pyrene removal from contaminated soils by modified Fenton oxidation using iron nano particles, J. Environ. Health Sci. Eng., 2013, 11(1), 17 CrossRef PubMed.
- I. Ocsoy, M. L. Paret, M. A. Ocsoy, S. Kunwar, T. Chen, M. You and W. Tan, Nanotechnology in plant disease management: DNA-directed silver nanoparticles on graphene oxide as an antibacterial against Xanthomonas perforans, ACS Nano, 2013, 7(10), 8972–8980 CrossRef CAS PubMed.
- W. A. Cromwell, J. Yang, J. L. Starr and Y.-K. Jo, Nematicidal effects of silver nanoparticles on root-knot nematode in bermudagrass, J. Nematol., 2014, 46(3), 261 CAS.
- M. Ali, B. Kim, K. D. Belfield, D. Norman, M. Brennan and G. S. Ali, Inhibition of Phytophthora parasitica and P. capsici by silver nanoparticles synthesized using aqueous extract of Artemisia absinthium, Phytopathology, 2015, 105(9), 1183–1190 CrossRef CAS PubMed.
- S. Mishra, B. R. Singh, A. Singh, C. Keswani, A. H. Naqvi and H. B. Singh, Biofabricated silver nanoparticles act as a strong fungicide against Bipolaris sorokiniana causing spot blotch disease in wheat, PLoS One, 2014, 9(5), e97881 CrossRef PubMed.
- M. L. Paret, G. E. Vallad, D. R. Averett, J. B. Jones and S. M. Olson, Photocatalysis: effect of light-activated nanoscale formulations of TiO2 on Xanthomonas perforans and control of bacterial spot of tomato, Phytopathology, 2013, 103(3), 228–236 CrossRef CAS PubMed.
- I. O. Adisa, V. L. Reddy Pullagurala, S. Rawat, J. A. Hernandez-Viezcas, C. O. Dimkpa, W. H. Elmer, J. C. White, J. R. Peralta-Videa and J. L. Gardea-Torresdey, Role of cerium compounds in Fusarium wilt suppression and growth enhancement in tomato (Solanum lycopersicum), J. Agric. Food Chem., 2018, 66(24), 5959–5970 CrossRef CAS PubMed.
- K.-Y. Yoon, J. H. Byeon, J.-H. Park and J. Hwang, Susceptibility constants of Escherichia coli and Bacillus subtilis to silver and copper nanoparticles, Sci. Total Environ., 2007, 373(2–3), 572–575 CrossRef CAS PubMed.
- P. Kanhed, S. Birla, S. Gaikwad, A. Gade, A. B. Seabra, O. Rubilar, N. Duran and M. Rai, In vitro antifungal efficacy of copper nanoparticles against selected crop pathogenic fungi, Mater. Lett., 2014, 115, 13–17 CrossRef CAS.
- N. Le Van, C. Ma, J. Shang, Y. Rui, S. Liu and B. Xing, Effects of CuO nanoparticles on insecticidal activity and phytotoxicity in conventional and transgenic cotton, Chemosphere, 2016, 144, 661–670 CrossRef PubMed.
- A. G. S. Prado, A. O. Moura and A. R. Nunes, Nanosized silica modified with carboxylic acid as support for controlled release of herbicides, J. Agric. Food Chem., 2011, 59(16), 8847–8852 CrossRef CAS PubMed.
- Q. Zhang, M. Liu and J. Ruan, Metabolomics analysis reveals the metabolic and functional roles of flavonoids in light-sensitive tea leaves, BMC Plant Biol., 2017, 17(1), 64 CrossRef PubMed.
- X. Wang, X. Liu, J. Chen, H. Han and Z. Yuan, Evaluation and mechanism of antifungal effects of carbon nanomaterials in controlling plant fungal pathogen, Carbon, 2014, 68, 798–806 CrossRef CAS.
- M. Zheng, C. Wang, Y. Wang, W. Wei, S. Ma, X. Sun and J. He, Green synthesis of carbon dots functionalized silver nanoparticles for the colorimetric detection of phoxim, Talanta, 2018, 185, 309–315 CrossRef CAS PubMed.
- Y. Tang, J. Tian, S. Li, C. Xue, Z. Xue, D. Yin and S. Yu, Combined effects of graphene oxide and Cd on the photosynthetic capacity and survival of Microcystis aeruginosa, Sci. Total Environ., 2015, 532, 154–161 CrossRef CAS PubMed.
- M. V. Khodakovskaya, K. D. Silva, A. S. Biris, E. Dervishi and H. Villagarcia, Carbon nanotubes induce growth enhancement of tobacco cells, ACS Nano, 2012, 6(3), 2128–2135 CrossRef CAS PubMed.
- V. Asadi, S. H. Jafari, H. A. Khonakdar, L. Häuβler and U. Wagenknecht, Incorporation of inorganic fullerene-like WS2 into poly(ethylene succinate) to prepare novel biodegradable nanocomposites: A study on isothermal and dynamic crystallization, RSC Adv., 2016, 6(6), 4925–4935 RSC.
- B. P. Tripathi and K. S. Vinod, Organic–inorganic nanocomposite polymer electrolyte membranes for fuel cell applications, Prog. Polym. Sci., 2011, 36(7), 945–979 CrossRef CAS.
- P. Begum, R. Ikhtiari, B. Fugetsu, M. Matsuoka, T. Akasaka and F. Watari, Phytotoxicity of multi-walled carbon nanotubes assessed by selected plant species in the seedling stage, Appl. Surf. Sci., 2012, 262, 120–124 CrossRef CAS.
- D. Stampoulis, K. S. Saion and C. W. Jason, Assay-dependent phytotoxicity of nanoparticles to plants, Environ. Sci. Technol., 2009, 43(24), 9473–9479 CrossRef CAS PubMed.
- B. Sharma, S. Shekhar, S. Gautam and P. Jain, Dynamic shear rheology behavior and long term stability kinetics of reduced graphene oxide filledpoly(vinyl alcohol)biofilm, Polym. Test., 2018, 69, 583–592 CrossRef CAS.
- S. Shekhar, A. Sarkar, B. Sharma and P. Jain, Electrochemical evaluation of functionalized graphene oxide filled PVA-chitosan biohybrid for supercapacitor applications, J. Appl. Polym. Sci., 2020, 137(17), 48610 CrossRef CAS.
- P. Deshpande, A. Dapkekar, M. D. Oak, K. M. Paknikar and J. M. Rajwade, Zinc complexed chitosan/TPP nanoparticles: a promising micronutrient nanocarrier suited for foliar application, Carbohydr. Polym., 2017, 165, 394–401 CrossRef CAS PubMed.
- R. C. Choudhary, R. V. Kumaraswamy, S. Kumari, S. S. Sharma, A. Pal, R. Raliya, P. Biswas and V. Saharan, Cu-chitosan nanoparticle boost defense responses and plant growth in maize (Zea mays L.), Sci. Rep., 2017, 7(1), 1 CrossRef CAS PubMed.
- F. Brunel, N. E. E. Gueddari and B. M. Moerschbacher, Complexation of copper(II) with chitosan nanogels: toward control of microbial growth, Carbohydr. Polym., 2013, 92(2), 1348–1356 CrossRef CAS PubMed.
- F. O. M. S. Abreu, F. O. Erick, H. C. B. Paula and R. C. M. de Paula, Chitosan/cashew gum nanogels for essential oil encapsulation, Carbohydr. Polym., 2012, 89(4), 1277 CrossRef CAS PubMed.
-
R. Choudhary, R. V. Chandra, S. Kumaraswamy, A. Kumari, R. Pal, P. B. Raliya and V. Saharan, Synthesis, characterization, and application of chitosan nanomaterials loaded with zinc and copper for plant growth and protection. Nanotechnology, Springer, Singapore, 2017, pp. 227–247 Search PubMed.
- C. Hellmann, A. Greiner and J. H. Wendorff, Design of pheromone releasing nanofibers for plant protection, Polym. Adv. Technol., 2011, 22(4), 407–413 CrossRef CAS.
- M. Li, Q. Huang and Y. Wu, A novel chitosan-poly(lactide) copolymer and its submicron particles as imidacloprid carriers, Pest Manage. Sci., 2011, 67(7), 831–836 CrossRef CAS PubMed.
- A. Sharma, K. Sood, J. Kaur and M. Khatri, Agrochemical loaded biocompatible chitosan nanoparticles for insect pest management, Biocatal. Agric. Biotechnol., 2019, 18, 101079 CrossRef.
- J. L. De Oliveira, E. V. R. Campos, A. E. S. Pereira, L. E. S. Nunes, C. C. L. Da Silva, T. Pasquoto, R. Lima, G. Smaniotto, R. A. Polanczyk and L. F. Fraceto, Geraniol encapsulated in chitosan/gum arabic nanoparticles: A promising system for pest management in sustainable agriculture, J. Agric. Food Chem., 2018, 66(21), 5325–5334 CrossRef PubMed.
- C. Yeguerman, E. Jesser, M. Massiris, C. Delrieux, A. P. Murray and J. O. W. González, Insecticidal application of essential oils loaded polymeric nanoparticles to control German cockroach: Design, characterization and lethal/sublethal effects, Ecotoxicol. Environ. Saf., 2020, 189, 110047 CrossRef CAS PubMed.
- Y. Gao, Y. Xiao, K. Mao, X. Qin, Y. Zhang, D. Li, Y. Zhang, J. Li, H. Wan and S. He, Thermoresponsive polymer-encapsulated hollow mesoporous silica nanoparticles and their application in insecticide delivery, Chem. Eng. J., 2020, 383, 123169 CrossRef CAS.
- M. Pascoli, F. P. de Albuquerque, A. K. Calzavara, B. Tinoco-Nunes, W. H. C. Oliveira, K. C. Gonçalves and R. A. Polanczyk,
et al., The potential of nanobiopesticide based on zein nanoparticles and neem oil for enhanced control of agricultural pests, J. Pest. Sci., 2020, 93(2), 793–806 CrossRef.
- K. Fukamachi, Y. Konishi and T. Nomura, Disease control of Phytophthora infestans using cyazofamid encapsulated in poly lactic-co-glycolic acid (PLGA) nanoparticles, Colloids Surf., A, 2019, 577, 315–322 CrossRef CAS.
- Y. Liang, Y. Gao, W. Wang, H. Dong, R. Tang, J. Yang, J. Niu, Z. Zhou, N. Jiang and Y. Cao, Fabrication of smart stimuli-responsive mesoporous organosilica nano-vehicles for targeted pesticide delivery, J. Hazard. Mater., 2020, 122075 CrossRef CAS PubMed.
-
M. R. Assalin, L. D. L. dos Santos, D. R. C. Souza, M. A. Rosa, R. C. R. M. Duarte, R. F. Castanha, P. P. R. Donaire and N. Durán, Ecotoxicity evaluation: preparation of poly-ε-caprolactone and chitosan nanoparticles as carriers of thiamethoxam pesticide, Journal of Physics: Conference Series, IOP Publishing, 2019, vol. 1323, no. 1, p. 012017 Search PubMed.
- E. V. R. Campos, L. F. P. Patrícia, J. L. Oliveira, A. E. S. Pereira, L. Nunes de Morais Ribeiro, F. O. Fernandes and K. C. Gonçalves,
et al., Carvacrol and linalool co-loaded in β-cyclodextrin-grafted chitosan nanoparticles as sustainable biopesticide aiming pest control, Sci. Rep., 2018, 8(1), 1–14 Search PubMed.
- H. C. Erythropel, J. B. Zimmerman, T. M. de Winter, L. Petitjean, F. Melnikov, C. H. Lam and A. W. Lounsbury, The Green ChemisTREE: 20 years after taking root with the 12 principles, Green Chem., 2018, 20(9), 1929–1961 RSC.
- P. Ponmurugan, Biosynthesis of silver and gold nanoparticles using Trichoderma atroviride for the biological control of Phomopsis canker disease in tea plants, IET Nanobiotechnol., 2017, 11(3), 261–267 CrossRef PubMed.
- P. Singh, Y.-J. Kim, D. Zhang and D.-C. Yang, Biological synthesis of nanoparticles from plants and microorganisms, Trends Biotechnol., 2016, 34(7), 588–599 CrossRef CAS PubMed.
- C. Xiang, G. T. Alan, J. P. Hinestroza and M. W. Frey, Controlled release of nonionic compounds from poly(lactic acid)/cellulose nanocrystal nanocomposite fibers, J. Appl. Polym. Sci., 2013, 127(1), 79–86 CrossRef CAS.
- M. C. Delfour, Drug release kinetics from biodegradable polymers via partial differential equations
models, Acta Appl. Math., 2012, 118(1), 161–183 CrossRef.
- R. Grillo, N. Z. P. dos Santos, C. R. Maruyama, A. H. Rosa, R. D. Lima and L. F. Fraceto, Poly(ε-caprolactone) nanocapsules as carrier systems for herbicides: Physico-chemical characterization and genotoxicity evaluation, J. Hazard. Mater., 2012, 231–232, 1–9 CrossRef CAS PubMed.
- D. J. Sarkar, J. Kumar, N. A. Shakil and S. Walia, Release kinetics of controlled release formulations of thiamethoxam employing nano-ranged amphiphilic PEG and diacid based block polymers in soil., J. Environ. Sci. Health, Part A: Toxic/Hazard. Subst. Environ. Eng., 2012, 47(11), 1701–1712 CrossRef CAS PubMed.
- P. L. Ritger and A. P. Nikolaos, A simple equation for description of solute release II. Fickian and anomalous release from swellable devices, J. Controlled Release, 1987, 5(1), 37–42 CrossRef CAS.
- P. Kaushik, N. A. Shakil, J. Kumar, M. K. Singh, M. K. Singh and S. K. Yadav, Development of controlled release formulations of thiram employing amphiphilic polymers and their bioefficacy evaluation in seed quality enhancement studies, J. Environ. Sci. Health, Part B, 2013, 48(8), 677–685 CrossRef CAS PubMed.
- K. M. Loha, N. A. Shakil, J. Kumar, M. K. Singh, T. Adak and S. Jain, Release kinetics of β-cyfluthrin from its encapsulated formulations in water, J. Environ. Sci. Health, Part B, 2011, 46(3), 201–206 CrossRef CAS PubMed.
- P. Saini, M. Gopal, R. Kumar and C. Srivastava, Development of pyridalyl nanocapsule suspension for efficient management of tomato fruit and shoot borer (Helicoverpa armigera), J. Environ. Sci. Health, Part B, 2014, 49(5), 344–351 CrossRef CAS.
- S. N. A. Pankaj, N. A. Shakil, J. Kumar, M. K. Singh and K. Singh, Bioefficacy evaluation of controlled release formulations based on amphiphilic nano-polymer of carbofuran against Meloidogyne incognita infecting tomato, J. Environ. Sci. Health, Part B, 2012, 47(6), 520–528 CrossRef PubMed.
-
Y. Sasson, G. Levy-Ruso, O. Toledano and I. Ishaaya. Nanosuspensions: emerging novel agrochemical formulations, Insecticides design using advanced technologies, Springer, Berlin, Heidelberg, 2007, pp. 1–39 Search PubMed.
-
P. Saini, S. Kumar, V. K. Sharma and K. A. Chobhe, Nanopesticides: manage food security and environmental pollution, Biotech Articles, 2018, published online, https://www.biote
charticles.com/Agriculture-Article/Nanopesticides-Manage-
Food-Security-and-Environmental-Pollution-4391.html#:B:
text=Nanopesticides%20has%20been%20visualized%20as,
materials%20to%20deliver%20pesticides.
- iGate Research. “Global nanotechnology (component and applications) markets 2018–2024”, Report by Research and Markets, 2018, pp. 3-191.
- FAOSTAT, data on consumption of pesticides on a global scale and country-wise, Food and Agriculture Organisation of the United Nations, http://www.fao.org/faostat/en/#data/RP/visualize.
- Our World in Data, data on prevalence of neoplasms or share of population with cancer, on a global scale and country-wise, Global Change Data Lab, University of Oxford and Oxford Martin School, https://ourworldindata.org/grapher/share-of-population-with-cancer?tab=table.
- M. T. Islam, Applications of nanomaterials for future food security: challenges and prospects, Malaysian Journal of Halal Research, 2019, 6–9 CrossRef CAS.
- A. Di Guardo, T. Gouin, M. MacLeod and M. Scheringer, Environmental fate and exposure models: advances and challenges in 21 st century chemical risk assessment, Environ. Sci.: Processes Impacts, 2018, 20(1), 58–71 RSC.
- R. Grillo, F. F. Leonardo, M. J. B. Amorim, J. James Scott-Fordsmand and R. Schoonjans, and Qasim Chaudhry. Ecotoxicological and regulatory aspects of environmental sustainability of nanopesticides, J. Hazard. Mater., 2020, 124148 Search PubMed.
- M. Nehra, N. Dilbaghi, G. Marrazza, A. Kaushik, C. Sonne, K.-H. Kim and S. Kumar, Emerging nanobiotechnology in agriculture for the management of pesticide residues, J. Hazard. Mater., 2021, 401, 123369 CrossRef CAS PubMed.
- J. J. Villaverde, B. Sevilla-Morán, C. López-Goti, J. L. Alonso-Prados and P. Sandín-España, Considerations of nano-QSAR/QSPR models for nanopesticide risk assessment within the European legislative framework, Sci. Total Environ., 2018, 634, 1530–1539 CrossRef CAS PubMed.
- A. S. Abdelsattar, A. Dawoud and M. A. Helal, Interaction of nanoparticles with biological macromolecules: A review of molecular docking studies, Nanotoxicology, 2021, 15(1), 66–95 CrossRef CAS PubMed.
- S. Mishra, W. Wang, I. Pires de Oliveira, A. J. Atapattu, S.-W. Xia, R. Grillo, C. H. Lescano and X. Yang, Interaction mechanism of plant-based nanoarchitectured materials with digestive enzymes of termites as target for pest control: Evidence from molecular docking simulation and in vitro studies, J. Hazard. Mater., 2021, 403, 123840 CrossRef CAS PubMed.
- W. Mushtaq, A. Shakeel, M. A. Fazili, I. Chakrabartty and M. Sevindik, Pros and cons of nanotechnology, Nanobiotechnology, 2020, 207–222 Search PubMed.
- P. L. Chariou, A. B. Dogan, A. G. Welsh, G. M. Saidel, H. Baskaran and N. F. Steinmetz, Soil mobility of synthetic and virus-based model nanopesticides, Nat. Nanotechnol., 2019, 14(7), 712–718 CrossRef CAS PubMed.
- P. Zhang, Z. Guo, S. Ullah, G. Melagraki, A. Afantitis and I. Lynch, Nanotechnology and artificial intelligence to enable sustainable and precision agriculture, Nat. Plants, 2021, 1–13 Search PubMed.
- A. Saha, Bionanotechnology-Based Nanopesticide Application in Crop Protection Systems, Funct. Nanomater., 2021, 89–107 Search PubMed.
- M. Pascoli, T. J. Mauricio, A. D. Agarrayua, D. S. Avila, R. Lima and L. F. Fraceto, Neem oil based nanopesticide as an environmentally-friendly formulation for applications in sustainable agriculture: An ecotoxicological perspective, Sci. Total Environ., 2019, 677, 57–67 CrossRef CAS PubMed.
- M. Zhao, H. Zhou, L. Hao, H. Chen and X. Zhou, Natural rosin modified carboxymethyl cellulose delivery system with lowered toxicity for long-term pest control, Carbohydr. Polym., 2021, 259, 117749 CrossRef CAS PubMed.
-
O. Christiaens, M. Petek, G. Smagghe and C. N. T. Taning, The use of nanocarriers to improve the efficiency of RNAi-based pesticides in agriculture, Nanopesticides, Springer, Cham, 2020, pp. 49–68 Search PubMed.
- S. Yan, B.-Y. Ren and J. Shen, Nanoparticle-mediated double-stranded RNA delivery system: A promising approach for sustainable pest management, Insect
Sci., 2021, 28(1), 21–34 CrossRef CAS PubMed.
- S. Arpaia, O. Christiaens, K. Giddings, H. Jones, B. Mezzetti, F. Moronta-Barrios and J. N. Perry,
et al., Biosafety of GM crop plants expressing dsRNA: data requirements and EU regulatory considerations, Front. Plant Sci., 2020, 11, 940 CrossRef PubMed.
- M. Vurro, C. Miguel-Rojas and A. Pérez-de-Luque, Safe nanotechnologies for increasing the effectiveness of environmentally friendly natural agrochemicals, Pest Manage. Sci., 2019, 75(9), 2403–2412 CrossRef CAS PubMed.
- T. Hofmann, G. V. Lowry, S. Ghoshal, N. Tufenkji, D. Brambilla, J. R. Dutcher and L. M. Gilbertson,
et al., Technology readiness and overcoming barriers to sustainably implement nanotechnology-enabled plant agriculture, Nat. Food, 2020, 1(7), 416–425 CrossRef.
- H. Kolge, K. Kadam, S. Galande, V. Lanjekar and V. Ghormade, New Frontiers in Pest Control: Chitosan Nanoparticles-Shielded dsRNA as an Effective Topical RNAi Spray for Gram Podborer Biocontrol, ACS Appl. Bio Mater., 2021, 4(6), 5145–5157 CrossRef CAS.
-
P. C. Balaure, D. Gudovan and I. Gudovan, Nanopesticides: a new paradigm in crop protection, New pesticides and soil sensors, Academic Press, 2017, pp. 129–192 Search PubMed.
Footnote |
† The pictorial representation (Fig. 7) is based on data collected by the Food and Agriculture Organisation of the United Nations and the Institute of Health Metrics and Evaluation, USA. |
|
This journal is © The Royal Society of Chemistry 2021 |
Click here to see how this site uses Cookies. View our privacy policy here.