DOI:
10.1039/D1RA05084B
(Paper)
RSC Adv., 2021,
11, 32841-32851
Trace carbonyl analysis in water samples by integrating magnetic molecular imprinting and capillary electrophoresis†
Received
1st July 2021
, Accepted 21st September 2021
First published on 6th October 2021
Abstract
In order to obtain high derivatization efficiency, the overuse of derivative agent 2,4-dinitrophenylhydrazine (2,4-DNPH) is necessary for carbonyl detection. But, the 2,4-DNPH residue will cause background interferences and limit the pre-concentration factor of the target analytes. In order to overcome the bottleneck problems, the magnetic molecularly imprinted polymer based solid-phase extraction (MMIPs-SPE) method was developed with 2,4-dinitroaniline (2,4-DNAN) as the dummy template. The characteristics and selectivity of the MMIPs were investigated. Under the optimized conditions, the enrichment of carbonyls-DNPH derivatives with simultaneous removal of the surplus 2,4-DNPH was achieved. By coupling with capillary electrophoresis (CE), a satisfactory analytical performance was obtained with the detection limit ranging from 1.2 to 8.7 μg L−1 for 8 carbonyls. The MMIPs-SPE-CE method was applied successfully for the carbonyl assessment in stream water, tap water and bottled water. In addition, the migration of carbonyls in bottled drinking water was investigated under UV irradiation and heating.
1. Introduction
With the development of industry, the deterioration of water quality is becoming more and more serious. Among the contaminants, the carbonyl pollutants in water bodies have aroused wide attention. The carbonyls may result from industrial discharging, atmospheric dry/wet deposition, ozonation/chlorination disinfection, or the release of polyacetal plastic fittings and paint coating.1–3 Various efforts have been carried out for the determination of the aldehydes and ketones in aqueous samples. The more frequently used detection methods include spectrophotometry,4 gas chromatography-mass spectrometry (GC-MS)5,6 and high-performance liquid chromatography (HPLC).7–10 Owing to the high sensitivity and easy operation, HPLC is more preferable in the standard methods, such as EPA method 8315A.11
Because carbonyls have high activity and polarity and lack fluorophore and chromophore, a derivatization process must be applied before the chromatographic detection. Usually the overdose 2,4-DNPH is applied to ensure the thorough derivatization of carbonyls into hydrazones before HPLC detection.12 The following formula shows the chemical reaction between 2,4-DNPH and carbonyl (eqn (1)).
|
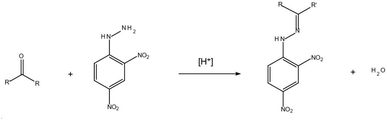 | (1) |
Considering the low levels of carbonyls, the sample enrichment step is required after the derivatization. Multiple extraction methods, such as solid-phase extraction (SPE),13,14 solid-phase microextraction (SPME),15,16 μ-SPE,17–19 liquid–liquid extraction (LLE),20 liquid-phase microextraction (LPME)21 and thin-film microextraction,22 have been developed to extract these hydrazones from water bodies. The common organic extractants used in LLE include ethyl acetate, cyclohexane, butyl acetate, dichloromethane, petroleum ether and room temperature ionic liquids, etc. The materials that are often used in SPE include C18, polydimethylsiloxane (PDMS) and resin, etc. Although the mentioned extraction methods have played positive roles in sample enrichment, the “bottleneck” problem is that they lack selectivity. Not only the carbonyls-DNPH derivatives but those unreacted 2,4-DNPH can be extracted together into the final extract solution. The excessive 2,4-DNPH residuals in the extract will result in huge chromatographic peak and extra spectra interference, causing a negative effect on the identification of the trace carbonyls-DNPH. In addition, the existence of too much surplus 2,4-DNPH may limit the enrichment factor for the target aldehydes and ketones, because a large volume of solvent is needed to dissolve all the compounds in the extract. Otherwise, the high levels of 2,4-DNPH residuals will precipitate and consequently cause the co-precipitation of carbonyls-DNPH. Therefore, removing the surplus 2,4-DNPH from the carbonyls-DNPH solution is crucial to improve the detection accuracy and sensitivity.
In order to reduce the background interferences caused by the surplus 2,4-DNPH, it is necessary to develop the special extraction materials with molecular recognition properties. Molecularly imprinted polymers (MIPs) with tailor-made binding sites are able to recognize the target molecules in terms of the unique molecular size, chemical functionalities, and stereostructure. The combination of MIPs and magnetic nano-particles gives birth to magnetic MIPs (MMIPs), which have significant advantages in real sample preparation. In addition to the recognition specificity, the feasibility of recovering MMIPs by means of an external magnetic field makes MMIPs the optimal adsorbent for dispersive solid-phase extraction (DSPE). Since they can eliminate the need for a centrifugation step, shorten the separation time, and reduce the loss of analytes during the separation, the MMIPs-SPE methods have attracted extensive attention in various fields.23–25 However, the leakage of template molecules remained a problem. Generally, about 5% of the template molecules cannot be removed from the synthetic MIP matrix by elution.26 These template molecules will leak out gradually into sample solution in the subsequent application process, and affect the analytical accuracy. To avoid this problem, the dummy template technology has been proposed. That is, the structural analogue of the target molecule is used as the imprinting template to prepare the molecular recognition binding sites. Owing to the structure similarity between the target molecule and the dummy imprinting template, the target molecules can be bound specifically by the resulted MMIPs. And at the same time, the interferences caused by the template leakage can be avoided by this way. In this paper, 2,4-dinitroaniline (2,4-DNAN), a structural analogue of 2,4-DNPH, was exploited as the substitute template to fabricate MMIPs.
Since acetone-DNPH and acrolein-DNPH are difficult to be separated from each other by HPLC. Thus, in this work the micellar electrokinetic chromatography (MEKC) was utilized to improve the separation resolution. MEKC is a modified capillary electrophoretic (CE) method with pseudo-stationary phase. Through distribution of analytes between micelles and the free solution, MEKC can exhibit the strong separation ability to either the charged or neutral molecules.27 Besides the improvement of the separation resolution, CE methods are preferable to HPLC in terms of low organic solvent consumption, small injection volume and high cost effectiveness, so they are suitable for the in-field monitoring.28,29 They have displayed the priority on the metabolite identification,29 chiral separation,30 enantiomeric resolution,31,32 metal ion speciation,33 microbiological diagnostics27,34 and so on in various fields, such as drug analysis, life science, environmental monitor and food control. We have ever developed a MMIPs based SPE-CE method for the continuous monitoring of pollutants on ambient PM 2.5, making use of the specific extraction capacity of MMIPs for the template molecules. The concentrations and gas-to-particle ratios of carbonyls in normal days or haze days were determined.35
In the present work, the MMIPs were applied in a different and more convenient way (Fig. S1†) compared to the previous work. Here, the ability of MMIPs to selectively release the non template molecules was utilized. Firstly, the carbonyls in water sample were derivatized by using 2,4-DNPH. Then, both the surplus 2,4-DNPH and carbonyls-DNPH derivatives were extracted onto the MMIPs from water body. After that, carbonyls-DNPH derivatives were washed out for further enrichment and CE analysis, while leaving 2,4-DNPH retained by the MMIPs owing to the imprinting effect. The target carbonyls in the present research include eight kinds of low molecular-weight carbonyls. They are formaldehyde, acetaldehyde, acetone, acrolein, propionaldehyde, trichloroacetaldehyde, butyraldehyde, and benzaldehyde. The MMIPs-SPE-CE method was applied for the enrichment and analysis of trace carbonyls in the stream water, tap water and bottled drinking water samples. In addition, the migration of carbonyls in bottled drinking water was investigated under the UV irradiation and heating. The analytical performance of the method was presented and discussed in detail. The schematic of the method is shown in Scheme 1.
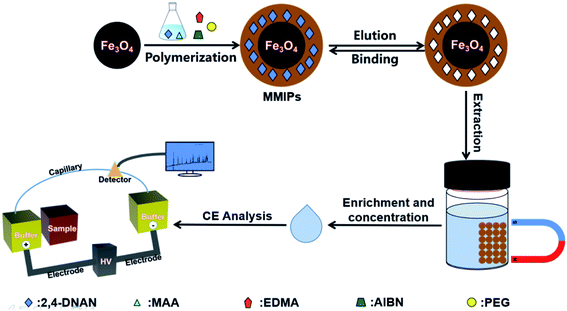 |
| Scheme 1 Schematic for the present work. | |
2. Experimental
2.1 Chemicals and reagents
Ferric chloride hexahydrate (FeCl3·6H2O), ferrous chloride tetrahydrate (FeCl2·4H2O) sodium hydroxide (NaOH), azobisisobutyronitrile (AIBN) and methacrylic acid (MAA) were obtained from Tianjin Damao Chemical Reagent Co., Ltd. (China). Sodium tetraborate decahydrate (Borax) and 2,4-DNPH were obtained from BDH Chemicals Co., Ltd (England). Sodium dodecyl sulfate (SDS) and 2,4-dinitroaniline (2,4-DNAN) were obtained from Aldrich Chemical Co., Ltd. (USA). HPLC-grade acetonitrile (ACN) was obtained from Shanghai ANPEL Scientific Instrument Co., Ltd. (China). Ethylene glycol dimethacrylate (EDMA) was obtained from Tokyo Chemical Industry Co., Ltd. (Japan). Acetic acid, polyethylene glycol (PEG), ethanol, acetonitrile, methanol, citric acid, phosphoric acid, trisodium citrate dihydrate, toluene and sodium chloride were obtained from Tianjin Zhiyuan Chemical Reagent Co., Ltd. (China). All chemical reagents were of AR grade and were used without further purification.
2.2 Apparatus
The field emission scanning electron microscope (JSM-7001F, JEOL, Japan) was used for the scanning electron microscopy (SEM) analysis. The CE experiments were carried out on the TriSep-3000 system (Unimicro Technologies, Pleasanton, CA, USA) equipped with a UV/Vis detector and a fused silica capillary (80 cm × 50 μm i.d., 51 cm to detector). A syringe pump (Legato 210, KD scientific, USA) was used for the capillary pretreatment. HPLC analysis was performed by Agilent 1200 (Agilent, USA).
2.3 Optimization of the adsorption experiments
With 2,4-DNAN as the template, MAA as functional monomer, EDMA or DVB as the cross-linker, the MMIPs were prepared with Fe3O4 as the magnetic core. The detailed procedure for preparation of MMIPs is described in the ESI† (Synthesis of MMIPs). The standard solution of 2,4-DNPH and 8 carbonyls-DNPH, including formaldehyde-DNPH, acetaldehyde-DNPH, acetone-DNPH, acrolein-DNPH, propionaldehyde-DNPH, trichloroacetaldehyde-DNPH, butyraldehyde-DNPH, and benzaldehyde-DNPH were prepared with each concentration at 5 mg L−1. Then, some amount of MMIPs were incubated in 100 mL of the above standard solution respectively. After shaking for some time, the microspheres were removed by the external magnet. And the supernatant was measured with an UV-Vis spectrometer at 360 nm.
2.4 The derivatization procedures
To derivatize the carbonyls into hydrazones, 2.5 mL citric acid/sodium citrate buffer solution (1 M, pH = 3) and 1 mL HCl-DNPH solution (500 mg L−1) were added into 50 mL water sample. After being mixed completely, the mixture was heated with water bath at 40 °C and stirred for 1 h. When cooling to room temperature, the solution was filtered through a 0.45 μm membrane filter.
2.5 MMIPs-SPE procedures
Ten mg MMIPs were added into 50 mL of the derived water sample. After shaking in the water sample for 10 minutes, the MMIPs were collected by the external magnet and dispersed in 10 mL toluene. Then, after shaking in toluene for 10 min, the MMIPs and toluene were collected separately by using the external magnet. The MMIPs were rinsed with 5 mL toluene. The above toluene extracts were combined and dried with a rotary evaporator. The products were then dissolved with 50 μL acetonitrile and mixed with 20 mM borate by 1
:
1 (v/v) prior to CE separation with conditions listed in the ESI† (MEKC separation).
2.6 Regeneration of MMIPs
The collected MMIPs were eluted with methanol/acetic acid solution (8/2, v/v) repeatedly to remove the 2,4-DNPH until no 2,4-DNPH can be detected in the extract at 360 nm with UV spectrometer. After being washed with deionized water and ethanol in sequence, the polymers were dried at 60 °C and stored for the future use.
2.7 LLE procedures
Fifty mL of the derived water sample and 5 mL saturated NaCl solution were mixed in the separating funnel, and then extracted 3 times with dichloromethane. During each extraction process, 10 mL dichloromethane was used. The resulted extracts were combined and evaporated to dryness with a rotary evaporator, and then dissolved with 200 μL acetonitrile before CE separation.
2.8 Real sample analysis
Dongjiang River provides the drinking water for more than 40 million residents in Hong Kong, Guangzhou, Heyuan, Huizhou and Dongguan. To explore the water safety, the content of carbonyls at the source of Dongjiang River, the tap water in a Dongjiang village, the tap water of Guangzhou University, and the commercially bottled water of a certain brand were analyzed. In order to investigate the migration of carbonyls from the PET bottle materials, the bottled water samples were exposed to the UV irradiation at 60 °C for 1 hour, 12 hours and 24 hours, respectively. And then the water samples were derived and analyzed with the developed MMIPs-SPE-CE method.
3. Results and discussion
3.1 Preparation and characterization of MMIPs
As mentioned in the introduction, overuse of the derivative agent 2,4-DNPH will cause background interferences and limit the pre-concentration factor of the target analytes. In order to overcome the bottleneck problems, the MMIPs-SPE-CE method was developed in the present work. In consideration of the possible side reaction between 2,4-DNPH and the intermediates during polymerization, and in order to avoid the problems caused by the template leaking in the subsequent application, 2,4-DNAN, the structure analogue of 2,4-DNPH, was used as the dummy imprinting template in the work. By this way, the spectra interference can also be avoided, since there is a big difference between the maximum absorption wavelength of 2,4-DNAN (338 nm) and carbonyls-DNPH derivatives (360 nm).
In order to remove the surplus 2,4-DNPH efficiently and at the same time improve the recovery of the target analytes, the synthesis and extraction conditions have been optimized. For the preparation of MIPs, EDMA and DVB are often used as the cross-linkers. It is known that EDMA is hydrophilic and possesses the flexible configuration, while DVB is hydrophobic and has the more rigid structure in comparison with EDMA. To investigate whether the hydrophilic/hydrophobic properties of MMIPs can affect the molecular recognition and extraction efficiency, the MMIPs-DVB and MMIPs-EDMA have been synthesized with DVB and EDMA as the cross-linker respectively. Both kinds of the prepared MMIPs have good magnetic properties. They can be isolated from the solution quickly under the external magnetic field.
The TEM and SEM experiments were used to study the structure of MMIPs (Fig. S2†). The particle size of Fe3O4 is about 15 nm, and the iron core is surrounded by MIP shell with thickness of lower than 10 nm for both kinds of MMIPs. The MMIPs agglomerate to some extent. The average diameter of MMIPs-EDMA and MMIPs-DVB are about 1.65 μm and 40 nm respectively. For MMIPs-EDMA, both the magnetic core and the polymer shell are hydrophilic, so the MMIPs-EDMA particles tend to agglomerate together to form larger clusters. As to MMIPs-DVB, the magnetic core is hydrophilic, but the polymer shell is hydrophobic and more rigid than that of MMIPs-EDMA, so they are not easy to agglomerate together. Consequently, the diameter of MMIPs-DVB is much smaller than MMIPs-EDMA.
When the two kinds of MMIPs (10 mg each) were dispersed respectively into 100 mL 2,4-DNPH aqueous solution (5 mg L−1), the adsorption equilibrium of MMIPs-EDMA was achieved within 10 minutes; while it took about 50 min to reach the adsorption equilibrium for MMIPs-DVB (Fig. 1). Since MMIPs-EDMA can be dispersed evenly in the water body, it can reach the adsorption equilibrium more rapidly than the hydrophobic MMIPs-DVB particles. Although the maximum adsorption efficiency of MMIPs-DVB is a little higher than MMIPs-EDMA, the MMIPs-EDMA are selected in the present work to shorten the time of sample pretreatment.
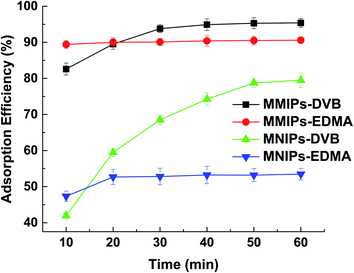 |
| Fig. 1 Adsorption kinetics of different adsorbent to 2,4-DNPH in water (sample volume: 50 mL; adsorbent: 10 mg; 2,4-DNPH: 5 mg L−1). | |
The magnetic non-imprinted polymers (MNIPs) were also prepared with procedures same as MMIPs-EDMA and MMIPs-DVB respectively, except that the template molecules were not included in the polymers. From Fig. 1, it can be seen that the adsorption efficiency of the MNIPs is much lower than the corresponding MMIPs due to the absence of the molecular imprinting sites. It can also be found that there is a significantly higher non-specific interaction for MNIPs when using DVB as a cross-linker than using EDMA. Since DVB molecule contains benzene ring, it has stronger hydrophobicity than EDMA. Moreover, the particle size of the polymers using DVB as cross-linker is smaller than those using EDMA. The larger specific surface area and stronger hydrophobicity of MNIPs-DVB will lead to an increase in the absorption of the hydrophobic compounds, thus exhibiting the higher non-specific interaction than MNIPs-EDMA.
The loading capacity of MMIPs-EDMA was estimated by the scatchard analysis (Fig. S2†). According to the Scatchard equation: Q/[Ce] = (Qmax − Q)/KD, where Ce is the equilibrium concentration, Q the amount of 2,4-DNPH bound to the MMIPs at equilibrium, Qmax the apparent maximum adsorption capacity, and KD the equilibrium dissociation constant, Qmax and KD can be determined from the intercept and slope, respectively, when Q/[Ce] is plotted versus Q. Results indicates that there are two different types of binding sites. Their maximum absorptive capacity are 104.6 mg g−1 and 1.277 mg g−1, respectively. These recognition sites contribute for the high rebinding affinity towards 2,4-DNPH.
3.2 Optimization of the extraction conditions
During the study, it is found that the MMIPs cannot discriminate 2,4-DNPH from the carbonyls-DNPH in the aqueous medium. In water, both 2,4-DNPH and carbonyls-DNPH can be adsorbed by MMIPs. The adsorption equilibrium can be achieved within 10 min with extraction efficiency above 90% for all the compounds. Results indicate that the molecular recognition properties of MMIPs have been inhibited by water. It is known that the recognition of MMIPs is commonly determined by the synergistic effect of electrostatic interaction and hydrogen bond between the target molecules and the functional groups of the MMIPs. The selectivity of MMIPs generally appears in the non-polar or low polar environment. Polar solvents (such as water) will weaken the hydrogen bond, thus leading to poor recognition properties. However, the nondiscrimination of MMIPs against carbonyls-DNPH in water is advantageous for the extraction and enrichment of the carbonyl derivatives from water samples. Basing on the principle of similar dissolve mutually, the organic carbonyls-DNPH can be adsorbed together with 2,4-DNPH on the MMIPs matrix from the water matrix.
In order to further isolate the surplus 2,4-DNPH from carbonyls-DNPH and realize the purification of carbonyls-DNPH, the next step is to find a proper organic solvent to wash the MMIPs. With this solvent these carbonyls-DNPH derivatives are expected to be washed out from the MMIPs, while the 2,4-DNPH be trapped in the MMIPs matrix owing to the imprinting effect. We have investigated the effect of the polarity of the washing solvent on the selectivity of the MMIPs. Results show that MMIPs have poor selectivity in the strong polar solvents, such as acetonitrile and methanol. The carbonyls-DNPH and 2,4-DNPH can be eluted together from MMIPs by these solvents. According to the principle of molecular recognition, the specific retention ability of MMIPs is resulted from not only the shape and size complementarity but also the hydrogen binding interaction between the analyte and binding sites in the polymers. Thus, the molecular recognition ability of MMIPs is stronger in aprotic or low polar organic solvents than in polar solvent. In polar solvent the molecular interaction between 2,4-DNPH and MMIPs is weakened, thus 2,4-DNPH can be eluted from MMIPs together with carbonyls-DNPH. In the present work, toluene was found to be the optimal washing solvent. In toluene, the MMIPs can discriminate 2,4-DNPH from carbonyls-DNPH. Therefore, in the subsequent experiment the toluene was employed to wash the MMIPs to collect the purified carbonyls-DNPH derivatives for MEKC analysis.
Under the optimized conditions, the standard solution containing 2,4-DNPH (5 mg L−1) and eight carbonyls-DNPH (1 mg L−1 each) were treated by the MMIPs-SPE method, then analyzed by MEKC. By comparing the electropherogram before and after the treatment (Fig. 2), it can be seen that the 2,4-DNPH peak is clearly eliminated, but the carbonyls-DNPH peaks do not change significantly after the MMIPs-SPE treatment. The results are attributed to the specific selectivity of MMIPs. By washing MMIPs with toluene, all the carbonyls-DNPH can migrate from MMIPs into toluene, but 2,4-DNPH is still held by the imprinting binding sites on MMIPs. Results prove that the MMIPs-SPE method can be used to isolate 2,4-DNPH from carbonyls-DNPH solution successfully.
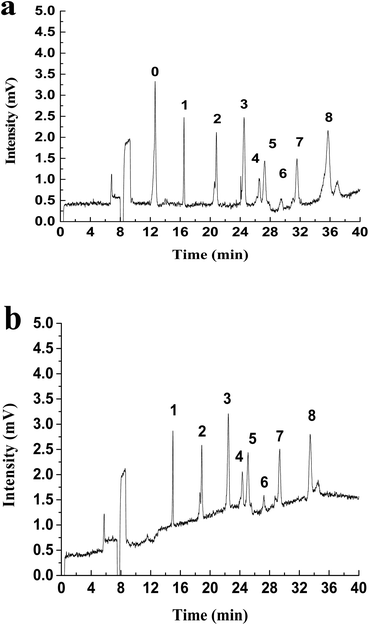 |
| Fig. 2 Electropherogram of standard mixture before (a) and after (b) MMIPs treatment. Note: the standard mixture contains 2,4-DNPH (5 mg L−1) and eight carbonyls-DNPH (1 mg L−1 each). Peaks: (0) DNPH, (1) formaldehyde, (2) acetaldehyde, (3) acetone, (4) acrolein, (5) propionaldehyde, (6) trichloroacetaldehyde, (7) butyraldehyde, (8) benzaldehyde. CE conditions: capillary 80 cm × 50 μm i.d., effective length 51 cm; separation voltage 24 kV; temperature: 20 °C, detection wavelength 360 nm, injection voltage 5 kV, injection time 3 s, buffer solution: 20 mM boric acid −50 mM sodium dodecyl sulfate (containing 15% methanol, pH 8.9). | |
In order to further optimize the experimental conditions, the effect of MMIPs dose for the adsorption was studied (Fig. 3). The recovery rate for aldehydes-DNPH and ketones-DNPH showed the similar trend. When 20 mg MMIPs were used for the adsorption in 100 mL standard solution (5 mg L−1), and then washed with 10 mL toluene, the maximum recovery rate was achieved for the target analytes. However, the further increase of the amount of MMIPs will lead to the decrease of the recovery rate, because some carbonyls-DNPH cannot be eluted sufficiently from the fluffy MMIPs by using 10 mL toluene. Thus, in the practical batch experiments, 20 mg MMIPs were used for every 100 mL water for the adsorption.
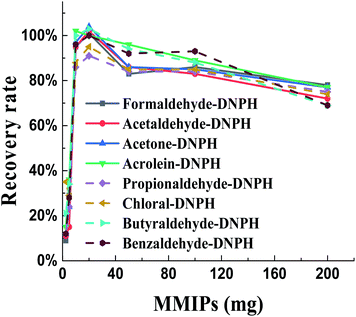 |
| Fig. 3 Effect of MMIPs-EDMA amount on the recovery rate of the carbonyls-DNPH derivatives. | |
3.3 Analytical performance
A series of standard solutions were analyzed with the MMIPs-SPE-CE method. The linear regression equations, correlation coefficients and detection limit of the method for the eight target compounds were calculated (Table 1). In the linear range, the correlation coefficients are between 0.9992–0.9999 for different compounds. Based on 3 times of signal-to-noise ratio, the limit of detection (LOD) for formaldehyde, acetaldehyde, acetone, acrolein, propionaldehyde, trichloroacetaldehyde, butyraldehyde, and benzaldehyde are ranging from 1.2 to 8.7 μg L−1.
Table 1 Analytical features of the MMIPs-CE method for the determination of carbonyl compounds
|
Analyte |
Linear range (mg L−1) |
Detection limit (μg L−1) |
Recoverya |
RSDd (n = 6) |
Repeatabilityb (n = 3) |
Intermediaryc (n = 3) |
Recoveries were evaluated by adding to drinking water samples the standard solutions of the target carbonyls-DNPH for reaching the concentration of 0.020 mg L−1 respectively. Repeatability: samples were prepared and analyzed by analyst no. 1 in triplicate. Intermediary precision: samples were prepared and analyzed by analyst no. 2 in triplicate on the following day. The relative standard deviation (RSD) is based on the 6 results from the two analysts. |
1 |
Formaldehyde-DNPH |
0.010–0.14 |
1.4 |
87.8% |
92.6% |
4.9% |
2 |
Acetaldehyde-DNPH |
0.010–0.16 |
1.2 |
96.2% |
97.6% |
3.3% |
3 |
Acetone-DNPH |
0.010–0.16 |
1.5 |
98.0% |
98.8% |
3.8% |
4 |
Acrolein-DNPH |
0.010–0.16 |
2.5 |
120.7% |
118.5% |
4.3% |
5 |
Propionaldehyde-DNPH |
0.010–0.14 |
1.5 |
93.5% |
89.9% |
4.7% |
6 |
Trichloroacetaldehyde-DNPH |
0.020–0.14 |
8.7 |
99.3% |
96.9% |
3.8% |
7 |
Butyraldehyde-DNPH |
0.020–0.16 |
1.6 |
104.0% |
97.0% |
4.8% |
8 |
Benzaldehyde-DNPH |
0.010–0.16 |
1.9 |
86.9% |
89.5% |
4.8% |
Considering the significance of water safety, the World Health Organization (WHO) has recommended that the tolerable concentration of formaldehyde in drinking water should be 2.6 mg L−1, but does not state the allowable concentrations of acetaldehyde and acetone.36 According to the quality standards for drinking water and surface water (GB 5749–2006, GB 3838–2002), the permitted concentration of formaldehyde and acetaldehyde is 0.9 mg L−1 and 0.05 mg L−1 respectively. The European Union (EU) has stipulated the specific migration restrictions for formaldehyde, acetaldehyde and acetone (expressed in mg substance per kg food). It is less than 15 mg kg−1 for formaldehyde, 6 mg kg−1 for acetaldehyde and 2 mg kg−1 for acetone.37 Thus, the present method is sensitive enough to be applied to analyze the trace levels of carbonyls in surface water and drinking water samples.
In order to investigate the determination accuracy, the recoveries were evaluated by adding to drinking water samples the standard solutions of the target carbonyls-DNPH for reaching the concentration of 0.020 mg L−1 respectively. The recovery was calculated according to eqn (2).
|
 | (2) |
The recovery experiments were firstly performed by analyst no. 1 in triplicate, and the results was used to estimate the repeatability. Intermediary precision was performed in the same way as the repeatability but with different technician on the following day. Results (Table 1) show that good recoveries have been obtained in the two systems. They are between 87.8–120.7% and 89.9–118.5% respectively for the target analytes. The relative standard deviation (RSD) based on the results from the two analysts was lower than 5% (n = 6).
LLE is the traditional method to enrich hydrazones from water. The common extractants include dichloromethane, cyclohexane, petroleum ether and ethyl acetate.38 Dichloromethane has been used as the extractant in the USEPA Method 8315A (1996).11 In order to compare with the extraction effect of MMIPs, a control test has been performed by using dichloromethane as the extractant according to the Experimental section to analyze the real stream water samples. Results (Fig. 4) show that there is a big difference between the electropherogram obtained by the two extraction methods. For the LLE treatment (Fig. 4a), there exist a huge peak of 2,4-DNPH and some tiny peaks of carbonyls-DNPH. It can be seen that the big broad peak of 2,4-DNPH in the electropherogram can interfere with the separation system. And the peaks of carbonyls-DNPH are so small that it is difficult for identification. The reason is that this LLE method has no selectivity, and the unreacted 2,4-DNPH can be extracted together with carbonyls-DNPH into the organic phase. Because of the existence of large amount of 2,4-DNPH, the volume of the solvent must be large enough to avoid the precipitation of the compounds. Thus, the trace carbonyls are difficult to be analyzed in this case.
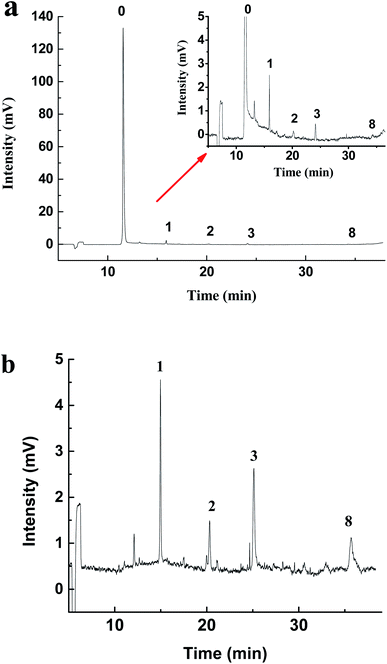 |
| Fig. 4 The electropherogram of real stream water sample treated with (a) LLE and (b) MMIPs. Peaks: (0) DNPH, (1) formaldehyde, (2) acetaldehyde, (3) acetone, (8) benzaldehyde. | |
However, the background interference caused by the excessive 2,4-DNPH can be eliminated when the MMIPs-SPE method is used. In this case, the surplus 2,4-DNPH was removed by the MMIPs, thus the extract can be concentrated by reducing the solvent volume. As shown in Fig. 4b, the huge peak of 2,4-DNPH is disappeared in the electropherogram after MMIPs treatment, and the peaks of formaldehyde, acetaldehyde, acetone and benzaldehyde are high enough for quantization. Thus, in comparison with the non-selective traditional LLE method, the MMIPs-SPE method is more favorable to the purification and enrichment of hydrazones from real samples. In addition, the MMIPs can be reused repeatedly after the regeneration with a mixture of acetic acid/methanol (2/8, v/v). After at least 6 cycles of regeneration, the MMIPs still exhibit the excellent analytical performance.
3.4 Real sample analysis
The established MMIPs-CE method was used to detect the carbonyls in various water samples with results shown in Fig. 5. Among the eight target analytes, acrolein, propionaldehyde, trichloroacetaldehyde, and butyraldehyde were not detected in any water samples. Formaldehyde, acetaldehyde and acetone were found in all the samples, and benzaldehyde was found only in the stream water.
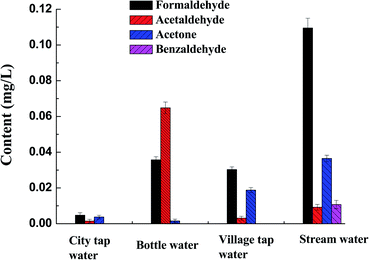 |
| Fig. 5 The distribution of carbonyl compounds in aqueous samples. | |
In the city tap water samples, a little amount of formaldehyde, acetaldehyde and acetone are found. In city, the disinfection of tap water mainly includes chlorination disinfection, chlorine dioxide disinfection, ultraviolet disinfection and ozone disinfection. These carbonyls may come from the release of polyacetal plastic fittings, or result from the degradation of some organic matters during the disinfection process.39,40 But the contents of the three carbonyls meet the demand of WHO 2011 for drinking water, EU 2011 for food, and the requirement for surface water quality (GB5749–2006, GB 3838–2002).36,37
In consideration of the health problems, increasing attention has been paid to the quality of bottled drinking water. Some diseases, including cancer and central nervous system damage were found to be related with the chemical nature of the bottled drinking water. In the present work, the bottled water sample of a brand was determined. Results show that the concentration of formaldehyde, acetaldehyde and acetone is 0.036, 0.065 and 0.0016 mg L−1 respectively. The concentration of formaldehyde and acetaldehyde in the bottled water is higher than the tap water, but still lower than the permitted migration limit of carbonyls from polyethylene terephthalate (PET) materials according to the commission regulation of EU 2011.37 Among the three carbonyls, the content of acetaldehyde is the highest. It exceeds the taste threshold of acetaldehyde, that is considered to be 20–40 μg L−1.41
The bottled water is indicated to have been produced by distillation at 105 °C. The carbonyl compounds might originate from the manufacture process, or from the thermo-oxidative and thermo-mechanical degradation of the PET bottle during storage.41–43 And the polypropylene caps could account for the trace amount of acetone in the bottled water.39,40
To simulate the effect of sunlight on the content of carbonyls in bottled water, the bottled water was exposed to the UV irradiation at 60 °C for 1 hour, 12 hours and 24 hours, respectively. And then the water samples were analyzed with the MMIPs-CE method. Results (Fig. 6) show that the continuous heating and exposure to UV light can cause the obvious increase of all the carbonyls, especially the formaldehyde and acetaldehyde.
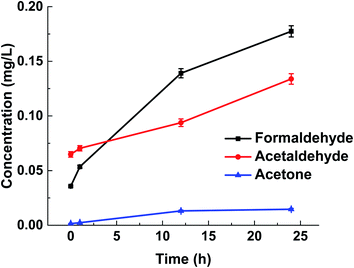 |
| Fig. 6 Effects of UV irradiation on carbonyls concentration in bottled water. | |
The treatment exerted the biggest effect on the increase of the formaldehyde concentration in this study, but the concentration of formaldehyde ranged within the permitted level for drinking water during the testing period.36 On the other hand, according to the quality standards for drinking water and surface water (GB 5749–2006, GB 3838–2002), it is noteworthy that the concentration of acetaldehyde has exceeded the allowed limit (0.05 mg L−1) by 87.6% when the exposure time is more than 12 h. Continually to increase the exposure time to 24 h, the concentration of acetaldehyde (0.13 mg L−1) has exceeded the allowable limit by 168%. The taste of the bottled water is a significant factor to estimate the quality of water. If the bottled distilled water tastes sweet, you should stop drinking it. This sweet taste is possibly caused by the excessive acetaldehyde.41 Results indicate that the PET bottled drinking water should be stored away from the heat sources and the direct exposure to sunlight to prevent the production of the harmful organic substances.
Besides the city tap water and bottled drinking water, the stream water and village tap water of Dongjiang village were also determined (Fig. 5). The content of formaldehyde, aldehyde and acetone in the collected stream water is about twice that of the village tap water. Furthermore, some benzaldehyde was found in the stream water. The higher concentration of carbonyls in the stream water may result from the wet deposition of airborne carbonyls and the degradation of humus and plants. In addition, benzaldehyde is rich in the stalk bark, leaves or seeds of some plants in the form of glycosides,44 which has possibly led to the appearance of benzaldehyde in stream water.
The village tap water comes from the pool on the mountain. After some simple purification, such as sedimentation and filtration, it is piped to the villagers' houses. The carbonyls content in the tested village tap water is higher than Guangzhou city tap water. Although the carbonyl content of the village tap water meets the requirements as drinking water, further water-treatment such as sterilization before drinking is suggested to guarantee the water safety.
3.5 Method comparison
As shown in Table S1,† various of methods have been developed to determine carbonyls in water samples. Spectrophotometry is mainly used for the determination of single aldehyde or the total aldehyde, and its sensitivity is poor.45–47 In the standard EPA method 8315a,11 the C18 SPE or LLE is coupled with HPLC. It can simultaneously determine a variety of aldehydes and ketones, but the sensitivity is lower than the MMIPs-SPE-MEKC method. With the integration of the advanced extraction and enrichment patterns, more sensitive methods, such as head space solid-phase microextraction and gas chromatography-mass spectrometry (HS-SPME-GC-MS),48 μSPE-HPLC18 and polymer monolith microextraction-HPLC (PMME-HPLC),49 have been developed for carbonyl analysis in aqueous samples. Although the sensitivity of the MMIPs-SPE-MEKC method is inferior to these extremely sensitive methods, this method has the advantages of simple pretreatment, high cost-effectiveness and suitable for field analysis. More importantly, it resolved the problems of the co-existence of 2,4-DNPH and carbonyls-DNPH together in the extract owing to the specially designed molecular recognition effect. The enrichment of carbonyls-DNPH with simultaneous removal of 2,4-DNPH can be achieved by this way. In addition, the method is not limited by the volume of water sample. The MMIPs can be dispersed and collected easily in any water samples. Thus, it is not only applicable for the treatment of the large amount of surface water samples, but also the rare biological samples, such as saliva, blood and other body fluid, for the in-field monitoring.
4. Conclusions
In this work, the efficient MMIPs-SPE-CE method have been developed for the analysis of trace carbonyls in real environmental water samples. Owing to the specially designed molecular recognition effect, it resolved the problems of the co-existence of 2,4-DNPH and carbonyls-DNPH together in the extract. With this method, the long-standing bottleneck problems caused by the overuse of the derivative agent 2,4-DNPH can be eliminated successfully, which is conductive to the sample enrichment and the improvement of the detection sensitivity. Satisfying analytical performance has been achieved with detection limits ranging from 1.2 to 8.7 μg L−1 for the 8 testing carbonyls. In the real sample analysis, good recovery, repeatability and intermediary precision has been achieved. The relative standard deviations were less than 5%. This method can be applied successfully in the investigation of the migration of carbonyls in bottled drinking water under UV irradiation and heating. After further optimization, this rapid and efficiency MMIPs-SPE-MEKC method can be extended to the analysis of other complex samples. Furthermore, this method also has potential on the analysis of other trace analytes by carefully design the imprinting molecules and operation system.
Conflicts of interest
The authors declare that they have no known competing financial interests or personal relationships that could have appeared to influence the work reported in this paper.
Acknowledgements
This work was supported by the National Natural Science Foundation of China (21477026, 21677053, 21876033), Scientific Research Projects in Colleges and Universities of Guangzhou Education Bureau (201831803) and the Undergraduate “innovation training” project of Guangzhou University (S202011078033).
References
- I. Skjevrak, A. Due, K. O. Gjerstad and H. Herikstad, Volatile organic components migrating from plastic pipes (HDPE, PEX and PVC) into drinking water, Water Res., 2003, 37, 1912–1920, DOI:10.1016/S0043-1354(02)00576-6.
- D. L. Giokas, G. Z. Tsogas and A. G. Vlessidis, On-line derivatization coupled to flow injection permanganate chemiluminescence detection of total carbonyl compounds in natural waters and drinking water, Anal. Chim. Acta, 2009, 651, 188–195, DOI:10.1016/j.aca.2009.08.028.
- A. Papageorgiou, D. Voutsa and N. Papadakis, Occurrence and fate of ozonation by-products at a full-scale drinking water treatment plant, Sci. Total Environ., 2014, 481, 392–400, DOI:10.1016/j.scitotenv.2014.02.069.
- N. K. Temel and R. Gurkan, Extraction, preconcentration, and quantification of low levels of free formaldehyde from some beverage matrices by combination of ultrasound-assisted-cloud point extraction with spectrophotometry, Food Analytical Methods, 2017, 10, 4024–4037, DOI:10.1007/s12161-017-0973-4.
- T. Dutta, K. H. Kim, R. J. C. Brown, Y. H. Kim and D. Boukhvalov, Metal-organic framework and Tenax-TA as optimal sorbent mixture for concurrent GC-MS analysis of C1 to C5 carbonyl compounds, Sci. Rep., 2018, 8, 5033, DOI:10.1038/s41598-018-23391-6.
- Y. H. Hu, M. Ning, X. Y. Zhang, Q. L. Zhu, Z. F. Tian, Y. B. Xu, C. H. Wang and Z. G. Xu, Pyrolysis products of tobacco released at different thermogravimetric stages, Tob. Sci. Technol., 2015, 48, 66–73, DOI:10.16135/j.issn1002-0861.20150312.
- M. Singh and R. Bhushan, A modification of a conventional technique for the synthesis of hydrazones of racemic carbonyls: prevention of spontaneous chiral inversion, RSC Adv., 2015, 5, 105719–105726, 10.1039/c5ra19904b.
- F. O. Santana, V. P. Campos, I. F. Santos, L. P. S. Cruz and A. V. S. Brito, Seasonal quimiometric study of formaldehyde and acetaldehyde atmospheric levels and health risk assessment, in urban areas of Salvador-Bahia, Brazil, Microchem. J., 2019, 147, 524–531, DOI:10.1016/j.microc.2019.03.069.
- T. Futagoishi, M. Murata, A. Wakamiya and Y. Murata, Encapsulation and dynamic behavior of methanol and formaldehyde inside open-cage C60 derivatives, Angew. Chem., Int. Ed. Engl., 2017, 56, 2758–2762, DOI:10.1002/anie.201611903.
- Z. Y. Liu, D. Y. Zhou, A. Li, M. T. Zhao, Y. Y. Hu, D. Y. Li, H. K. Xie, Q. Zhao, X. P. Hu, J. H. Zhang and F. Shahidi, Effects of temperature and heating time on the formation of aldehydes during the frying process of clam assessed by an HPLC-MS/MS method, Food Chem., 2020, 38, 125650, DOI:10.1016/j.foodchem.2019.125650.
- EPA: U.S, EPA Method 8315A.: Determination of carbonyl compounds by high performance liquid chromatography (HPLC), 1996, http://www.epa.gov/epawaste/hazard/testmethods/sw846/pdfs/8315a.pdf Search PubMed.
- S. Uchiyama, T. Tomizawa, Y. Inaba and N. Kunugita, Simultaneous determination of volatile organic compounds and carbonyls in mainstream cigarette smoke using a sorbent cartridge followed by two-step elution, J. Chromatogr. A, 2013, 1314, 31–37, DOI:10.1016/j.chroma.2013.09.019.
- H. Sun, J. P. Lai and Y. S. Fung, Simultaneous determination of gaseous and particulate carbonyls in air by coupling micellar electrokinetic capillary chromatography with molecular imprinting solid-phase extraction, J. Chromatogr. A, 2014, 1358, 303–308, DOI:10.1016/j.chroma.2014.06.101.
- S. M. Breckenridge, X. Yin, J. M. Rosenfeld and Y. H. Yu, Analytical derivatizations of volatile and hydrophilic carbonyls from aqueous matrix onto a solid phase of a polystyrene-divinylbenzene macroreticular resin, J. Chromatogr. B: Biomed. Sci. Appl., 1997, 694, 289–296, DOI:10.1016/S0378-4347(97)00118-7.
- C. H. Ma, J. J. Ji, C. Tan, D. M. Chen, F. Luo, Y. R. Wang and X. Chen, Headspace solid-phase microextraction coupled to gas chromatography for the analysis of aldehydes in edible oils, Talanta, 2014, 120, 94–99, DOI:10.1016/j.talanta.2013.11.021.
- X. Q. Wang, L. Pan, J. J. Feng, Y. Tian, C. N. Luo and M. Sun, Silk fiber for in-tube solid-phase microextraction to detect aldehydes by chemical derivatization, J. Chromatogr. A, 2017, 1522, 16–22, DOI:10.1016/j.chroma.2017.09.058.
- J. M. Fernandez-Molina and M. Silva, Micro solid-phase derivatization analysis of low molecular mass aldehydes in treated water by micellar electrokinetic chromatography, Electrophoresis, 2014, 35, 819–826, DOI:10.1002/elps.201300433.
- C. Basheer, S. Pavagadhi, H. Yu, R. Balasubramanian and H. K. Lee, Determination of aldehydes in rainwater using micro-solid-phase extraction and high-performance liquid chromatography, J. Chromatogr. A, 2010, 1217, 6366–6372, DOI:10.1016/j.chroma.2010.08.012.
- L. Xia, Y. Q. Du, X. H. Xiao and G. K. Li, One-step membrane protected micro-solid-phase extraction and derivatization coupling to high-performance liquid chromatography for selective determination of aliphatic aldehydes in cosmetics and food, Talanta, 2019, 202, 580–590, DOI:10.1016/j.talanta.2019.05.035.
- A. Safavi, R. Ahmadi and A. M. Ramezani, Vortex-assisted liquid-liquid microextraction based on hydrophobic deep eutectic solvent for determination of malondialdehyde and formaldehyde by HPLC-UV approach, Microchem. J., 2018, 143, 166–174, DOI:10.1016/j.microc.2018.07.036.
- Y. Li, F. Yi, Y. L. Zheng, Y. Wang and J. N. Ye, Hollow-fiber liquid-phase microextraction coupled with miniature capillary electrophoresis for the trace analysis of four aliphatic aldehydes in water samples, J. Sep. Sci., 2015, 38, 2873–2879, DOI:10.1002/jssc.201500323.
- J. Huang, H. T. Deng, D. D. Song and H. Xu, Electrospun polystyrene/graphene nanofiber film as a novel adsorbent of thin-film microextraction for extraction of aldehydes in human exhaled breath condensates, Anal. Chim. Acta, 2015, 878, 102–108, DOI:10.1016/j.aca.2015.03.053.
- A. Zamora-Gálvez, A. Ait-Lahcen, L. A. Mercante, E. Morales-Narváez, A. Amine and A. Merkoci, Molecularly imprinted polymer-decorated magnetite nanoparticles for selective sulfonamide detection, Anal. Chem., 2016, 88, 3578–3584, DOI:10.1021/acs.analchem.5b04092.
- S. Y. Fang, Y. Y. Liu, J. H. He, L. Q. Zhang, Z. X. Liyin, X. Y. Wu, H. Sun and J. P. Lai, Determination of aldehydes in water samples by coupling magnetism-reinforced molecular imprinting monolith microextraction and non-aqueous capillary electrophoresis, J. Chromatogr. A, 2020, 1632, 461602, DOI:10.1016/j.chroma.2020.461602.
- Y. H. He, S. J. Tan, A. M. Abd El-Aty, A. Hacimuftuoglu and Y. X. She, Magnetic molecularly imprinted polymers for the detection of aminopyralid in milk using dispersive solid-phase extraction, RSC Adv., 2019, 9, 29998–30006, 10.1039/c9ra05782j.
- P. A. G. Cormack and K. Mosbach, Molecular imprinting: recent developments and the road ahead, React. Funct. Polym., 1999, 41, 115–124, DOI:10.1016/S1381-5148(99)00024-3.
- B. Buszewski and E. Klodzinska, Rapid microbiological diagnostics in medicine using electromigration techniques, TrAC, Trends Anal. Chem., 2006, 78, 95–108, DOI:10.1016/j.trac.2016.02.008.
- A. Roychoudhury, K. A. Francis, J. Patel, S. K. Jha and S. Basu, A decoupler-free simple paper microchip capillary electrophoresis device for simultaneous detection of dopamine, epinephrine and serotonin, RSC Adv., 2020, 10, 25487–25495, 10.1039/d0ra03526b.
- C. A. Groom, S. Beaudet, A. Halasz, L. Paquet and J. Hawari, Application of sodium dodecyl sulfate micellar electrokinetic chromatography (SDS MEKC) for the rapid measurement of aqueous phase 2,4,6-trinitrotoluene metabolites in anaerobic sludge: A comparison with LC/MS, Environ. Sci. Technol., 2000, 34, 2330–2336, DOI:10.1021/es991233x.
- I. Ali, Z. A. Al-Othman, A. Al-Warthan, L. Asnin and A. Chudinov, Advances in chiral separations of small peptides by capillary electrophoresis and chromatography, J. Sep. Sci., 2014, 18, 2447–2466, DOI:10.1002/jssc.201400587.
- Z. A. Al-Othman, A. Al-Warthan, S. D. Alam and I. Ali, Enantio-separation
of drugs with multiple chiral centers by chromatography and capillary electrophoresis, Biomed. Chromatogr., 2015, 28, 1514–1524, DOI:10.1002/bmc.3259.
- I. Ali, M. Suhail, Z. A. Al-Othman, A. Alwarthan and Y. Aboul-Enein, Enantiomeric resolution of multiple chiral centres racemates by capillary electrophoresis, Biomed. Chromatogr., 2016, 30, 683–694, DOI:10.1002/bmc.3691.
- I. Ali, V. K. Gupta and H. Y. Aboul-Enein, Metal ion speciation and capillary electrophoresis: Application in the new millennium, Electrophoresis, 2005, 26, 3988–4002, DOI:10.1002/elps.200500216.
- I. Ali, A. Haque, W. A. Wani, K. Saleem and M. A. Zaabi, Analyses of anticancer drugs by capillary electrophoresis: a review, Biomed. Chromatogr., 2013, 27, 1296–1311, DOI:10.1002/bmc.2953.
- Y. L. Li, H. Sun, J. P. Lai, X. Y. Chang, P. Zhang and S. L. Chen, Determination of carbonyl pollutants adsorbed on ambient particulate matter of type PM 2.5 by using magnetic molecularly imprinted microspheres for sample pretreatment and capillary electrophoresis for separation and quantitation, Microchim. Acta, 2018, 185, 122, DOI:10.1007/s00604-017-2650-0.
- D. Burns, Guidelines for drinking-water quality, 2011, 4th edn, https://scholar.google.com/scholar_lookup?title=Guidelines%20for%20Drinking-water%20Quality%26author=WHO%26publication_year=2011 Search PubMed.
- E. Commission, Commission Regulation (EU) No 10/2011/EC of 14 January 2011 on plastic materials and articles intended to come into contact with food, Off. J. Eur. Communities, 2011, 12, 1–138 Search PubMed , https://www.researchgate.net/publication/304749604_Commission_Regulation_EU_No_102011EC_of_14_January_2011_on_plastic_materials_and_articles_intended_to_come_into_contact_with_food.
- H. Yamada and I. Somiya, Identification of products resulting from ozonation of organic compounds in water, Ozone: Sci. Eng., 1980, 2, 251–260, DOI:10.1080/01919518008550887.
- M. Abboudi and A. Odeh, Impact of sunlight/dark storage on natural spring water bottled in polyethylene terephthalate, J. Water Supply: Res. Technol.–AQUA, 2015, 64, 149–156, DOI:10.2166/aqua.2014.076.
- J. Nawrocki, A. Dabrowska and A. Borcz, Investigation of carbonyl compounds in bottled waters from Poland, Water Res., 2002, 36, 4893–4901, DOI:10.1016/S0043-1354(02)00201-4.
- B. Nijssen, T. K. And and J. Jetten, Acetaldehyde in mineral water stored in polyethylene terephtalate (PET) bottles: odour threshold and quantification, Packag. Technol. Sci., 1996, 9, 175–185, DOI:10.1002/(SICI)1099-1522(199607)9.
- M. Mutsuga, Y. Kawamura, Y. Sugita-Konishi, Y. Hara-Kudo, K. Takatori and K. Tanamoto, Migration of formaldehyde and acetaldehyde into mineral water in polyethylene terephthalate (PET) bottles, Food Addit. Contam., 2006, 23, 212–218, DOI:10.1080/02652030500398361.
- C. Bach, X. Dauchy, M. C. Chagnon and S. Etienne, Chemical compounds and toxicological assessments of drinking water stored in polyethylene terephthalate (PET) bottles: a source of controversy reviewed, Water Res., 2012, 46, 571–583, DOI:10.1016/j.watres.2011.11.062.
- E. M. Bauske, P. A. Backman, K. M. Harper, P. M. Brannen, R. Rodriguez-Kabana and J. W. Kloepper, Effect of Botanical Aromatic Compounds and Seed-surface pH on Growth and Colonization of Cotton Plant Growth-promoting Rhizobacteria, Biocontrol Science and Technology, 1997, 7, 415–422, DOI:10.1080/09583159730820.
- S. Sukharev, R. Mariychuk, M. Onysko, O. Sukhareva and S. Delegan-Kokaiko, Fast determination of total aldehydes in rainwaters in the presence of interfering compounds, Environ. Chem. Lett., 2019, 17, 1405–1411, DOI:10.1007/s10311-019-00875-z.
- C. F. Nascimento, M. A. S. Brasil, S. P. F. Cost, P. C. A. G. Pinto, M. L. M. F. S. Saraiva and F. R. P. Rocha, Exploitation of pulsed flows for on-line dispersive liquid-liquid microextraction: Spectrophotometric determination of formaldehyde in milk, Talanta, 2015, 144, 1189–1194, DOI:10.1016/j.talanta.2015.07.076.
- W. K. Lia, Y. Z. Ding, J. T. Feng and Z. Q. Ma, A novel luminescent dual-ligands europium(III) complex prepared for acetaldehyde sensitive detection, Sens. Actuators, B, 2020, 306, 127542, DOI:10.1016/j.snb.2019.127542.
- H. J. Kim and H. S. Shin, Simple and automatic determination of aldehydes and acetone in water by headspace solid-phase microextraction and gas chromatography-mass spectrometry, J. Sep. Sci., 2011, 34, 693–699, DOI:10.1002/jssc.201000679.
- H. J. Zhang, J. F. Huang, H. Wang and Y. Q. Feng, Determination of low-aliphatic aldehyde derivatizatives in human saliva using polymer monolith microextraction coupled to high-performance liquid chromatography, Anal. Chim. Acta, 2006, 565, 129, DOI:10.1016/j.aca.2006.02.050.
Footnotes |
† Electronic supplementary information (ESI) available. See DOI: 10.1039/d1ra05084b |
‡ Jiahua He and Jiawei Liu contributed equally to this work. |
|
This journal is © The Royal Society of Chemistry 2021 |
Click here to see how this site uses Cookies. View our privacy policy here.