DOI:
10.1039/D0RA08573A
(Paper)
RSC Adv., 2021,
11, 2025-2039
The controlled synthesis of g-C3N4/Cd-doped ZnO nanocomposites as potential photocatalysts for the disinfection and degradation of organic pollutants under visible light irradiation
Received
8th October 2020
, Accepted 1st December 2020
First published on 7th January 2021
Abstract
The in situ growth of well-dispersed Cd-doped ZnO nanoparticles (Cd-ZnO NPs) on graphitic carbon nitride (g-C3N4) nanosheets was successfully achieved through the co-precipitation method for the formation of Cd-doped ZnO nanocomposites with g-C3N4 (Cd-ZnO/g-C3N4 NCs). The effect of different compositions of ternary nanocomposites (Cd-ZnO/g-C3N4 NCs) on photocatalytic properties was investigated. Ternary NCs, in which 60% g-C3N4 hybridized with 7% Cd-doped ZnO (g-C3N4/Cd-ZnO) NCs were proven to be optimum visible-light-driven (VLD) photocatalysts for the degradation of methylene blue (MB) dye. The enhanced photodegradation of MB is mainly due to the increase in the generation of photogenerated charge carriers (reactive oxygen species (ROS), O2−, and ˙OH radicals). The electron spin resonance (ESR) experiment revealed that the superoxide and hydroxyl radicals were the leading species responsible for the degradation of MB. Moreover, the NC exhibited tremendous stability with a consistently high MB degradation rate for 10 successive catalytic cycles. The structural and optical properties of CdO, ZnO NPs, Cd-ZnO NPs, g-C3N4 NSs, and g-C3N4/Cd-ZnO NCs were investigated via XRD, SEM, EDX, TEM, FTIR spectroscopy, UV-Vis spectroscopy, ESR spectroscopy, and PL spectroscopy techniques. The synthesized photocatalysts were also applied against Gram-positive and Gram-negative bacterial strains to evaluate their antibacterial activities.
1. Introduction
Currently, synthesized metal oxide nanoparticles have been employed as photocatalysts for the degradation of industrial dyes and detoxification of wastewater. Furthermore, the production of doped nanoparticles and formation of nanocomposites are modification techniques used to develop heterogeneous photocatalysts with enhanced dye degradation and antibacterial activity. Accordingly, as-fabricated doped semiconductor NPs have also demonstrated to be improved antimicrobial agents and photocatalytic materials.1 Similarly, the synthesis of metal-doped conjugated heterogeneous NCs has also been reported and proven to possess enhanced photocatalytic ability.2 Although there are various carbon-based polymeric materials available such as graphene, graphene oxide, and carbon nanotubes, most researchers used graphitic carbon nitride (g-C3N4) to fabricate NCs semiconductor metal oxides such as TiO2 and ZnO. The preference of g-C3N4 over other mesoporous materials is based on its high adsorption capacity, electronic properties, and highly porous nature.3 Furthermore, the doping of g-C3N4 nanomaterials with metals and semiconductors was reported to enhance its photocatalytic nature due to the increase in the availability of electrons in the g-C3N4 lattice.4
A research group used ZnO NPs hybridized with g-C3N4 to synthesized binary NCs for the degradation of MB dye under UV-light irradiation, which exhibited enhanced photocatalytic activity.5 Another study explained the synthesis of graphitic carbon nitride/ZnO NCs and reported that it exhibited enhanced photocatalytic activity compared with simple ZnO and g-C3N4 nanomaterials.6 The prepared NCs were used to degrade different organic dyes and environmental pollutants to study their photocatalytic nature.7 The 10 wt% g-C3N4-doped ZnO NC was also reported to exhibit high photocatalytic activity for the degradation of organic dyes such as Orange II, Reactive Black 5, and resazurin under UV-visible light irradiation. The large surface area, good photo-response, and production of abundant photogenerated charge carriers of the abovementioned binary NCs are possible reasons for their enhanced photocatalytic activity. The morphology and optical properties of abovementioned NCs were characterized via TEM, XRD, XPS, and UV-visible diffuse reflectance spectroscopy. The g-C3N4-doped ZnO NCs were also proven to exhibit a high degree of stability and reusability up to ten times, but were not considered good visible light harvesters.8
In photocatalysis, once the photocatalyst is exposed to light, the electrons present in its valance band are transferred to its conduction band by absorbing energy.9 Due to the transfer of photoelectrons (ecb−) to the conduction band, a positive identity is created in the valence band, which is known as a positive hole (hvb+).10 The photoelectrons (ecb−) and photogenerated holes (hvb+) can recombine on the surface in a few nanoseconds by dissipating the energy as heat. These electrons can also be trapped on the surface of donor (D) or acceptor (A) species such as water, which is adsorbed on or close to the surface of the photocatalytic material, containing contaminants in it.11 To utilize photoelectrons (ecb−) and photogenerated holes (hvb+) before their recombination, doping and composite formation are the reported solutions to date, which work by delaying the recombination of electron–hole pairs.12
The abovementioned binary composites were not suitable for electron–hole pair generation since they utilize a limited amount of the visible range of the light spectrum, which constitutes more than 40% energy of the total sunlight energy. In the modern world, antibacterial materials are frequently used because they can protect human beings from many diseases that are initiated due to bacterial exposure or contact. The reactive oxygen species (ROS) released by antibacterial agents can kill bacteria instantly when they come into contact. Undoped ZnO nanomaterials and transition metal-doped ZnO nanomaterials have been reported as antibacterial agents.13 The methods used to study the antibacterial properties of synthesized materials include the bioautographic method, agar diffusion method, and agar dilution method.14 The ZnO/g-C3N4 NCs were also reported to exhibit improved antibacterial activity compared to that of the pristine ZnO NPs but not to an optimum extent.15
This study is based on overcoming the challenge of the ever-increasing dye pollution in wastewater from the textile industry via the dye degradation method by applying unique ternary NCs. The purpose of the synthesis of the cadmium-doped ZnO hybridized with heterogeneous conjugated g-C3N4 NCs was to harvest the visible portion of the sunlight. This dye degradation and antibacterial action can be performed in a shorter time limit and faster mode of action using the visible portion of sunlight.
2. Materials and methods
2.1. Reagents
The materials used for the synthesis of the ternary nanocomposites included zinc acetate dihydrate (Zn(CH3COO)2·2H2O), silver nitrate (AgNO3), tin chloride pentahydrate (SnCl4·5H2O), cadmium acetate dihydrate (Cd(CH3COO)2·2H2O), ammonium heptamolybdate tetrahydrate ((NH4)6Mo7O24·4H2O), sodium hydroxide (NaOH), urea ((NH2)2CO), de-ionized (DI) water and sodium lauryl sulfate. All chemicals and reagents were purchased from Sigma-Aldrich and used as received without further purification. MB, a well-known industrial dye, was obtained from Shafi Reso-Chem Lahore, Pakistan.
2.2. Bacterial strains
The bacterial strains for the antibacterial study were obtained from the Pakistan Council of Scientific & Industrial Research (PCSIR) Laboratories Lahore, Pakistan. The bacterial strains with their ATCC numbers are given as Escherichia coli ATCC® 25922™, Bacillus subtilis ATCC® 6051™, Streptococcus salivarius ATCC® 13419™, and Staphylococcus aureus ATCC® 23235™.
2.3. Synthesis of ZnO NPs
ZnO NPs were synthesized using the simple chemical precipitation method. Briefly, 21.96 g (100 mM) of Zn(CH3COO)2·2H2O was dissolved in 200 mL of deionized water. For proper dissolution, the prepared solution was stirred for 30 min. After 30 min, 500 mg of sodium lauryl sulfate was added, and this solution was further stirred for 15 min. For precipitation, the pH of the prepared solution was increased to 11 by the dropwise addition of 4 M NaOH with continuous stirring. After attaining pH 11, the addition of NaOH was stopped. Then the solution was stirred for 30 min. Later, the solution was filtered using a filtration assembly. The precipitate was washed several times with deionized water. After washing, the precipitate was recovered and dried at 100 °C for 2 h in a vacuum oven. Subsequently, the dried sample was calcined in a muffle furnace at 580 °C for 2 h. The calcinated ZnO NPs were recovered from the furnace and employed for subsequent experiments after grinding to a fine powder. The proposed mechanism for the synthesis of the ZnO NPs is as follows: |
Zn(CH3COO)2·2H2O + 2NaOH → Zn(OH)2 + 2CH3COONa + 2H2O
| (1) |
|
Zn(OH)2 + 2H2O = Zn2+ + 2OH− + 2H2O = [Zn(OH)4]2– + 2H+
| (2) |
|
[Zn(OH)4]2– ↔ ZnO22− + 2H2O
| (3) |
|
ZnO22− + 2H+ ↔ ZnO + H2O
| (4) |
2.4. Synthesis of Cd-ZnO NPs
For the preparation of (1, 3, 5, 7, and 9) wt% Cd-doped ZnO NPs, (266 mg, 798 mg, 1330 mg, 1862 mg, and 2394 mg) of Cd(CH3COO)2·2H2O and 21.96 g of Zn(CH3COO)2·2H2O were added to conical flasks, respectively. Then 200 mL of deionized water was added to each conical flask. The samples were stirred for 30 min to prepare homogenous solutions. After 30 min of stirring, 500 mg of sodium lauryl sulfate was added to each conical flask, and was stirring continued for 15 min. For precipitation, the pH of the prepared solution was increased to 11 by the dropwise addition of 4 M NaOH with continuous stirring. After attaining pH 11, the addition of NaOH was stopped. The solutions were stirred for 30 more min. Later, the solutions were filtered using filtration assemblies. The precipitates were washed several times with the deionized water. After washing, the precipitates were recovered and dried at 100 °C for 2 h in a vacuum oven. The calcination of dried samples was performed in a muffle furnace at 580 °C for 2 h. The calcinated (1%, 3%, 5%, 7%, and 9%) Cd-doped ZnO NPs were employed for subsequent experiments after grinding to a fine powder. The proposed mechanism for the synthesis of the Cd-doped ZnO NPs is as follows: |
2[xZn(CH3COO)2 + (1 − x)Cd(CH3COO)2] + 8NaOH → 2ZnxCd1−xO + 8CH3COONa + 4H2O + O2
| (5) |
2.5. Synthesis of g-C3N4/Cd-ZnO ternary NCs
To prepare the (25%, 50%, 55%, 60%, and 65%) g-C3N4/Cd-ZnO NCs, a weighed amount of Cd-ZnO NPs, and g-C3N4 NSs was added to a 500 mL flask containing 200 mL deionized water to prepare a mixture. This mixture was ultra-centrifuged for 30 min to form a homogeneous solution. The filtration of the synthesized solution was performed to recover the precipitates using a Gooch-crucible filtration assembly. The recovered precipitates were washed several times with deionized water. After washing, the precipitates were dried at 100 °C for 2 h in a vacuum oven. The calcination of the dried samples was performed in a muffle furnace for 2 h at 580 °C. The (25%, 50%, 55%, 60%, and 65) g-C3N4/Cd-ZnO NCs were employed for subsequent experiments after grinding to a fine powder. The proposed mechanism for the synthesis of g-C3N4/Cd-ZnO NCs is as follows: |
yg-C3N4 + xZn(CH3COO)2 + (1 − x)Cd(CH3COO)2 + 8NaOH → (g-C3N4)yZnxCd1xO + 8CH3COONa + 4H2O + O2
| (6) |
A schematic flow chart of the synthetic process for the g-C3N4/Cd-ZnO NCs is shown in Fig. 1.
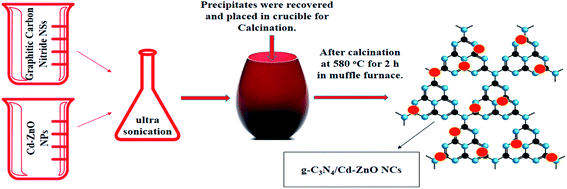 |
| Fig. 1 Schematic diagram showing the synthesis of g-C3N4/Cd-ZnO NCs. | |
2.6. Characterization
The composition and crystallinity of the samples were evaluated using an X-ray diffractometer (XRD, Bruker D8 Advance Eco), and the morphology of the synthesized samples was measured via transmission electron microscopy (TEM, JEOL-JEM-1230) and scanning electron microscopy (SEM, Jeol, 5910LV). The elemental proportions of the synthesized samples were identified via energy-dispersive spectroscopy (EDS). The Brunauer–Emmett–Teller (BET) surface area was determined by N2 adsorption in an automated gas sorption analyzer (Micromeritics ASAP 2020 instrument). Steady-state photoluminescence (PL) spectra were measured using a Fluoromax-4 spectrofluorometer (Horiba Scientific, Japan). The optical properties and photocatalytic dye degradation were examined and recorded using a UV-visible spectrophotometer (UV-Vis, JESCO). Fourier transform infrared (FT-IR) spectra were measured using a Bruker V70 spectrometer with a resolution of 1 cm−1. The optical bandgap of the synthesized samples was studied with a UV-vis-NIR diffuse reflectance spectrometer (UV-3600, Shimadzu). The electron spin resonance (ESR) signals of the spin-trapped paramagnetic species with 5,5-dimethyl-1-pyrroline N-oxide (DMPO) were recorded with a Magnet Tech MS400 spectrometer.
2.7. Photocatalytic activity
All the synthesized nanomaterials were assessed for their photocatalytic performance through the degradation of MB dye. In this study, anhydrous MB dye was used to prepare the test solutions (10 mg of the MB dye was dissolved in 100 mL of deionized water). Next, 12 mL of the above solution of MB dye was added to Petri dishes and 188 mL of deionized water was added. Then, 0.01 g of the synthesized nanomaterials was added. These dishes were kept in the dark for 30 min. Subsequently, 5 mL of sample solution was withdrawn from each Petri dish, and the absorption spectrum was recorded using a UV-visible spectrophotometer (JASCO 770) after centrifugation of the drawn sample to separate the residual catalyst. Subsequently, the above Petri dishes were exposed to sunlight, and 5 mL of sample solution from each Petri dish was withdrawn at 30 min intervals for the measurement of absorption spectra. The UV-visible spectra were obtained until the complete degradation of the MB dye occurred.
2.8. Antibacterial study
The first step was the preparation of the agar solution followed by its sterilization. After sterilization, the agar solution was poured into the previously sterilized Petri dishes in a laminar flow chamber. The dishes were left under the laminar flow until the media semi-solidified. Then, 1 mL of inoculum was added to each dish and shaken gently. Then the Petri dish was allowed to solidify completely. Afterward, wells were created in these Petri dishes using a steel borer. For each bacterium, the same procedure was repeated.
Negative and positive controls were also used, where sterilized de-ionized water was used as the negative control, and ampicillin trihydrate was used as the positive control. For sample placement, a microsyringe was used. For the antibacterial study, the Petri dishes were left for 24 h at 37 °C in an incubator. After 24 h, the plates were taken out from the incubator, and the inhibition zones were measured using a millimeter ruler. Each experiment was repeated thrice, and the inhibition zones were reported as mean ± standard deviation.16
3. Results and discussion
3.1. Scanning electron microscopy (SEM) and energy dispersive spectroscopy (EDS)
The SEM images of the cadmium metal-based NCs are shown in Fig. 2, together with their EDS spectra and elemental percentages. Specifically, the SEM images of the g-C3N4 NSs, ZnO NPs, g-C3N4/ZnO NCs, CdO NPs, Cd-ZnO NPs, and g-C3N4/Cd-ZnO NCs together with their EDS spectra and elemental percentages are shown. It is clear from these images that all the synthesized materials had particle sizes in the nanometer range. In Fig. 2(a), two-dimensional (2D) sheet-like sheets of g-C3N4 were observed to be stacked to each other. It can also be seen that the cross-sectional area of these NSs is in the range of 100 nm to 1000 nm, which serves as an excellent embedding site for hybridization with the NPs. In Fig. 2(a), the EDS spectrum of the g-C3N4 NSs shows intense peaks for C and N, and a small peak for O, confirming that these elements are present in the sample.
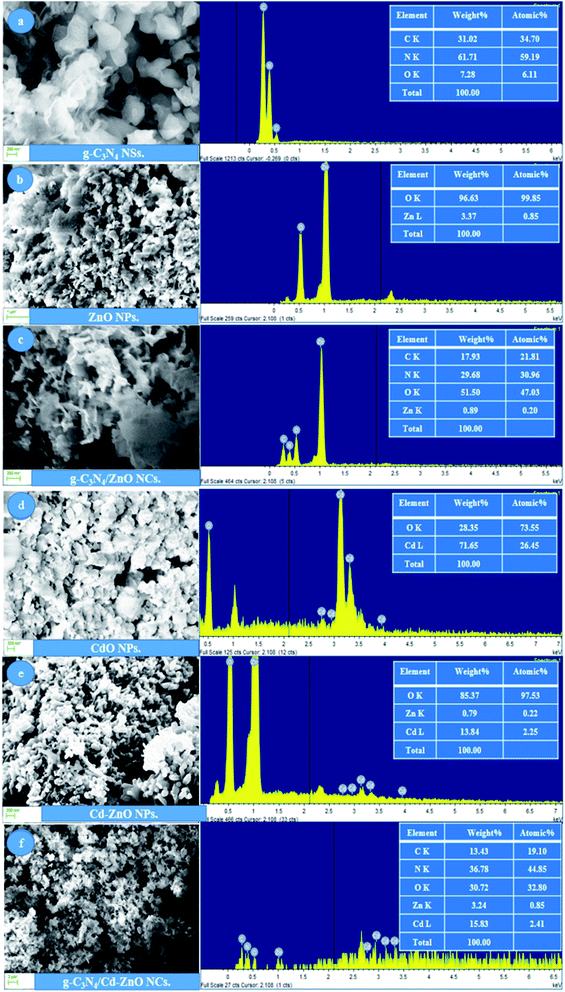 |
| Fig. 2 SEM images and EDS spectra of (a) g-C3N4 NSs, (b) ZnO NPs, (c) g-C3N4/ZnO NCs, (d) CdO NPs, (e) Cd-ZnO NPs, and (f) g-C3N4/Cd-ZnO NCs. | |
The empirical formula g-C3N4 was calculated from the weight percentages of the C and N elements. Oxygen with a low weight percentage may occur due to the oxidation of the terminal atoms of the g-C3N4 NSs, and this may also include adsorbed environmental oxygen in the scanned sample. Due to the absence of any residual peak in the EDS of g-C3N4, it can be considered that the synthesized NSs were highly pure. In Fig. 2(b), the ZnO NPs can be observed to have a particle size of less than 100 nm. The ZnO NPs exhibit the characteristic shapes ranging from rod-like, oblong, and flower-like shapes with agglomeration. The EDS spectrum of the ZnO NPs exhibited significant peaks of Zn and O, confirming the presence of these elements in the sample. The empirical formula ZnO was calculated based on the weight percentage of the Zn and O elements. Due to the absence of any residual peak in the EDS of ZnO, it can be considered that the synthesized NPs are highly pure. In Fig. 2(c), the SEM image of the g-C3N4/ZnO NC show the ZnO NPs uniformly embedded in the g-C3N4 NSs. The EDS spectrum of the g-C3N4/ZnO NC contains peaks of Zn, C, N, and O elements, which confirm the successful synthesis of the g-C3N4/ZnO NC. The absence of any other residual element peaks confirms that the synthesized NCs are highly pure. The SEM image of the CdO NPs is shown in Fig. 2(d), which exhibit a petal-like shape and particle size in the range of 100 nm to 200 nm with agglomeration in the form of petal chunks. The EDS spectrum of the CdO NPs contains intense peaks for the Cd and O elements, confirming the formation of the desired metal oxide NPs. The presence of residual peaks may account for the impurities in the sample with extremely minute quantity.
The empirical formula of CdO was calculated based on the weight percentage of the elements present in the sample. The EDS spectrum of the Cd-ZnO NPs confirmed their successful synthesis. Fig. 2(e) shows rod-like Cd-ZnO NPs with an average diameter of around 40 nm to 50 nm and length of around 200 nm to 500 nm with a stacked formation. The EDS spectrum of the Cd-ZnO NPs contains intense peaks for Cd, Zn, and O confirming the formation of desired cadmium-doped zinc oxide NPs. The absence of residual peaks confirmed that highly pure cadmium-doped zinc oxide NPs were successfully synthesized. The SEM image of the g-C3N4/Cd-ZnO NC is shown in Fig. 2(f). It shows that nano-rods of Cd-ZnO are embedded on the g-C3N4 NSs uniformly and the size of the composite is in the nanometer range with nano-rods erected in all possible vertical directions and angles. This confirmed the successful synthesis of the desired NCs using the proposed synthetic strategy. The synthesized g-C3N4/Cd-ZnO NC possesses a large surface area, which provides excessive space for the absorption of sunlight, resulting in high photocatalytic activity. The EDS spectrum of the g-C3N4/Cd-ZnO NC contains intense peaks for Cd, C, N, Zn, and O, confirming the formation of the desired NC. The absence of residual peaks confirms that a highly pure g-C3N4/Cd-ZnO NC was successfully synthesized.
3.2. Transmission electron microscopy (TEM)
The TEM images of the cadmium metal-based nanomaterials are described below. Fig. 3(a–f) show the TEM images of the g-C3N4 NSs, ZnO, CdO, Cd-ZnO NPs, g-C3N4/ZnO, and g-C3N4/Cd-ZnO NCs, respectively. It was clear from the images that all the synthesized materials possess a particle size in the nanometer range. In Fig. 3(a), two nanosheets of g-C3N4 can be observed to be stacked from ends angularly, having a width of around 200 nm and length of around 500 nm. Small spherical petals having a diameter of around 50 nm to 100 nm for g-C3N4 can also be observed to be stacked in a dispersed manner on top of larger sheets. In Fig. 3(b), the ZnO NPs can be seen with particle sizes ranging from 20 nm to less than 100 nm. The ZnO NPs exhibit the characteristic shapes ranging from round, spherical, rod-like, oblong, and flower-shaped in loosely agglomerated form. In Fig. 3(c), the g-C3N4/ZnO NC show ZnO NPs uniformly embedded on the g-C3N4 NSs.
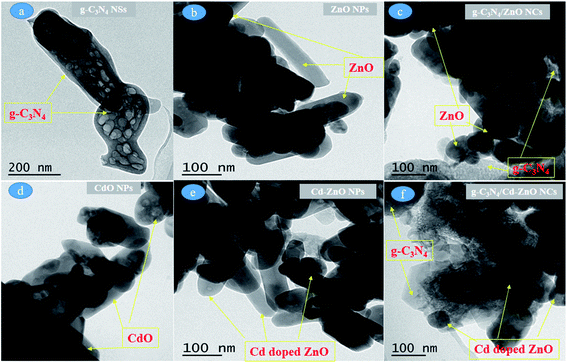 |
| Fig. 3 TEM images of (a) g-C3N4 NSs, (b) ZnO NPs, (c) g-C3N4/ZnO NCs, (d) CdO NPs, (e) Cd-ZnO NPs, and (f) g-C3N4/Cd-ZnO NCs. | |
The CdO NPs shown in Fig. 3(d) possess a round petal-like shape and particle size in the range of 50 nm to 100 nm. The CdO NPs exist as large chunks with excessive agglomeration. Fig. 3(e) shows rod-like Cd-ZnO NPs with an average diameter of around 30 nm to 40 nm and length of around 200 nm to 500 nm. The Cd-ZnO NP also exist as large chunks with excessive agglomeration. The g-C3N4/Cd-ZnO NCs shown in Fig. 3(f) exhibit 7% Cd-doped ZnO nano-rods embedded uniformly on the g-C3N4 NSs.
3.3. X-ray diffraction spectra
The X-ray diffraction spectra of all the cadmium metal-based synthesized nanomaterials are in the form of a combined graph in Fig. 4(a and b). The XRD pattern of the ZnO NPs shows diffraction peaks at 2θ = 32.1°, 34.5°, 36.4°, 47.7°, 56.7°, 62.5° and 68.1°, with the corresponding Miller indices of (1 0 0), (0 0 2), (1 0 1), (1 0 2), (1 1 0), (1 0 3) and (2 0 0), respectively. These peaks are consistent with the standard JCPDS pattern of ZnO NPs (36-1451), confirming the wurtzite structure of the ZnO NPs.17 The diffraction spectrum of the Cd-ZnO NPs shows eleven diffraction peaks, corresponding to (100), (002), (101), (102), (110), (103), (200), (112), (201), (004) and (202) and a wurtzite-type structure. The XRD pattern of the Cd-ZnO NPs was also compared and found to be in total agreement with the standard JCPDS pattern of Cd-ZnO NPs (36-1451). Considering the XRD pattern observed for Cd-ZnO NPs, it was proposed that the incorporation of Cd into the ZnO lattice successfully occurred either in the form of an interstitial atom or as a substitute for Zn2+ ions. The substitution of the Zn2+ ions with Cd atoms should result in a shift in the peaks in the diffraction pattern of Cd-ZnO NPs shown in Fig. 4(b). It was also notable that the ionic radii of the Zn2+ (0.72 Å) and Cd2+ (0.97 Å) ions were comparable. Thus, the possibility of substitution cannot be ruled out.18
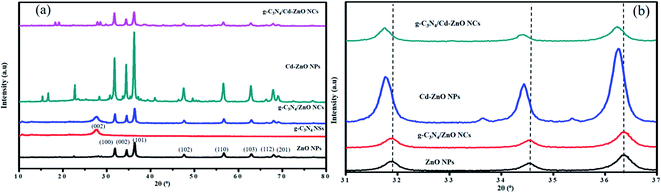 |
| Fig. 4 (a)XRD spectra of the g-C3N4 NSs, ZnO, Cd-ZnO NPs, g-C3N4/ZnO and g-C3N4/Cd-ZnO NCs. (b) XRD spectra of ZnO, Cd-ZnO NPs, g-C3N4/ZnO and g-C3N4/Cd-ZnO NCs. | |
The XRD diffraction pattern of the g-C3N4 NSs exhibits a diffraction peak at 2θ = 27.4°, corresponding to the Miller index of (0 0 2). The observed XRD pattern of the g-C3N4 NSs was compared to the standard JCPDS of nanosized g-C3N4 (87-1526), which were the same. The synthesized g-C3N4 NSs exist in the form of a stacked interlayer aromatic geometry.19 The XRD pattern of the g-C3N4/ZnO NCs is also shown in Fig. 4(a). The diffraction peaks were observed at 2θ = 27.4°, 32.1°, 34.5°, 36.4°, 47.7°, 56.7°, 62.5°, and 68.1°. A shift in the diffraction peaks did not occur, confirming that the ZnO NPs were incorporated uniformly on the sheets of g-C3N4 without any distortion.20 Similarly, no change in the wurtzite structure of ZnO occurred.21 The XRD pattern of the g-C3N4/Cd-ZnO NC is also shown in Fig. 4(a). The diffraction peaks were observed at 2θ = 27.4°, 32.1°, 32.8°, 34.5°, 36.4°, 38.2°, 47.7°, 55.3°, 56.7°, 62.5°, 65.9°, and 68.1°. A shift in the diffraction peaks occurred, confirming that the 7% Cd-doped ZnO NPs were incorporated uniformly in the sheets of g-C3N4 with a slight distortion.
3.4. FTIR spectroscopy
The FTIR spectra of the cadmium metal-based nanomaterials are in Fig. 5. The spectrum of g-C3N4 shows various absorption bands due to the presence of different stretching and bending vibration modes. An absorption band was observed at 1615 cm−1 due to the stretching vibration of the –C
C bonds. The sharp peak at 1313 cm−1 is associated with the C–C and C–N stretching vibrations. The absorption peak at 805 cm−1 matches the C–N bending vibrations.22 The absorption bands at 1403, 1455, and 1537 cm−1 are associated with the heptazine-derived repeating units.23 The broad absorption band at around 3100 cm−1 corresponds to the N–H stretching vibrations.24 The FTIR spectrum of the ZnO NP shows two absorption bands in the range of 1380 cm−1 to 1420 cm−1, which are ascribed to the bending vibrations of the O–H group. A sharp peak was also observed at 1110 cm−1 due to the stretching mode of the Zn–O bond.25 The FTIR spectrum of the g-C3N4-ZnO NC shows strong signals between 1650 cm−1 and 1150 cm−1, which are associated with the stretching modes of the C
N and C–N bonds in the aromatic heterocycles. The sharp peak at 806 cm−1 corresponds to the 1,3,5-triazine ring.26 The FTIR spectrum of the Cd-ZnO NPs is also shown in Fig. 5. Three absorption bands appear at 601 cm−1, 610 cm−1, and 620 cm−1 due to the presence of metal–oxygen bonds, which confirm the formation of Cd-ZnO nano-junctions.27 The small absorption band observed at 722 cm−1 is associated with the Zn–O bending vibrational mode, which is the same as that observed for the pure ZnO NPs. Similarly, for the stretching mode of the Zn–O bond, a sharp peak was also observed at 1033 cm−1. This confirmed that cadmium doping shifted the absorption peaks towards the lower frequency region compared to that of the pure ZnO NPs. The peak at 858 cm−1 is mostly associated with the samples that were measured in air. The strong band located at 1400 cm−1 corresponds to the bending vibration mode of the O–H group of water molecules present in the form of moisture. A single absorption peak was also observed at around 2357 cm−1, which may account for the Cd metal doping in ZnO. The peak located at 3400 cm−1 is related to the O–H group stretching mode. The FTIR spectrum of the 60% g-C3N4 hybridized with 7% Cd-doped ZnO NCs is shown in Fig. 5. A sharp absorption band at 804 cm−1 corresponding to the 1,3,5-triazine ring modes was observed.28 A small absorption peak was observed at 2150 cm−1, which may account for the additional stretching modes produced due to the interaction of the cadmium metal with g-C3N4 and ZnO. The broad absorption band at 3180 cm−1 corresponding to the stretching vibration of the N–H bond merged with and caused a shift in the band from 3400 cm−1 to 3330 cm−1 for the stretching mode of the O–H groups.29
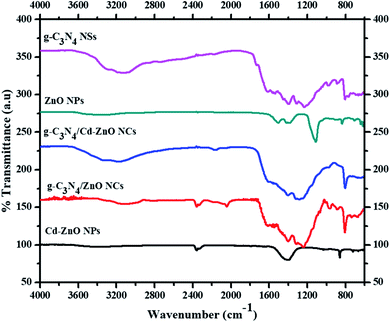 |
| Fig. 5 FTIR spectra of g-C3N4 NSs, ZnO NPs, Cd-ZnO NPs, g-C3N4/Cd-ZnO and g-C3N4/ZnO NCs. | |
3.5. Photoluminescence (PL) spectroscopy and BET surface area
The PL spectra of the ZnO, Cd, and Cd-ZnO NPs, g-C3N4 NSs, g-C3N4/ZnO, and g-C3N4/Cd-ZnO NCs were excited at an excitation wavelength of 320 nm and shown in Fig. 6(a). The PL spectra of the ZnO, Cd, and Cd-ZnO NPs, g-C3N4 NSs, g-C3N4/ZnO, and g-C3N4/Cd-ZnO NCs exhibit two emission bands at ∼390 and ∼445 nm. The emission band at ∼390 is ascribed to the near-band-edge emission rather than exciton–exciton collision. The emission band shape and position at ∼445 nm of the ZnO, Cd, and Cd-ZnO NPs, g-C3N4 NSs, g-C3N4/ZnO, and g-C3N4/Cd-ZnO NCs are the same except for a change in intensity. This may be due to the fact that all the nanomaterials were synthesized under the same conditions. The emission peak at ∼445 nm can be attributed to the combination of e− and h+ pairs. It was previously reported that the strong emission peak at ∼445 nm depends on the particle size of the nanomaterial.30
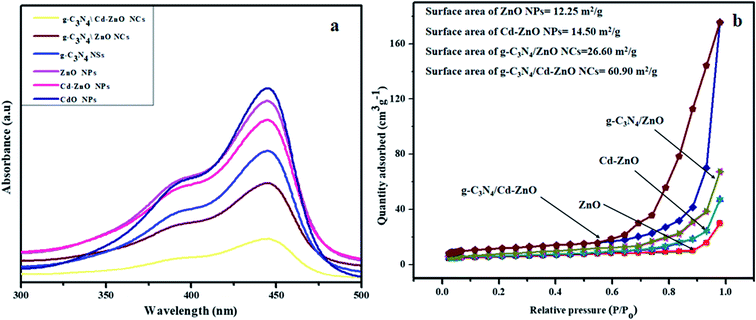 |
| Fig. 6 (a) PL spectra of the g-C3N4 NSs, CdO, ZnO and Cd-ZnO NPs, g-C3N4/Cd-ZnO, and g-C3N4/ZnO NCs and (b) BET curves of the ZnO and Cd-ZnO NPs, g-C3N4/Cd-ZnO, and g-C3N4/ZnO NCs. | |
The broad, intense peak at ∼445 nm is attributed to the surface-state emission or deep trap emission. It was found that the PL intensity of the doped and NC samples decreased in comparison to that of the pure ZnO NPs, CdO NPs, and g-C3N4 NSs. It may be due to the interfacial charge transfer from the NPs to g-C3N4 NSs. The prolonged lifetime and suppression of e–h recombination in g-C3N4/Cd-ZnO may result from the strong contact between ZnO and g-C3N4. Similar results are reported in the literature. Similarly, the emission peak intensity of g-C3N4/ZnO was low compared to the peak intensity of g-C3N4, suggesting that the heterostructure designed by coupling ZnO with g-C3N4 suppressed the frequency of e–h pair recombination.31 Concurrently, g-C3N4 functioning as an electron sink improves the migration of the photogenerated electrons and slows down the e–h recombination in both the g-C3N4/ZnO and g-C3N4/Cd-ZnO composites. The overall PL analysis exhibited that the lifetime of the photo-promoted electrons of the prepared samples followed the order of g-C3N4/Cd-ZnO > g-C3N4/ZnO > g-C3N4 > Cd-ZnO > ZnO > CdO. Hence, it is expected that the maximum MB photodegradation will be achieved by the g-C3N4/Cd-ZnO catalyst under solar radiation.
The pore volumes and BET surface areas of the ZnO and Cd-ZnO NPs, g-C3N4/ZnO, and g-C3N4/Cd-ZnO NCs were examined via N2 adsorption measurements. The N2 adsorption–desorption results are presented in Fig. 6(b) and listed in Table 1. The samples display type IV isotherms with an H3 hysteresis loop according to the IUPAC classification, indicating that the composite materials possess a mesoporous structure.32 It can be observed in Fig. 6(b) that the spherical-shape CdO NPs possess the smallest surface area (11.12 m2 g−1), while the g-C3N4/Cd-ZnO heterostructured photocatalyst has the largest surface area (60.90 m2 g−1). The greater surface area of the g-C3N4/Cd-ZnO heterostructure compared with other samples can promote the growth and number of surface active sites. Also, because of its distinctive structure (rod-like NPs of Cd-ZnO embedded in g-C3N4 NSs) and high specific surface area, the g-C3N4/Cd-ZnO NC is very effective for realizing an enhanced photocatalytic performance.
Table 1 The surface area, pore-volume, and average pore width of the synthesized NMs
Serial no. |
Photocatalyst |
Surface area, m2 g−1 |
Pore volume, cm3 g−1 |
Average pore width, Å |
1 |
ZnO NPs |
12.25 |
0.06 |
126.6 |
2 |
CdO NPs |
11.12 |
0.03 |
55.6 |
3 |
g-C3N4 NSs |
20.6 |
0.08 |
85.2 |
4 |
Cd doped ZnO NPs |
14.5 |
0.07 |
113.6 |
5 |
ZnO/g-C3N4 NC |
26.6 |
0.10 |
170.6 |
6 |
Cd-doped ZnO/g-C3N4 NC |
60.9 |
0.12 |
78.6 |
3.6. Bandgap analysis
All the synthesized samples were analyzed via UV-vis-NIR diffuse reflectance spectroscopy, and their Tauc plots were drawn using the standard procedure and mathematical calculations. The absorption spectra and Tauc plots of the g-C3N4 NSs, ZnO NPs, Cd-ZnO NPs, g-C3N4/ZnO, and g-C3N4/ZnO NCs are presented in Fig. 7(a and b).
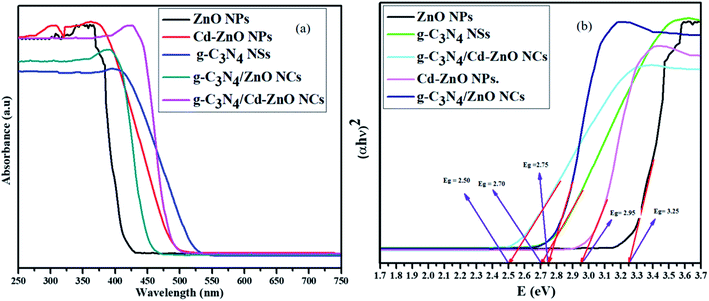 |
| Fig. 7 (a) Absorption spectra and (b) Tauc plots of the g-C3N4 NSs, ZnO NPs, and Cd-ZnO NPs, g-C3N4/ZnO, and g-C3N4/ZnO NCs. | |
The bandgap value of the g-C3N4 NSs was found to be 2.70 eV with λmax at around 410 nm, which is the same as that reported in the literature.6 The bandgap of the ZnO NPs was found to be 3.25 eV, and λmax at around 380 nm, which are also consistent with the values reported in the literature.33 The bandgap values of the g-C3N4 NSs and ZnO NPs indirectly confirmed their successful synthesis in the desired nanoform. Similarly, the bandgap value and λmax of the g-C3N4/ZnO NC were also calculated to be 2.75 eV and around 405 nm, respectively.34 Again, this means that the hybridization of ZnO with g-C3N4 caused a decrease in its bandgap. Also, it shifted its light-absorbing ability towards the visible region of the light spectrum.
Similarly, the bandgap values of the Cd-ZnO NPs and g-C3N4/Cd-ZnO NC were also calculated to be 2.95 (ref. 35) and 2.50 eV, respectively. The λmax of the Cd-ZnO NPs and g-C3N4/Cd-ZnO NC were also found to be around 380 and 430 nm, as shown in Fig. 7(a and b), respectively. The g-C3N4/ZnO photocatalyst showed improved visible light absorption (redshift) compared to pure g-C3N4. The same trend was observed for the g-C3N4/Cd-ZnO NC, where its optical absorption is notably shifted to the visible region with a bandgap of 2.50 eV. Moreover, among the samples, the g-C3N4/Cd-ZnO NC exhibited the strongest absorption over a long wavelength (<430 nm) in the visible region (Fig. 7(a)). This enhancement in visible light response by the g-C3N4/Cd-ZnO photocatalyst may be ascribed to the coupling of g-C3N4 NSs with Cd-ZnO NPs, and their matching energy band gaps, which can enable the NC to degrade pollutant efficiently.36
3.7. Photocatalytic activity
All the synthesized Cd-based nanomaterials were assessed for their photodegradation potential. The experiments were performed in a systematic way. In the first round of photocatalytic degradation experiments, the ZnO, CdO, and (1%, 3%, 5%, 7%, and 9%) Cd-ZnO NPs were assessed for their photocatalytic activity against MB, a well-known standard textile dye. The UV-visible absorption spectra and percentage degradation of MB dye of the above-described samples are shown in Fig. 8(a and b), respectively. It is clear from the spectra shown below that the Cd-ZnO NPs were photodegradation nanomaterials than the simple ZnO and CdO NPs. It was further proven that the 7 wt% Cd-doped ZnO (Cd-ZnO) NPs showed the optimal degradation rate among the doped NPs, which was 95% in just 120 min. The percentage of the MB dye degraded using pure ZnO NPs was 76% in 120 min. Thus, it can be concluded from the above results that although the degradation ability of Cd-ZnO NPs was greater than the simple ZnO NPs, it was not very significant. The only reason behind the small increase in degradation ability of ZnO after doping with Cd was that the surface area was not much enhanced due to the agglomeration of the Cd-ZnO NPs.37
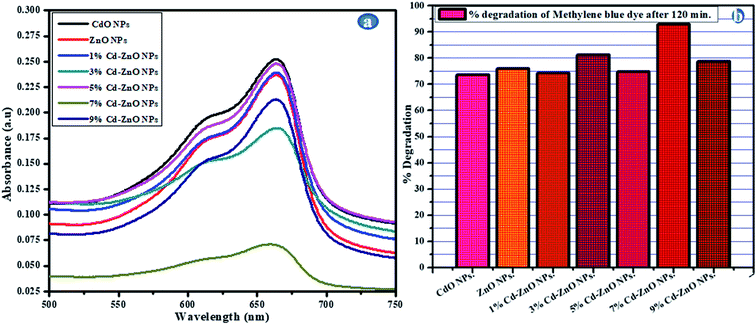 |
| Fig. 8 (a) Absorption spectra and (b) % degradation of MB for CdO, ZnO, (1%, 3%, 5%, 7%, and 9%) Cd-ZnO NPs. | |
The 7% Cd-doped ZnO NPs were studied to determine their photodegradation potential. The optical bandgap of the 7% Cd-doped ZnO NPs was calculated to be 2.95 eV.21 The 7 wt% doping with Cd lowered the bandgap of ZnO from 3.27 eV to 2.95 eV, and also the λmax after doping shifted towards the visible region, confirming that the 7% Cd-doped ZnO NPs has potential as a VLD photocatalyst. Consequently, a greater number of electron–hole pairs were generated, and hence enhanced optical activity was observed.
In the second round of photocatalytic degradation experiments, the 60% g-C3N4 hybridized with ZnO nanocomposites (g-C3N4/ZnO NCs), 7% Cd-doped ZnO (Cd-ZnO) NPs, and (25%, 50%, 55%, 60%, and 65%) g-C3N4 hybridized with 7% Cd-doped ZnO(g-C3N4/Cd-ZnO) NCs were employed for photodegradation of the MB dye. The UV-visible absorption spectra and percentage degradation of MB dye for the above-described samples are shown in Fig. 9(a and b), respectively. A tertiary combination of Cd, ZnO, and g-C3N4 was used to synthesize various percentages of g-C3N4 hybridized with 7% Cd-doped ZnO NCs. Among the tertiary composites, the 60% g-C3N4/ZnO NCs showed the optimum degradation activity. The maximum degradation potential of the g-C3N4/ZnO NCs may account for the optimal compatibility ratio of the materials. The low bandgap of 2.50 eV for the synthesized tertiary composite together with the existence of its λmax (430) in the visible region of the spectrum makes it an excellent VLD photocatalyst.
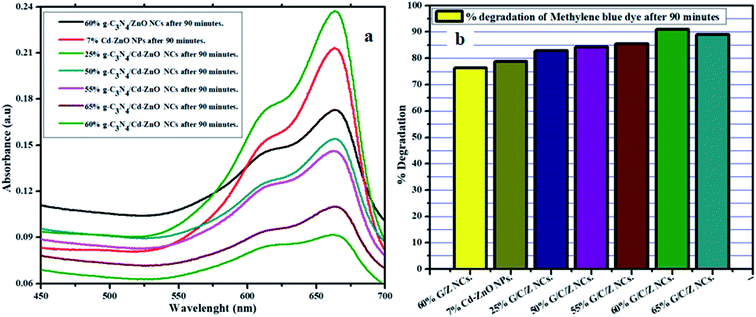 |
| Fig. 9 (a) Absorption spectra and (b) % degradation of the MB chart for the 60% g-C3N4/ZnO NCs, 7% Cd-ZnO NPs and (25%, 50%, 55%, 60%, and 65%) g-C3N4/Cd-doped ZnO NCs. | |
The generation of several charged species (electron–hole pairs) and their availably at the surface are the prominent factors for high photocatalytic degradation ability. The proposed mechanism for the generation of electron–hole pairs and photocatalytic degradation of the 60% g-C3N4 hybridized with 7% Cd-doped ZnO NCs is shown below.38
|
ZnOxCdyg-C3N4 + hυ → ZnOxCdyg-C3N4 + (e−)CB + (h+)VB
| (7) |
|
ZnOxCdyg-C3N4 (e−)CB + O2 → ZnOxCdyg-C3N4 + O2˙−
| (8) |
|
H2O + O2˙− → HO2− + OH˙
| (9) |
|
H2O + ZnOxCdyg-C3N4 (h+)VB → H+ + OH˙
| (10) |
|
OH˙ + MB → CO2 + H2O + other degradation products
| (12) |
|
O2˙− + MB → CO2 + H2O + other degradation products
| (13) |
To compare the degradation rates of all the synthesized nanomaterials, a graph was plotted with “C/Co” on the y-axis and “t” on the x-axis, as shown in Fig. 10. A pseudo-first-order kinetic relationship shown below in equation form was applied to determine the rate constant (k) for dye degradation for the 60% g-C3N4/Cd-ZnO NCs.39
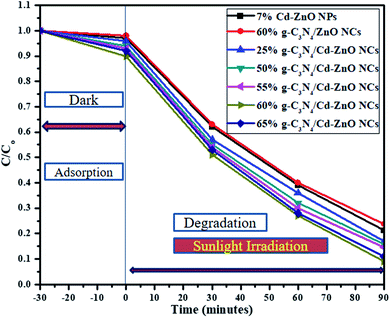 |
| Fig. 10 Comparing the rate of change in concentration with time for the 7% Cd-ZnO NPs, (25%, 50%, 55%, 60%, and 65%) g-C3N4/Cd-ZnO NCs and 60% g-C3N4/ZnO NCs. | |
The degradation constant (k) for the 60% g-C3N4 hybridized with 7% Cd-doped ZnO NCs was calculated to be 0.0255 min−1, which is highest among the synthesized NCs.40 Similarly, another graph was plotted with “ln
Co/C” on the y-axis and time “t” on the x-axis, as shown in Fig. 11(a). To further show the linear relationship, another graph was plotted with “ln
C/Co” on the y-axis and time “t” on the x-axis, as shown in Fig. 11(b). From all these graphs, it becomes clear that the 60% g-C3N4/Cd-ZnO NCs is the best VLD photocatalyst.
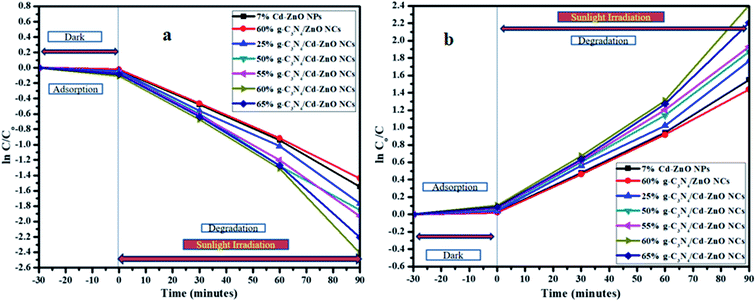 |
| Fig. 11 (a) Natural logarithm and (b) inverse of natural logarithm with the rate of the change in concentration with time for the 7% Cd-ZnO NPs, (25%, 50%, 55%, 60%, and 65)% g-C3N4/Cd-ZnO and 60% g-C3N4/ZnO NCs. | |
The 60% g-C3N4/Cd-ZnO NCs were used for the degradation of MB dye for 10 successive cycles, and it was established that the efficacy of the photocatalyst remained the same as that with its first use, as shown in Fig. 12(b). The XRD patterns of the tertiary NCs were measured three times, namely before their use, after 5-times use, and after 10-times use, as shown above in Fig. 12(a). Consequently, the XRD spectra were found to be identical. Thus, this indicates that the 60% g-C3N4/Cd-ZnO NCs retain their structural morphology and activity even after 10 uses.
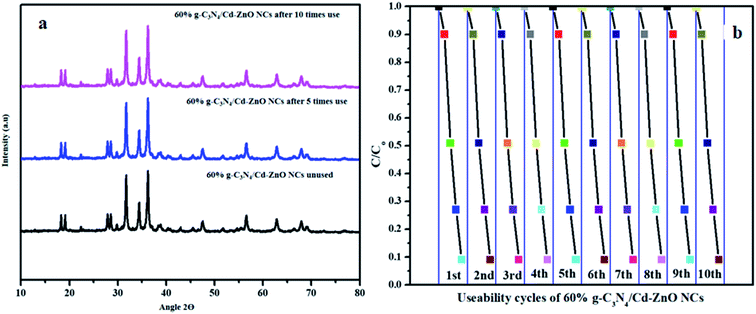 |
| Fig. 12 (a) XRD patterns of the 60% g-C3N4/Cd-ZnO NCs before use, after 5-times use after 10-time uses, and (b) 10 usability cycles for the 60% g-C3N4/Cd-ZnO NCs. | |
3.8. Photocatalytic degradation mechanism
To study the mechanism of photocatalytic dye degradation by applying the 60% g-C3N4/Cd-ZnO NCs, the influence of the active species (˙OH, h+, and ˙O2−) produced during the photodegradation process was scrutinized, as shown in Fig. 13(a). Ammonium oxalate (AO), benzoquinone (BQ), and isopropanol (IPA) scavengers were used as capture agents to remove photogenerated h+, ˙O2−, and ˙OH radicals, respectively.41,42 When benzoquinone (BQ) was added to the dye solution, the photodegradation of MB by the 60% g-C3N4/Cd-ZnO catalysts was limited to 11.62%. Similarly, with the addition of ammonium oxalate (AO) radical scavenger, the photodegradation declined from 92% to 79.59%. Thus, it is evident that the superoxide radicals are the dominant species and holes are minor oxidative species in the dye photodegradation process. Also, dye degradation was reduced to 37.83% upon the addition of isopropanol (IPA), which indicates that hydroxyl radicals act as secondary active species. To further validate the presence of hydroxyl and superoxide radicals, an electron spin resonance (EPR) experiment was conducted, and the results are depicted in Fig. 13(b). The DMOP-˙OH− and DMPO-˙O2− signals were distinctly observed when the 60% g-C3N4/Cd-ZnO photocatalyst suspension was exposed to visible light radiation, but no signal was detected in the dark. This study further confirms that the superoxide radicals are the dominant species and the hydroxyl radicals are present as the secondary active species. Thus, the EPR results are consistent with the observations of the active species capturing experiments.
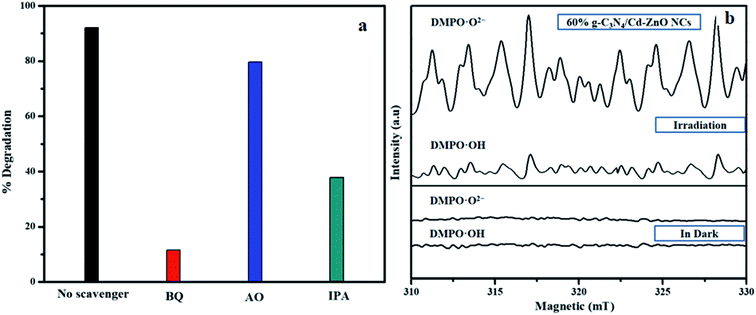 |
| Fig. 13 (a) Trapping experiment of active species during the photodegradation of MB over the 60% g-C3N4/Cd-ZnO composite and (b) ESR spectra of DMPO adducts. | |
3.9. Antibacterial study
The antibacterial activity of the Cd samples was studies against Gram-positive and against Gram-negative bacteria. The antibacterial activity was investigated using the standard ager diffusion protocol. The antibacterial study was performed against four bacterial strains (Bacillus subtilis, Streptococcus salivarius, Escherichia coli, and Staphylococcus aureus). After incubation, the Petri dishes were taken out and placed in a laminar flow hood. The zone of inhibition of all the samples, and the negative and positive controls was measured, and the results are presented in Table 2. The same procedure was repeated to measure and record the zone of inhibition for all the nanomaterials against all four bacterial strains.
Table 2 The zone of inhibition of the Cd-based nanomaterials against different bacterial strainsa
Antimicrobial agent |
Escherichia coli |
Bacillus subtilis |
Staphylococcus aureus |
Streptococcus salivarius |
Inhibition zone (mm) includes the diffusion assay disc diameter (4 mm), using 100 μL NM suspension. The inhibition zone diameter indicates triplicate ± standard deviation p < 0.05 compared with the negative control, i.e., blank/solvent (p < 0.05 is considered significant). |
100 μL |
mm |
mm |
mm |
mm |
Negative control |
00 |
00 |
00 |
00 |
Positive control |
23 |
17 |
26 |
28 |
ZnO NPs |
11 |
12 |
13 |
15 |
g-C3N4 NSs |
06 |
08 |
07 |
11 |
CdO NPs |
10 |
12 |
01 |
13 |
Cd doped ZnO NPs |
12 |
13 |
14 |
15 |
ZnO/g-C3N4 NC |
14 |
17 |
18 |
22 |
Cd doped ZnO/g-C3N4 NC |
18 |
20 |
21 |
24 |
It was found that all the nanomaterials (NPs, doped NPs, binary NC, and tertiary NC) showed a zone of inhibition against all four bacterial strains. The CdO NPs showed the minimum, while the 60% g-C3N4/Cd-doped ZnO NC (tertiary NC) showed the maximum bacterial inhibition zone. The antibacterial activity of the 60% g-C3N4/Cd-doped ZnO NC was compared with ampicillin as the positive control and found to have better values. The increase in the generation of reactive oxygen species (ROS) due to the reduction of the bandgap of ZnO and the large surface area provided by the g-C3N4 NSs for the surface interaction of the NC with the bacterial membrane were the possible reasons for the optimum antibacterial activity of the 60% g-C3N4/Cd-ZnO NC.17–19 The zone of inhibition was measured for all the synthesized samples against the four bacterial strains, as shown in Fig. 14. It is evident from the bar graph below that the ternary composite exhibit superior antibacterial activity among the synthesized nanomaterials.
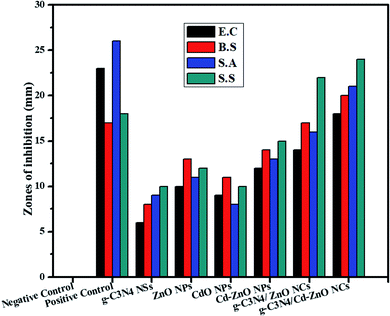 |
| Fig. 14 Zone of inhibition of the ZnO, CdO, and Cd-ZnO NPs, g-C3N4 NSs, 60% g-C3N4/ZnO, and 60% g-C3N4/Cd-ZnO NC against the studies bacterial strains. | |
4. Conclusion
A unique nanocomposite consisting of 60% g-C3N4 hybridized with 7% Cd-ZnO (60% g-C3N4/Cd-ZnO) was synthesized using a simple co-precipitation method. The systematic study of the synthesized NC revealed its superior photocatalytic and antibacterial nature. Its enhanced photocatalytic and antibacterial activity were attributed to the formation of a well-defined heterojunction between the g-C3N4 nanosheets, and rod-like Cd-doped ZnO NPs, which were verified by the characterization results. The photocatalytic performance of the 60% g-C3N4/Cd-ZnO NC was found to be superior to that of the CdO NPs, ZnO NPs, g-C3N4 NSs, Cd-ZnO NPs, and 60% g-C3N4/ZnO NC. Further, the enhanced photocatalytic nature of the 60% g-C3N4/Cd-ZnO NC was ascribed to its suppression of electron–hole pair recombination, advanced conjugation of electrons, and greater surface area, as verified through PL, EPR, and BET analysis. The antibacterial analysis revealed that the 60% g-C3N4/Cd-ZnO NC was the most effective and had high antibacterial susceptibility against Gram-positive and Gram-negative bacterial strains. Thus, on account of the remarkable photoactivity and bactericidal performance of the 60% g-C3N4/Cd-ZnO NC, it may be applied for the bacterial disinfection of wastewater and the photodegradation of numerous organic pollutants.
Conflicts of interest
The authors declare that they have no known competing financial interests or personal relationships that could have appeared to influence the work reported in this paper.
Acknowledgements
The authors would like to show appreciation to the University of Management & Technology, Lahore, Pakistan and the Korean Research Fellowship Program through the National Research Foundation of Korea (NRF), Korea, funded by the Ministry of Science and ICT (Grant No. NRF-2019H1D3A1A01102931) for providing the financial support of this work.
References
- H. Pan, Y. W. Zhang, V. B. Shenoy and H. Gao, Effects of H-, N-, and (H, N)-doping on the photocatalytic activity of TiO2, J. Phys. Chem. C, 2011, 115, 12224–12231 CrossRef CAS
. - X. Wang, X. Chen, A. Thomas, X. Fu and M. Antonietti, Metal-containing carbon nitride compounds: a new functional organic–metal hybrid material, Adv. Mater., 2009, 21, 1609–1612 CrossRef CAS
. - S. A. Khan, Z. Arshad, S. Shahid, I. Arshad, K. Rizwan, M. Sher and U. Fatima, Synthesis of TiO2/Graphene oxide nanocomposites for their enhanced photocatalytic activity against methylene blue dye and ciprofloxacin, Composites, Part B, 2019, 175, 107120 CrossRef CAS
. - H. Gao, S. Yan, J. Wang and Z. Zou, Ion coordination significantly enhances the photocatalytic activity of graphitic-phase carbon nitride, Dalton Trans., 2014, 43, 8178–8183 RSC
. - X. Wang, K. Maeda, A. Thomas, K. Takanabe, G. Xin, J. M. Carlsson and M. Antonietti, A metal-free polymeric photocatalyst for hydrogen production from water under visible light, Nat. Mater., 2009, 8, 76–80 CrossRef CAS
. - W. Liu, M. Wang, C. Xu and S. Chen, Facile synthesis of g-C3N4/ZnO composite with enhanced visible light photooxidation and photoreduction properties, Chem. Eng. J., 2012, 209, 386–393 CrossRef CAS
. - H. Cao, Y. G. Zhao, H. C. Ong, S. T. Ho, J. Y. Dai, J. Y. Wu and R. P. Chang, Ultraviolet lasing in resonators formed by scattering in semiconductor polycrystalline films, Appl. Phys. Lett., 1998, 73, 3656–3658 CrossRef CAS
. - H. Moussa, B. Chouchene, T. Gries, L. Balan, K. Mozet, G. Medjahdi and R. Schneider, Growth of ZnO nanorods on graphitic carbon nitride (g-C3N4) sheets for the preparation of photocatalysts with high visible-light activity, ChemCatChem, 2018, 10, 4973–4983 CrossRef
. - A. Fujishima, T. N. Rao and D. A. Tryk, Titanium dioxide photocatalysis, J. Photochem. Photobiol., B, 2000, 1, 1–21 CrossRef CAS
. - M. A. Oturan and J. J. Aaron, Advanced oxidation processes in water/wastewater treatment: principles and applications, A review, Crit. Rev. Environ. Sci. Technol., 2014, 44, 2577–2641 CrossRef CAS
. - K. Selvam, M. Muruganandham, I. Muthuvel and M. Swaminathan, The influence of inorganic oxidants and metal ions on semiconductor sensitized photodegradation of 4-fluorophenol, Chem. Eng. J., 2007, 128, 51–57 CrossRef CAS
. - J. Kim, K. C. Song, S. Foncillas and S. E. Pratsinis, Dopants for synthesis of stable bimodally porous Titania, J. Eur. Ceram. Soc., 2001, 21, 763–2872 Search PubMed
. - A. Sirelkhatim, S. Mahmud, A. Seeni, N. H. M. Kaus, L. C. Ann, S. K. M. Bakhori and D. Mohamad, Review on zinc oxide NP's: antibacterial activity and toxicity mechanism, Nano-Micro Lett., 2015, 7, 219–242 CrossRef CAS
. - M. I. Atta-ur-Rhman Choudhary and W. J. Thomsen, Bioassay Technique for Drug Development, Harwood Academic Publishers, 2001 Search PubMed
. - C. Liu, L. Wang, H. Xu, S. Wang, S. Gao, X. Ji and W. Lan, “One pot” green synthesis and the antibacterial activity of g-C3N4/Ag NCs, Mater. Lett., 2016, 164, 567–570 CrossRef CAS
. - M. A. Qamar, S. Shahid, M. Javed, S. Iqbal, M. Sher and M. B. Akbar, Highly efficient g-C3N4/Cr-ZnO nanocomposites with superior photocatalytic and antibacterial activity, J. Photochem. Photobiol., A, 2020, 112776 CrossRef CAS
. - M. J. Height, S. E. Pratsinis, O. Mekasuwandumrong and P. Praserthdam, Ag-ZnO catalysts for UV-photodegradation of
methylene blue, Appl. Catal., B, 2006, 63, 305–312 CrossRef CAS
. - H. Nagabhushana, R. B. Basavaraj, B. D. Prasad, S. C. Sharma, H. B. Premkumar and G. R. Vijayakumar, Facile EGCG assisted green synthesis of raspberry shaped CdO NPs, J. Alloys Compd., 2016, 669, 232–239 CrossRef CAS
. - K. Wang, Q. Li, B. Liu, B. Cheng, W. Ho and J. Yu, Sulfur-doped g-C3N4 with enhanced photocatalyticCO2-reduction performance, Appl. Catal., B, 2015, 176, 44–52 Search PubMed
. - Z. Xing, Y. Chen, C. Liu, J. Yang, J. Xu, Y. Situ and H. Huang, Synthesis of core-shell ZnO/oxygen doped g-C3N4 visible light driven photocatalyst via hydrothermal method, J. Alloys Compd., 2017, 708, 853–861 CrossRef CAS
. - J. Wang, Z. Yang, X. Gao, W. Yao, W. Wei, X. Chen and Y. Zhu, Core-shell g-C3N4@ZnO composites as photoanodes with double synergistic effects for enhanced visible-light photoelectrocatalytic activities, Appl. Catal., B, 2017, 217, 169–180 CrossRef CAS
. - W. K. Jo and N. C. S. Selvam, Enhanced visible light-driven photocatalytic performance of ZnO-g-C3N4 coupled with graphene oxide as a novel ternary nanocomposite, J. Hazard. Mater., 2015, 299, 462–470 CrossRef CAS
. - S. Kumar, T. Surendar, A. Baruah and V. Shanker, Synthesis of a novel and stable gC3N4–Ag3PO4 hybrid nanocomposite photocatalyst and study of the photocatalytic activity under visible light irradiation, J. Mater. Chem. A., 2013, 1, 5333–5340 RSC
. - S. Kumaresan, K. Vallalperuman and S. Sathishkumar, A Novel one-step synthesis of Ag-doped ZnO NP's for high performance photocatalytic applications, J. Mater. Sci.: Mater. Electron., 2017, 28, 5872–5879 CrossRef CAS
. - S. C. Yan, Z. S. Li and Z. G. Zou, Photodegradation performance of g-C3N4 fabricated by directly heating melamine, Langmuir, 2009, 25, 10397–10401 CrossRef CAS
. - A. Al-Hajry, A. Umar, Y. B. Hahn and D. H. Kim, Growth, properties and dye-sensitized solar cells-applications of ZnO nanorods grown by low-temperature solution process, Superlattices Microstruct., 2009, 45, 529–534 CrossRef CAS
. - R. A. Nyquist and R. O. Kagel, Handbook of infrared and Raman spectra of inorganic compounds and organic salts: infrared spectra of inorganic compounds, 2012, Academic Press, vol. 4 Search PubMed
. - X. Xu, S. Wang, T. Hu, X. Yu, J. Wang and C. Jia, Fabrication of Mn/O co-doped g-C3N4: excellent charge separation and transfer for enhancing photocatalytic activity under visible light irradiation, Dyes Pigm., 2020, 175, 108107 CrossRef CAS
. - N. Boonprakob, N. Wetchakun, S. Phanichphant, D. Waxler, P. Sherrell, A. Nattestad and B. Inceesungvorn, Enhanced visible-light photocatalytic activity of g-C3N4/TiO2 films, J. Colloid Interface Sci., 2014, 17, 402–409 CrossRef
. - X. Yuan, C. Zhou, Q. Jing, Q. Tang, Y. Mu and A. K. Du, Facile synthesis of g-C3N4 nano-sheets/ZnO NC's with enhanced photocatalytic activity in reduction of aqueous chromium (VI) under visible light, Nanomaterials, 2016, 6, 173 CrossRef
. - N. Wang, B. Han, J. Wen, M. Liu and X. Li, Synthesis of novel Mn-doped Fe2O3 nanocube supported g-C3N4 photocatalyst for overall visible-light driven water splitting, Colloids Surf., A, 2019, 567, 313–318 CrossRef CAS
. - G. V. Khade, M. B. Suwarnkar, N. L. Gavade and K. M. Garadkar, Sol–gel microwave assisted synthesis of Sm-doped TiO2 NPs and their photocatalytic activity for the degradation of Methyl Orange under sunlight, J. Mater. Sci.: Mater. Electron., 2016, 27, 6425–6432 CrossRef CAS
. - A. S. Manikandan, K. B. Renukadevi, K. Ravichandran, P. V. Rajkumar and K. Boubaker, Enhanced photo-catalytic, antibacterial and magnetic properties
of ZnO nanopowders through lattice compatible cobalt doping, J. Mater. Sci.: Mater. Electron., 2016, 27, 11890–11901 CrossRef CAS
. - G. P. Awasthi, S. P. Adhikari, S. Ko, H. J. Kim, C. H. Park and C. S. Kim, Facile synthesis of ZnO flowers modified graphene like MoS2 sheets for enhanced visible-light-driven photocatalytic activity and antibacterial properties, J. Alloys Compd., 2016, 682, 208–215 CrossRef CAS
. - R. Karthik and S. Thambidurai, Synthesis of cobalt doped ZnO/reduced graphene oxide nanorods as active material for heavy metal ions sensor and antibacterial activity, J. Alloys Compd., 2017, 715, 254–265 CrossRef CAS
. - D. Neena, M. Humayun, W. Zuo, C. S. Liu, W. Gao and D. J. Fu, Hierarchical hetero-architectures of in situ g-C3N4-coupled Fe-doped ZnO micro-flowers with enhanced visible-light photocatalytic activities, Appl. Surf. Sci., 2020, 506, 145017 CrossRef CAS
. - L. Ge, C. Han and J. Liu, In situ synthesis and enhanced visible light photocatalytic activities of novel PANI–gC3N4 composite photocatalysts, J. Mater. Chem., 2012, 22, 11843–11850 RSC
. - D. Zhang and F. Zeng, Visible light-activated cadmium-doped ZnO nanostructured photocatalyst for the treatment of methylene blue dye, J. Mater. Sci., 2012, 47, 2155–2161 CrossRef CAS
. - K. Karthik, S. Dhanuskodi, C. Gobinath and S. Sivaramakrishnan, Microwave-assisted synthesis of CdO–ZnO nanocomposite and its antibacterial activity against human pathogens, Spectrochim. Acta, Part A, 2015, 139, 7–12 CrossRef CAS
. - C. Karunakaran, A. Vijayabalan and G. Manikandan, Photocatalytic and bactericidal activities of hydrothermally synthesized nanocrystalline Cd-doped ZnO, Superlattices Microstruct., 2012, 51, 443–453 CrossRef CAS
. - S. Zhang, C. Su, H. Ren, M. Li, L. Zhu, S. Ge and X. Cao, In-situ fabrication of g-C3N4/ZnO nanocomposites for photocatalytic degradation of methylene blue: synthesis procedure does matter, Nanomaterials, 2019, 9, 215 CrossRef CAS
. - X. Zheng, J. Yuan, J. Shen, J. Liang, J. Che, B. Tang and H. Chen, A carnation-like rGO/Bi2O2CO3/BiOCl composite: efficient photocatalyst for the degradation of ciprofloxacin, J. Mater. Sci.: Mater. Electron., 2019, 30, 5986–5994 CrossRef CAS
.
|
This journal is © The Royal Society of Chemistry 2021 |
Click here to see how this site uses Cookies. View our privacy policy here.