DOI:
10.1039/D1RA03804D
(Paper)
RSC Adv., 2021,
11, 22433-22438
Self-assembly of diclofenac prodrug into nanomicelles for enhancing the anti-inflammatory activity†
Received
15th May 2021
, Accepted 19th June 2021
First published on 24th June 2021
Abstract
Non-steroidal anti-inflammatory drugs (NSAIDs) are widely prescribed for the treatment of various types of inflammatory conditions. Diclofenac is a very common NSAID that is utilized to relieve pain and reduce fever and, most importantly, inflammation. However, it suffers from low water solubility and a low dissolution profile. Therefore, we aim to develop a new drug delivery system based on the synthesis of amphiphilic structures that are capable of self assembling into nano-micelles which will be a water-soluble delivery system for the diclofenac. The amphiphilic structure consists of a hydrophilic moiety of triethylene glycol (TEG), polyethylene glycol PEG 400, or PEG 600 linked with the hydrophobic drug diclofenac through an ester linkage. The diclofenac derivatives were successfully synthesized as confirmed by nuclear magnetic resonance. Moreover, the formation of the micellar structure of the synthesized amphiphilic derivatives was confirmed by atomic force microscopy obtaining a spherical shape of the micelles with average diameters of 200 nm for Dic-PEG400-Dic, and 110 nm for Dic-PEG600-Dic. The critical micelle concentration has been determined as 2.7 × 10−3 mg mL−1 for Dic-PEG400-Dic, and 1 × 10−4 mg mL−1 for Dic-PEG600-Dic. The in vitro diclofenac release profile by esterase enzyme was conducted and showed almost complete conversion to free diclofenac within 35 h in the case of Dic-PEG400-Dic micelles and more than 85% of Dic-PEG600-Dic micelles. Then the anti-inflammatory activity was determined by testing the TNF-α production in LPS-stimulated Balb/c mice. Diclofenac micelles significantly suppressed TNF-α production after a 5 mg kg−1 dose was given. The developed micelles showed TNF-α inhibition up to 87.4% and 84% after 48 hours of treatment in the case of Dic-PEG400-Dic and Dic-PEG600-Dic micelles respectively in comparison to 42.3% in the case of diclofenac alone. Dic-PEG400-Dic micelles showed the most potent anti-inflammatory activity with improved TNF-α suppression through time progress. Therefore, the developed nano-micelles provide a facile synthetic approach to enhance diclofenac water solubility, improve the anti-inflammatory effect and achieve a sustained release profile to get better patient compliance.
1. Introduction
Molecular self-assembled nanomaterials are a type of well-organized nanostructures generated by spontaneous molecular arrangement using specific non-covalent interactions like electrostatic interactions, π–π stacking, hydrogen bonding, and van der Waals forces to keep these nanostructures in a stable state, achieving minimal energy in the system.1,2 The self-assembly process plays an important role in the formation of novel nanomaterials with exceptional chemical and physical properties and with various nanostructures (nanotubes, nanoparticles, micelles, vesicles, and nanofibers).3 The developed nanostructures represent a broad field of applications in chemistry, biology, physics, and medicine.4,5 Self-assembled nanomaterials-based drug delivery models have been investigated extensively as will enhance the drug therapeutic efficacy, water-solubility, biodistribution, and provide a sustained drug release profile.2,6–9 In cancer therapy, various research groups reported the utilization of the prodrug approach with polyethylene glycol to enable the self-assembly into micellar structures for passive drug delivery. For example, Shen et al. reported the conjugation of two molecules of camptothecin with an oligomer of ethylene glycol for the formation of nanocapsules.10 Another study conducted by Li et al. synthesized prodrug nanomicelles of camptothecin using two molecules of camptothecin (CPT) conjugated with polyethylene glycol (PEG) through disulfide linkage. The synthesized CPT-SS–PEG–SS-CPT was able to form nanomicelles that were selectively delivered to the cancer cells.11 Dong et al. synthesized methotrexate–PEG–methotrexate nanomicelles which showed good pharmacological efficacy on HepG2 cells.12 Herein, we almost utilized the same approach to synthesize nanomicelles for the treatment of inflammatory conditions.
Non-steroidal anti-inflammatory drugs (NSAIDs) are a class of drugs that are excessively prescribed as a curative tool for various types of inflammatory conditions. Their pharmacological activities are achieved through the inhibition of cyclooxygenase enzymes (COX-1 and COX-2) thus suppressing the biosynthesis of prostaglandins.13 However, this mechanism is also responsible for various side effects include gastrointestinal, renal, and cardiovascular risks.14 Diclofenac is among the most frequently used NSAIDs worldwide.15 It is a non-selective inhibitor of COX enzymes that belongs to the phenylacetic acid class. Besides the mentioned side effects, it has low water solubility which affects its dissolution profile and its absorption.16 Regarding the systematic toxicity, the inflammatory tissues are characterized by a leakage blood vascularity that permits the penetration and passive accumulation of nanomaterials in the inflamed tissues much more than healthy ones through the enhanced permeability and retention phenomena (EPR).17,18 Therefore, various nanoformulations of diclofenac have recently developed to improve its activity and/or reduce the undesirable side effects.7,19–22 The reported studies have shown the improvement of anti-inflammatory and the biodistribution of diclofenac by its encapsulation in polymeric nanoparticles or nanoliposomes. Herein, we developed a facile synthesis of amphiphilic structures containing diclofenac as the hydrophobic core and polyethylene glycol as the hydrophilic shell (Dic-PEG-Dic) that are capable of self-assembling to nanomicelles as shown in Scheme 1. We chose polyethylene glycol (PEG) as it has FDA approval for its utilization in pharmaceutical formulations. Moreover, because of its biocompatibility, it reduced immunogenicity and prolong the circulation time.23 These developed nanomicelles have improved the diclofenac water solubility and enhanced its anti-inflammatory activity with a sustained release profile.
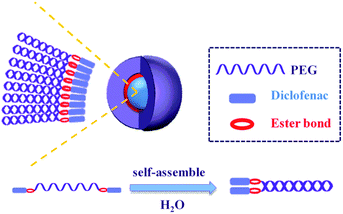 |
| Scheme 1 Graphical demonstration of the self-assembled diclofenac nanomicelles. | |
2. Experimental
2.1. Materials and instruments
Pure diclofenac powder gifted from Pharmacare Pharmaceutical Company, PEG 400, and PEG 600 were purchased from Sun Pharm Ltd. Triethylene glycol (TEG) was purchased from Merck Company. Lipopolysaccharide (LPS) from Escherichia coli, N,N-diisopropylethylamine (DIPEA), human Tumor Necrosis Factor α ELISA Kit, esterase from the porcine liver, 2-(1H-benzotriazole-1-yl)-1,1,3,3-tetramethylaminium tetrafluoroborate (TBTU), silica gel (230–400 mesh), and pyrene were supplied from Sigma-Aldrich company. Spectra/Por® 4 dialysis membrane (12–14 kDa MWCO) was used for in vitro release study.
Rotary evaporator (VV2000 OB2000, Heidolph, Germany) was used. Centrifuge (Micro CL 17R, Thermofischer Scientific, Germany), and water path sonicator (Elmasonic S 70 H, Elma®, Germany) were utilized in the preparation and dispersion of functionalized micelles. The Bruker Avance 500 spectrometer was used to record Nuclear Magnetic Resonance (NMR) spectra. Chemical shifts and coupling constants were applied in ppm, and Hz, respectively. UV/vis spectrophotometer (Jenway, UK) was utilized to record Ultraviolet-visible (UV-vis) spectrausing quartz cuvettes. Regarding the Atomic Force Microscopy (AFM) analysis, a tapping mode and Si3N4 cantilevers were used for the analysis. Fluorescence spectroscopy of Perkin Elmer Luminescence spectrometer was used.
2.2. An in-house synthetic procedure
At room temperature, diclofenac, TBTU, and DIPEA with a ratio of 1
:
1.1
:
0.75 respectively were dissolved in 3 mL dimethylformamide (DMF). The solution was stirred for 5 min, then a solution of polyethylene glycol (TEG or PEG 400 or PEG 600) and DIPEA with a ratio of 0.5
:
0.75 dissolved in DMF (3 mL) was added slowly and the reaction was stirred for 24 h. After that, the solvent was evaporated using a rotary evaporator. Then, the crude product was extracted by ether (120 mL) and washed with H2O (60 mL). After that, a drying agent (Na2SO4) was added to the organic layer followed by evaporation. Finally, the crude product was purified by column chromatography.
2.2.1. Synthesis of diclofenac-TEG-diclofenac (1). Following the general synthetic procedure, the following quantities were used: diclofenac (200 mg, 0.68 mmol), TEG (50.6 mg, 0.34 mmol), TBTU (240.1 mg, 0.75 mmol), DIPEA (177 μL, 1.01 mmol), and 6 mL of DMF to obtain compound 1 as a yellowish oil with a yield of 89% and Rf = 0.28 DCM/MeOH (9
:
1). 1H NMR (400 MHz, CDCl3): δ 7.44 (d, J = 8.3 Hz, 4H), 7.32 (d, J = 7.8 Hz, 2H), 7.28 (d, J = 8.3 Hz, 2H), 7.12–7.19 (m, 2H), 7.03 (t, J = 7.8 Hz, 2H), 6.34 (d, J = 7.8 Hz, 2H), 4.43 (s, 4H), 3.72 (s, 4H), 3.05–2.82 (m, 8H). 13C NMR (100.6 MHz, CDCl3): δ 173.6, 143.4, 135.6, 130.8, 130.5, 129.1, 128.0, 124.9, 124.4, 123.1, 109.2, 62.4, 62.2, 62.0, 35.8.
2.2.2. Diclofenac-PEG400-diclofenac (2). Following the general synthetic procedure, the following quantities were used: diclofenac (400 mg, 1.35 mmol), PEG 400 (270 mg, 0.68 mmol), TBTU (477.0 mg, 1.49 mmol), DIPEA (353 μL, 2.02 mmol), and 6 mL DMF to obtain compound 2 as a yellowish oil with a yield of 64%, and (Rf = 0.21) DCM/MeOH (9
:
1). 1H NMR (400 MHz, CDCl3): δ 7.46–7.44 (m, 4H), 7.32 (d, J = 8.3 Hz, 2H), 7.28 (d, J = 8.8 Hz, 2H), 7.14 (t, J = 7.9 Hz, 2H), 7.03 (t, J = 7.7 Hz, 2H), 6.34 (d, J = 7.8 Hz, 2H), 4.65 (s, 4H), 3.72 (4H), 3.35–3.18 (m, 30H). 13C NMR (100.6 MHz, CDCl3): δ 174.1, 143.7, 135.4, 131.2, 130.9, 129.5, 128.4, 125.3, 124.7, 123.5, 109.6, 64.4, 64.2, 64.0, 62.2, 60.4, 36.2.
2.2.3. Diclofenac-PEG600-diclofenac (3). Following the general synthetic procedure, the following quantities were used: diclofenac (400 mg, 1.35 mmol), PEG 600 (405 mg, 0.68 mmol), TBTU (477.0 mg, 1.49 mmol), DIPEA (353 μL, 2.02 mmol), and 6 mL of DMF to obtain a yellowish oil with a yield of 48%, and (Rf = 0.18) DCM/MeOH (9
:
1). 1H NMR (400 MHz, CDCl3): δ 7.45 (d, J = 8.3 Hz, 4H), 7.32 (d, J = 7.8 Hz, 2H), 7.28 (d, J = 7.8 Hz, 2H), 7.13 (t, J = 7.3 Hz, 2H), 7.03 (t, J = 7.3 Hz, 2H), 6.34 (d, J = 7.8 Hz, 2H), 4.5 (s, 4H), 3.72 (s, 4H), 3.34–3.02 (m, 48H). 13C NMR (100.6 MHz, CDCl3): δ 174.1, 143.7, 135.9, 131.2, 130.9, 129.5, 128.4, 125.3, 124.7, 123.5, 109.6, 64.1, 63.9, 63.7, 63.6, 36.2.
2.3. Formation of diclofenac micelles
The self-assembly of the micelles have been obtained by dissolving 1 mg of the synthesized derivatives in 100 mL phosphate-buffered saline (PBS) (1X, pH = 7.4), then the solution was sonicated for 30 min at 50 °C, and analyzed by AFM.
2.3.1. Analysis by AFM. Stock solutions of 1 × 10−1 mg mL−1 of diclofenac micelles were prepared by adding a sufficient amount of micelles to 100 mL volumetric flask, followed by the addition of PBS to get a final concentration of 1 × 10−1 mg mL−1. Then a drop of the stock solution was added to detached mica, and AFM analysis was performed.
2.3.2. CMC determination. Stock solutions of 1 × 10−1 mg mL−1 of diclofenac micelles were prepared. A defined amount of pyrene was dissolved in acetone (6 × 10−7 M) and was added to various volumetric flasks (10 mL) which then the acetone was evaporated to obtain sample solutions. A measured amount of stock solution was added to each flask followed by double distilled water. These flasks were heated for 2 hours at 60 °C with shaking and thenlet to cool overnight to room temperature. The micelle concentration in the sample ranged from 10−2 to 10−6 mg mL−1. For fluorescence measurements, 2 mL of sample was put in square cells, and the used λemission was 390 nm with a spectrum range of (300–450 nm). The CMC was measured as the intersection between the extrapolated intensity ratios of I336/I334 and the concentration range. The experiments were done in triplicate for statistical.
2.4. In vitro diclofenac release by esterase enzyme
A calibration curve of diclofenac dissolved in phosphate buffer saline (PBS)/1% methanol at pH = 7.4 was implemented at λmax = 275 nm to quantify the released amount of diclofenac upon hydrolysis. 3 mL of micelle solution was added with 10 U of porcine liver esterase (PLE) in dialysis membrane and then was immersed in 300 mL PBS with stirring at 37 °C, aliquots were taken, and (UV-vis) spectra analysis was performed.
2.5. In vivo anti-inflammatory activity
Balb/c mice (5–6 weeks of age) were provided from An-Najah National University animal house. The animal house provided the items after taking the required approval from the university ethical committee. The mice were kept in controlled conditions of temperature (23 ± 2 °C) and 12 h light/dark cycle under pathogen-free conditions. The animals were supplied with water and proper food. The mice were classed into fourgroups: group 1 (control) injected with PBS 200 μL after half-hour injected with lipopolysaccharide LPS 1 mg kg−1. Group 2 (positive control) mice first injected with diclofenac (immediate-release) 5 mg kg−1, after half-hour injected with LPS 1 mg kg−1 groups 3 and 4 mice injected with diclofenac micelles (5 mg kg−1) after half-hour injected with LPS 1 mg kg−1. The mice were anesthetized by isoflurane and after ninety minutes, the blood was withdrawn and centrifuged at 3000 rpm for 15 min at 4 °C. The collected serum was stored at −80 °C until analysis. Mouse TNF-α ELISA kit was used to measure the serum levels of TNF-α. The experiment was performed in triplicate and GraphPad prism 6 was used for the data analysis.
3. Results and discussion
Diclofenac suffers from low water solubility (intrinsic aqueous solubility of 1.0 ± 0.1 μg mL−1 at 25 °C) which affects its absorption.24 Moreover, it is biodistribution in the body could cause various systematic side effects. Therefore, we aimed to synthesize various diclofenac derivatives that could be self-assembled into micelle structures. This nanostructure will improve diclofenac water solubility, its accumulation in the inflammation site, and provide a sustained release system. The synthesis is simply achieved by double esterification of two molecules of diclofenac with three different polyethylene glycol–PEG–(triethylene glycol, PEG 400, and PEG 600). Polyethylene glycol is a biocompatible polymer that has FDA approval for its utilization in pharmaceutical formulations. The simple esterification reaction was achieved by using hydrolyzable ester bond formation between the PEG and diclofenac using the coupling agent TBTU and Hünig's base (DIPEA)25,26 as shown in Scheme 2.
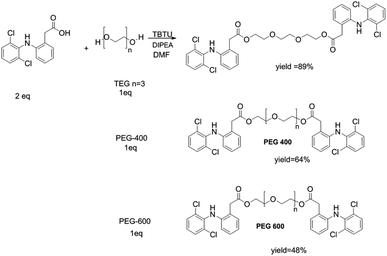 |
| Scheme 2 Synthetic scheme of the different amphiphiles of diclofenac with TEG, PEG 400, or PEG 600. | |
The structure of the synthesized compounds was confirmed by NMR analysis as it is a powerful tool for structural elucidation and was used previously to characterize drug-PEG conjugates.10–12 As can be observed in Fig. 1, the spectra showed upfield shifts of the diclofenac (Dic) as well as the polyethylene glycol protons upon their interaction. The 1H NMR spectra of the conjugates contain all expected resonance peaks characteristic for PEG and diclofenac, including the –CH of diclofenac in a range of 6–7.5 ppm and the –CH2– of TEG at 3.5–4.5 ppm (Fig. 1a), for Dic-PEG400-Dic 2.5–3.5 ppm (Fig. 1b), and Dic-PEG600-Dic 3–3.5 ppm (Fig. 1c).
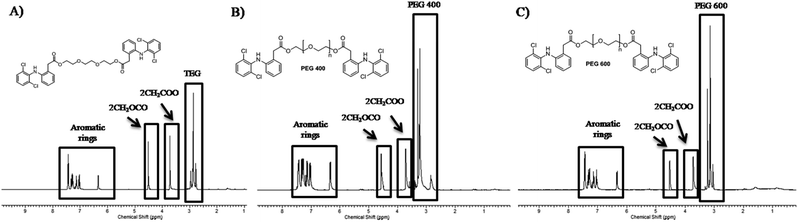 |
| Fig. 1 Representative 1H NMR spectra of (A) Dic-TEG-Dic; (B) Dic-PEG400-Dic; (C) Dic-PEG600-Dic. | |
Once the three diclofenac derivatives have successfully been synthesized, they were analyzed for their ability to form micelles by atomic force microscopy (AFM). The micelle formation was achieved by the simple self-assembling of amphiphilic structures in water. Fig. 2 showed the successful formation of micellar structures in the case of Dic-PEG400-Dic and Dic-PEG600-Dic whereas failed in Dic-TEG-Dic. This could be explained as the spherical shape of the micelle is consisting of the hydrophobic micelle core (two-terminal diclofenac molecules) and a hydrophilic moiety of polyethylene glycol derivative that form the shell of the nanomicelles which interacts with the aqueous medium. Depending on the hydrophilic shell of the polyethylene glycol, the size of the grown nanomicelles will be formed. The dominant factor in the consideration of micelle shape is the fact that one dimension of the hydrophobic core cannot exceed the length of the hydrophilic moiety. These results are coincided with previously published work by Li et al. who utilized camptothecin or methotrexate drugs as the hydrophobic core and the PEG as a hydrophilic shell that formed the nanomicelles.11,12 This immediately precludes the possibility that micelles are normally observed in Dic-PEG4000-Dic and Dic-PEG600-Dic, but it is failed in the case of Dic-TEG-Dic to form micelle due to its short hydrophilic chain. Therefore, we presumed that the hydrophobic moiety (diclofenac) will be self-assembled to form the core of the micelles and the hydrophilic chain is extended out to the surface of the micelle as shown in Scheme 1. Fig. 2 illustrates the formation of a spherical micellar structure with a mean diameter of 200 nm in the case of Dic-PEG400-Dic (Fig. 2A) and 110 nm micelle size of Dic-PEG600-Dic (Fig. 2B). Moreover, Dic-PEG400-Dic has a water solubility of 1 mg mL−1 while Dic-PEG600-Dic has a water solubility of 1.2 mg mL−1 at 25 °C.
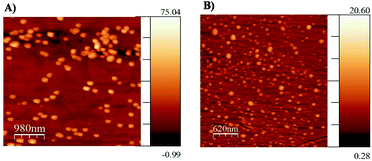 |
| Fig. 2 Atomic force microscopy for (A) Dic-PEG400-Dic; (B) Dic-PEG600-Dic. | |
The designed structure of Dic-PEG400-Dic and Dic-PEG600-Dic implied amphiphilic properties of the synthesized micelles. Therefore, it is essential to determine the critical micelle concentration (CMC) of these amphiphilic molecules that are required to form stable micelles. The aggregation behavior of aqueous micelle solutions was determined by fluorescence spectroscopy using pyrene as a probe.27–30 As shown in Fig. 3, the CMC of Dic-PEG400-Dic was determined as 2.7 × 10−3 mg mL−1, whereas it was 1 × 10−4 mg mL−1 for Dic-PEG600-Dic. As seen the intensity below a certain concentration “2.7 × 10−3 mg mL−1 for Dic-PEG400-Dic, 1 × 10−4 mg mL−1 for Dic-PEG600-Dic” stay constant. After that concentration, the intensity increases as the log concentration increases which explains the formation of the nanomicelles and the distribution of pyrene between the aqueous and micelle phase. Pyrene has very small absorption at 333 nm in water, which increases dramatically when transfer to the less polar micelle domain at 336 nm.31,32 Then, from the intersection of the twostraight lines, the CMC was determined. The CMC values of Dic-PEG400-Dic and Dic-PEG600-Dic nanomicelles were low, which indicates that the nanomicelles are highly stable in an aqueous environment especially for in vivo application.33
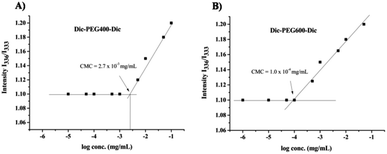 |
| Fig. 3 Critical micelle concentrations of (A) Dic-PEG400-Dic; (B) Dic-PEG600-Dic. | |
To determine the in vitro release profile of diclofenac from the formed micelles upon their incubation with the esterase enzyme, we have constructed an adequate calibration curve of diclofenac at λmax = 275 nm with R2 = 0.998 and we chose porcine liver esterase (PLE) as a model esterase for proof of concept studies at a concentration of 10 U mL−1, where this enzyme activity would be comparable to levels found in mouse serum.7,34,35 In the absence of PLE, diclofenac micelles alone were stable in PBS buffer (pH 7.4) and no hydrolysis was observed in 5 days. In the presence of 10 U mL−1 PLE, conversion of diclofenac micelles to diclofenac was proved by UV-vis spectroscopy illustrated by the concomitant increase of the diclofenac peak at λmax. The release profile in terms of the relative amount of diclofenac released from the micelle was completed within 2 days. After 24 h, more than 96% was released from Dic-PEG400-Dic derivative micelles and more than 85% of Dic-PEG600-Dic derivative was converted within 30 hours as shown in Fig. 4. We noticed that the release of diclofenac from micelles was slow with a sustained release manner as the polyethylene glycol chain could hinder the esterase enzyme activity to hydrolyze the ester bond.
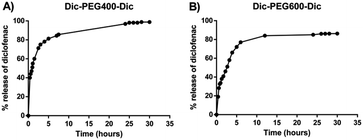 |
| Fig. 4 In vitro release of diclofenac from (A) Dic-PEG400-Dic; (B) Dic-PEG600-Dic. | |
Finally, to identify the anti-inflammatory activity of diclofenac derivatives, an in vivo study on Balb/c mice was conducted to test the activity of synthesized diclofenac micelles in comparison to the activity of diclofenac alone. Lipopolysaccharides (LPS) are well-known that stimulate the immune system in normal mammalian cells strongly. Therefore, different proinflammatory cytokines, including TNF-α and IL-1 are released in response to LPS that areengaged in pathogen clearance.36 TNF-α has several biological processes including the regulation and production of various inflammatory mediators and can indicate the severity of inflammation. In this study, the mice were injected with LPS to initiate the inflammation process and the level of TNF-α was measured after the treatment of diclofenac alone or the synthesized micelles (Dic-PEG400-Dic and Dic-PEG600-Dic). In this in vivo study and after the acute inflammation triggered by LPS, we observed a noticeable drop in the TNF-α release in mice serum upon the treatment of diclofenac or diclofenac micelles in all treated mice at various time points as shown in Fig. 5.
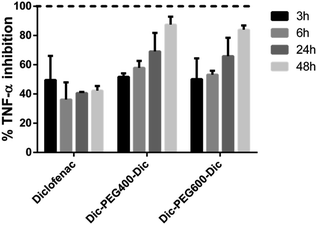 |
| Fig. 5 Percentage of TNF-α inhibition after treatment of diclofenac, Dic-PEG400-Dic, and Dic-PEG600-Dic at four different time intervals (3 h, 6 h, 24 h, 48 h). | |
In Fig. 5, it can be observed that the inhibited amount of TNF-α in the case of diclofenac alone was almost constant at different time intervals with an average of inhibition by 49.5%, 36.1%, 40.6%, and 42.3% at 3, 6, 24, and 48 h, respectively. However, in the case of Dic-PEG400-Dic, the average percentage of inhibition of TNF-α was 51.8%, 57.9%, 69.1%, and 87.4% whereas in the case of Dic-PEG600-Dic was 50.1%, 53.2%, 65.9%, and 84% at 3, 6, 24, and 48 h, respectively. Therefore, both diclofenac micelles showed a superior inhibition of TNF-α up to 87.4% and 84% after 48 hours of treatment whereas 42.3% in the case of diclofenac alone. Moreover, the inhibition of TNF-α has increased in both diclofenac micelles upon the increasing of the treatment duration. This observation is due to the sustained release behavior of diclofenac from the developed micelles as shown in Fig. 4. Therefore, these micelles showed an enhancement of the anti-inflammatory activity in comparison to the drug alone with a slow-release profile that improves patient compliance.
4. Conclusions
We have successfully synthesized two amphiphilic structures of diclofenac by simply attaching polyethylene glycol derivatives to improve diclofenac water solubility, anti-inflammatory activity as well as obtaining a sustained release profile. The atomic force microscope study confirms the formation of a spherical micellar structure. Moreover, a sustained release behavior has been obtained of diclofenac as obtained by the in vitro release study with an esterase enzyme. Finally, the in vivo study showed the improvement of the diclofenac anti-inflammatory activity as the micellar derivatives decreased the TNF-α production up to 87% in LPS-stimulated Balb/c mice. Therefore, the developed nanomicelles enhanced the anti-inflammatory activity of the diclofenac with a sustained release manner which will improve patient compliance.
Conflicts of interest
There are no conflicts to declare.
Acknowledgements
The authors acknowledge Hamdi Mango center at the University of Jordan for NMR analysis.
Notes and references
- N. Habibi, N. Kamaly, A. Memic and H. Shafiee, Nano Today, 2016, 11, 41–60 CrossRef CAS PubMed.
- S. Yadav, A. K. Sharma and P. Kumar, Front. Bioeng. Biotechnol., 2020, 8, 127 CrossRef PubMed.
- J. Castillo-Leon, K. B. Andersen and W. E. Svendsen, in Biomaterials Science and Engineering, ed. R. Pignatello, Intechopen, United Kingdom, 2011, ch. 5, pp. 115–138 Search PubMed.
- P. Xing and Y. Zhao, Adv. Mater., 2016, 28, 7304–7339 CrossRef CAS PubMed.
- E. Busseron, Y. Ruff, E. Moulin and N. Giuseppone, Nanoscale, 2013, 5, 7098 RSC.
- M. Assali, N. Kittana, S. A. Qasem, R. Adas, D. Saleh, A. Arar and O. Zohud, RSC Adv., 2019, 9, 1055–1061 RSC.
- M. Assali, R. Shawahna, S. Dayyeh, M. Shareef and I.-A. Alhimony, Eur. J. Pharm. Sci., 2018, 122, 179–184 CrossRef CAS PubMed.
- M. Assali, A. N. Zaid, M. Bani-Odeh, M. Faroun, R. Muzaffar and H. Sawalha, Int. J. Polym. Mater. Polym. Biomater., 2017, 66, 717–725 CrossRef CAS.
- H. Lu, J. Wang, T. Wang, J. Zhong, Y. Bao and H. Hao, J. Nanomater., 2016, 2016, 1–12 Search PubMed.
- Y. Shen, E. Jin, B. Zhang, C. J. Murphy, M. Sui, J. Zhao, J. Wang, J. Tang, M. Fan, E. Van Kirk and W. J. Murdoch, J. Am. Chem. Soc., 2010, 132, 4259–4265 CrossRef CAS PubMed.
- X.-Q. Li, H.-Y. Wen, H.-Q. Dong, W.-M. Xue, G. M. Pauletti, X.-J. Cai, W.-J. Xia, D. Shi and Y.-Y. Li, Chem. Commun., 2011, 47, 8647 RSC.
- H. Dong, C. Dong, W. Xia, Y. Li and T. Ren, MedChemComm, 2014, 5, 147–152 RSC.
- J. R. Vane and R. M. Botting, Am. J. Med., 1998, 104, 2S–8S CrossRef CAS PubMed.
- S. Harirforoosh, W. Asghar and F. Jamali, J. Pharm. Pharm. Sci., 2014, 16, 821 Search PubMed.
- F. M. Turnbull, P. McGettigan and D. Henry, PLoS Med., 2013, 10, e1001388 CrossRef PubMed.
- A. Llinàs, J. C. Burley, K. J. Box, R. C. Glen and J. M. Goodman, J. Med. Chem., 2007, 50, 979–983 CrossRef PubMed.
- K. Greish, H. Nehoff, N. Parayath, L. Domanovitch and S. Taurin, Int. J. Nanomed., 2014, 2539 CrossRef PubMed.
- L. K. Prasad, H. O'Mary and Z. Cui, Nanomedicine, 2015, 10, 2063–2074 CrossRef CAS PubMed.
- H. Al-Lawati, M. R. Vakili, A. Lavasanifar, S. Ahmed and F. Jamali, J. Pharm. Sci., 2019, 108, 2698–2707 CrossRef CAS PubMed.
- S. S. Guterres, H. Fessi, G. Barratt, F. Puisieux and J. P. Devissaguet, J. Biomater. Sci., Polym. Ed., 2012, 11, 1347–1355 CrossRef PubMed.
- M. Hakim, J. Z. Goh, S. N. Tang, H. S. Chiong, Y. K. Yong and A. Zuraini, Int. J. Nanomed., 2015, 10, 297–303 Search PubMed.
- R. Jukanti, G. Devaraj, R. Devaraj and S. Apte, Int. J. Pharm., 2011, 414, 179–185 CrossRef CAS PubMed.
- F. M. Veronese and A. Mero, BioDrugs, 2008, 22, 315–329 CrossRef CAS PubMed.
- A. Llinàs, J. C. Burley, K. J. Box, R. C. Glen and J. M. Goodman, J. Med. Chem., 2007, 50, 979–983 CrossRef PubMed.
- J.-d. A. K. Twibanire and T. B. Grindley, Org. Lett., 2011, 13, 2988–2991 CrossRef CAS.
- R. Knorr, A. Trzeciak, W. Bannwarth and D. Gillessen, Tetrahedron Lett., 1989, 30, 1927–1930 CrossRef CAS.
- M. Assali, N. Kittana, S. A. Qasem, R. Adas, D. Saleh, A. Arar and O. Zohud, RSC Adv., 2019, 9, 1055–1061 RSC.
- J. W. Bae, E. Lee, K. M. Park and K. D. Park, Macromolecules, 2009, 42, 3437–3442 CrossRef CAS.
- C. L. Zhao, M. A. Winnik, G. Riess and M. D. Croucher, Langmuir, 1990, 6, 514–516 CrossRef CAS.
- M. Wilhelm, C. L. Zhao, Y. Wang, R. Xu, M. A. Winnik, J. L. Mura, G. Riess and M. D. Croucher, Macromolecules, 1991, 24, 1033–1040 CrossRef CAS.
- I. Astafieva, X. F. Zhong and A. Eisenberg, Macromolecules, 1993, 26, 7339–7352 CrossRef CAS.
- J. Cao, A. Lu, C. Li, M. Cai, Y. Chen, S. Li and X. Luo, Colloids Surf., B, 2013, 112, 35–41 CrossRef CAS PubMed.
- H. Su, F. Wang, W. Ran, W. Zhang, W. Dai, H. Wang, C. F. Anderson, Z. Wang, C. Zheng, P. Zhang, Y. Li and H. Cui, Proc. Natl. Acad. Sci. U. S. A., 2020, 117, 4518–4526 CrossRef CAS PubMed.
- B. M. Liederer and R. T. Borchardt, J. Pharm. Sci., 2006, 95, 1177–1195 CrossRef CAS PubMed.
- M. Assali, M. Joulani, R. Awwad, M. Assad, M. Almasri, N. Kittana and A. N. Zaid, ChemistrySelect, 2016, 1, 1132–1135 CrossRef CAS.
- J. E. Parrillo, N. Engl. J. Med., 1993, 328, 1471–1477 CrossRef CAS PubMed.
Footnote |
† Electronic supplementary information (ESI) available. See DOI: 10.1039/d1ra03804d |
|
This journal is © The Royal Society of Chemistry 2021 |
Click here to see how this site uses Cookies. View our privacy policy here.