DOI:
10.1039/D1SC03023J
(Edge Article)
Chem. Sci., 2021,
12, 12911-12917
Biphasic electrochemical peptide synthesis†
Received
4th June 2021
, Accepted 1st September 2021
First published on 2nd September 2021
Introduction
Recently, peptides have been recognized as candidates for “medium” molecular medicines,1a which refers to pharmaceutical compounds whose molecular weights are roughly in the 1000 to 5000 range. This class of medicines has more specificity and fewer side effects than conventional small molecular medicines.1b In 2018, over 60 peptides were approved as drugs in the United States, Europe and Japan. In addition, over 150 peptides are in active clinical development, and more than 260 have been tested in human clinical trials.2 Even as peptides are gaining such a great deal of attention, the long-standing problem of the large amount of waste produced has not been resolved.1 One contributor to waste generation is the use of coupling reagents that enable efficient peptide bond formation.3 Generally, peptides are synthesized through repetitive deprotection and peptide bond formation reactions. To form peptide bonds between carboxylic acids and amines, stoichiometric amounts of coupling reagents are required (Scheme 1a), which generate stoichiometric byproducts, leading to accumulation of chemical waste.4 In this regard, the development of greener peptide synthetic processes is identified as a key for green chemistry.1a,e,g
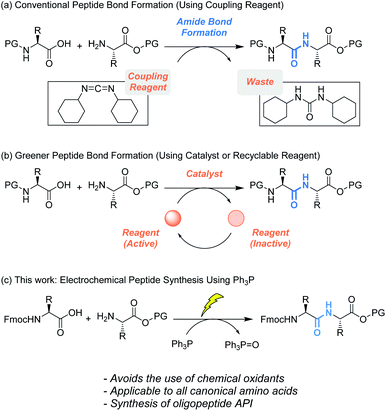 |
| Scheme 1 (a) Conventional and (b) greener peptide bond formation. (c) Electrochemical peptide bond formation. | |
To address this issue, catalytic peptide synthesis has emerged (Scheme 1b, above arrow). Since Yamamoto reported boronic acid catalyzed amide bond formation,5a various organoboron catalysts have been developed,5b–g some of which have proven to be effective for oligopeptide synthesis. The mechanism of the reaction has been well studied, which has aided researchers in designing more sophisticated catalysts. In addition, recent studies revealed that several metals act as Lewis acid catalysts. These catalysts form active complexes with in situ generated esters, and promote peptide bond formation while avoiding epimerization.5h,i
We envisioned that development of an efficient peptide synthetic method using potentially recyclable reagents would pave the way to an alternative solution (Scheme 1b, below arrow). For this strategy, the use of phosphine (R3P) would be a promising approach. Oxidative activation of R3P generates an electrophilic phosphine cation, which serves as the coupling reagent to activate carboxylic acids and facilitate amide bond formation.6 In 2019, the Arora group reported efficient oligopeptide synthesis using Bu3P activated by a diselenide catalyst. Although phosphine oxide (R3P
O) is produced as a stoichiometric byproduct, its reduction back to R3P has been achieved in various ways.7 Therefore, R3P
O can be a recyclable byproduct, unlike byproducts from typical peptide coupling reagents. Among R3P, triphenylphosphine (Ph3P) would be most suitable because Ph3P is easy to handle and reduction of triphenylphosphine oxide (Ph3P
O) to Ph3P proceeds more efficiently than the case for alkyl phosphines. Moreover, Sevov reported an electrochemical reduction method applicable on a large scale,7k and Favre-Réguillon has also succeeded in the conversion of Ph3P
O to Ph3P on a 100 g scale by the combination of Ti(OiPr)4 and hydrosiloxane.7i Therefore, development of peptide synthesis utilizing Ph3P and recovery of Ph3P
O would demonstrate the potential of Ph3P as a recyclable coupling reagent.
In this context, we aimed to develop a peptide synthesis method using Ph3P by performing electrochemical amide bond formation as reported by Frontana-Uribe.8 In this electrochemical method, because electrons themselves act as an oxidant to generate a phosphine cation to facilitate amide bond formation, waste from chemical oxidants can be avoided.9 To facilitate recovery of Ph3P
O, soluble tags as the carboxylic acid protecting groups could be combined with the electrochemical method.10 Soluble tags are benzyl alcohols bearing long alkyl chains, so peptides protected with soluble tags dissolve in THF, CH2Cl2 and c-Hex, but precipitate in polar solvents like MeCN. Hence, peptide synthesis is conducted in the liquid phase, and purification can be accomplished simply by filtration, which realizes facile separation of peptides and Ph3P
O. In this paper, we describe biphasic electrochemical peptide bond formation, leading to the synthesis of a commercial peptide active pharmaceutical ingredient (API), leuprorelin, without the use of traditional coupling reagents and recovery of Ph3P
O from the reaction mixture (Fig. 1).
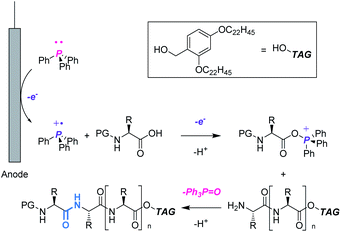 |
| Fig. 1 Electrochemical peptide synthesis utilizing soluble tag-assisted method. | |
Results and discussion
First, we optimized the reaction between Fmoc-Asp(OtBu)-OH (1a) and H-Asp(OtBu)-OTAG (2a) as models using Ph3P (Table 1). In CH2Cl2, the reaction proceeded efficiently to give the dipeptide (3aa) in 92% yield (entry 1). On the other hand, the yield was significantly decreased to 19% in THF and most starting materials were recovered even when 4.8 F mol−1 was passed (entry 2). Since THF is more coordinating than CH2Cl2, it is likely that THF coordinates to Ph3P˙+ to lower its electrophilicity, which leads to low efficiency of carboxylic acid activation.11 A biphasic condition (MeCN/c-Hex) was also found to be effective,12 affording 3aa in excellent yield (entry 3, Fig. S1 and Scheme S1†). Hydrophobic 2a is localized in the upper c-Hex, while polar 1a and other reagents are selectively dissolved in the lower MeCN, where the electrochemical reaction takes place. The peptide bond formation between the activated 1a and 2a is expected to occur at the surface of the reversed micelle. Since 3aa is also localized in c-Hex, it is noteworthy that its separation from the supporting electrolyte is possible by simple phase separation. Furthermore, in the biphasic condition, since c-Hex is not conductive, undesired oxidation of 2a and/or overoxidation of 3aa could be suppressed. Avoiding the use of hazardous CH2Cl2 is preferred from the green chemistry viewpoint, and therefore, further investigations were carried out using the biphasic condition.
Table 1 Optimization and comparison studies for electrochemical peptide bond formationa
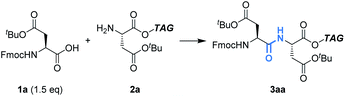
|
Entryb |
Electrolyte solution |
Yieldc (%) |
Conditions: Ph3P (2.0 eq.), 2,6-lutidine (3.0 eq.), supporting electrolyte (0.05 M), platinum electrodes, 2.0 mA, 4.8 F mol−1, rt, undivided cell.
Carried out at 0.20 mmol scale (2a).
Determined by NMR analysis.
|
1 |
Bu4NClO4/CH2Cl2 |
92 |
2 |
Bu4NClO4/THF |
19 |
3 |
Bu4NClO4/c-Hex/MeCN |
95 |
4 |
LiClO4/c-Hex/MeCN |
86 |
5 |
NaClO4/c-Hex/MeCN |
— |
6 |
KClO4/c-Hex/MeCN |
55 |
When LiClO4 or KClO4 was used instead of Bu4NClO4, the yields decreased somewhat (entries 4 and 6), while the use of NaClO4 caused severe gelation of the reaction mixture (entry 5). These observations suggest that tetrabutylammonium ions are more suitable for the reaction than alkali metal cations, presumably due to weaker interactions with the carboxylate anion of 1a. Moreover, 31P NMR indicated that the transformation of Ph3P into Ph3P
O was selective. After further investigation of the procedure, we succeeded in the recovery of both Bu4NClO4 (>99%) and Ph3P
O (91%) with high purity (>95%) without column purification (detailed procedure and purity assessment are in ESI†).
Having confirmed the potential of Ph3P as a recyclable coupling reagent, we explored the feasibility of electrochemical peptide bond formation with other Fmoc-protected amino acids (1b–1u) instead of 1a, using 2a as a reaction partner (Table 2). Although heating was needed in some cases to avoid gelation of the reaction mixture, all the Fmoc-protected canonical amino acids were demonstrated to be amenable to the reaction. It should be noted that not only amino acids with redox inactive alkyl side chains but also those with redox active moieties, such as Cys and Met, gave the desired products in excellent yields. When Fmoc-His(Trt)-OH was used (3pa), however, partial epimerization occurred, likely due to the basicity of the imidazole moiety.13 Boc is an electron-withdrawing alternative to Trt for the protection of imidazole, which may decrease its basicity. Using Boc, we succeeded in preventing the epimerization and the desired product (3qa) was obtained in excellent yield as a pure stereoisomer. Slightly excessive amounts of reagents and electricity were needed for the reaction of Fmoc-Pro-OH (3ua), presumably due to steric hindrance.
Table 2 Scope of Fmoc-protected amino acids
We next turned our attention to explore the compatibility of this method with other tag-protected amino acids (2b–2u) instead of 2a, using 1a as a reaction partner (Table 3). Although heating was also needed in some cases to avoid gelation of the reaction mixture, all the tag-protected canonical amino acids were demonstrated to be amenable to the reaction. In this case, no significant epimerization was observed for the reaction of H-His(Trt)-OTAG, and the desired product (3ap) was obtained in excellent yield as a pure stereoisomer. The reaction of H-Pro-OTAG (3au) was less efficient than others, requiring slightly higher amounts of reagents and electricity. It should be noted that the protecting groups commonly employed in conventional peptide synthesis were proven to be compatible with the reaction, and therefore, commercially available building blocks could be used directly in this method.
Table 3 Scope of tag-protected amino acids
Having confirmed that electrochemical peptide bond formation can be applied to all canonical amino acids, we applied this method to the synthesis of a commercial peptide active pharmaceutical ingredient (API), Leuprorelin, as a model (Scheme 2A, see ESI† for experimental details).14 Starting from Fmoc-Pro-NEtTAG (4),10d all 8 peptide bonds were electrochemically formed. Deprotections of Fmoc were carried out under typical conditions using DBU and piperidine. After repetitive deprotections and couplings, the protected form of leuprorelin (5) was successfully obtained in 45% yield over 16 steps (56% by weight, and its purity was estimated to be 81% by HPLC, Scheme 2B and Fig. S2†). The average yield in each step was >95%. Finally, acidic global deprotection, which is commonly used in conventional peptide synthesis, afforded leuprorelin (6) quantitatively (Fig. S3†). This result demonstrates that electrochemical peptide synthesis has the potential to be an alternative choice for conventional SPPS and/or LPPS.
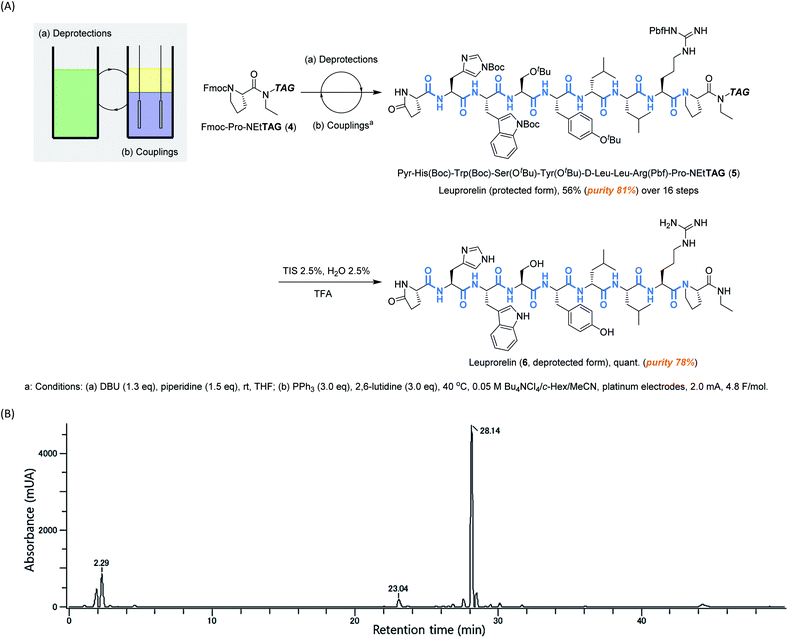 |
| Scheme 2 (A) Biphasic electrochemical synthesis of leuprorelin, and (B) HPLC analysis of leuprorelin (protected form, R. T. = 28.14) (5). | |
Conclusions
We succeeded in developing a biphasic electrochemical peptide bond formation reaction using Ph3P. Electrochemically generated Ph3P˙+ promoted efficient peptide bond formation with no significant epimerization. Protecting groups commonly employed in conventional peptide synthesis were proven to be compatible with the reaction, and therefore, commercially available building blocks could be used directly in this method. In combination with a soluble tag-assisted LPPS, the synthesis of a commercial peptide API, leuprorelin, was achieved without the use of traditional coupling reagents. Although using Ph3P as a coupling reagent under electrochemical conditions results in the generation of Ph3P
O, the biphasic system enabled selective recovery of the desired peptides and Ph3P
O by simple phase separation. Given that methods to reduce Ph3P
O to Ph3P have been reported, Ph3P is a potentially recyclable coupling reagent. Therefore, this soluble tag-assisted electrochemical method can be used to address sustainability challenges in peptide synthesis. Aiming for further improvement, we are exploring novel methods to reduce Ph3P
O to Ph3P.
Data availability
The datasets supporting this article have been uploaded as part of the ESI.†
Author contributions
K. C. conceived and directed the project. S. N. designed and performed the experiments. S. N., Y. O., Y. K., and K. C. discussed results. S. N. and Y. O. wrote the manuscript.
Conflicts of interest
There are no conflicts to declare.
Acknowledgements
This work was supported in part by JSPS KAKENHI Grant No. 17K19222, 19H00930, 19K22272 (to K. C.), 16H06193, 17K19221 (to Y. O.), 21J11675 (to S. N.), and JST CREST JPMJCR19R2 (to K. C.).
Notes and references
-
(a) M. C. Bryan, P. J. Dunn, D. Entwistle, F. Gallou, S. G. Koenig, J. D. Hayler, M. R. Hickey, S. Hughes, M. E. Kopach, G. Moine, P. Richardson, F. Roschangar, A. Steven and F. J. Weiberth, Green Chem., 2018, 20, 5082–5103 RSC;
(b) V. R. Pattabiraman and J. W. Bode, Nature, 2011, 480, 471–479 CrossRef CAS PubMed;
(c) R. M. de Figueiredo, J. S. Suppo and J. M. Campagne, Chem. Rev., 2016, 116, 12029–12122 CrossRef CAS PubMed;
(d) X. Wang, Nat. Catal., 2019, 2, 98–102 CrossRef;
(e) M. T. Sabatini, L. T. Boulton, H. F. Sneddon and T. D. Sheppard, Nat. Catal., 2019, 2, 10–17 CrossRef CAS;
(f) A. S. Santos, A. M. S. Silva and M. M. B. Marques, Eur. J. Org. Chem., 2020, 2501–2516 CrossRef CAS;
(g) A. Isidro-Llobet, M. N. Kenworthy, S. Mukherjee, M. E. Kopach, K. Wegner, F. Gallou, A. G. Smith and F. Roschangar, J. Org. Chem., 2019, 84, 4615–4628 CrossRef CAS PubMed.
- J. L. Lau and M. K. Dunn, Bioorg. Med. Chem., 2018, 26, 2700–2707 CrossRef CAS PubMed.
- A. El-Faham and F. Albericio, Chem. Rev., 2011, 111, 6557–6602 CrossRef CAS PubMed.
-
(a) B. M. Trost, Science, 1991, 254, 1471–1477 CrossRef CAS PubMed;
(b) R. A. Sheldon, Green Chem., 2007, 9, 1273–1283 RSC.
-
(a) K. Ishihara, S. Ohara and H. Yamamoto, J. Org. Chem., 1996, 61, 4196–4197 CrossRef CAS PubMed;
(b) R. M. Al-Zoubi, O. Marion and D. G. Hall, Angew. Chem., Int. Ed., 2008, 47, 2876–2879 CrossRef CAS PubMed;
(c) M. T. Sabatini, L. T. Boulton and T. D. Sheppard, Sci. Adv., 2017, 3, e1701028 CrossRef PubMed;
(d) K. Wang, Y. Lu and K. Ishihara, Chem. Commun., 2018, 54, 5410–5413 RSC;
(e) H. Noda, M. Furutachi, Y. Asada, M. Shibasaki and N. Kumagai, Nat. Chem., 2017, 9, 571–577 CrossRef CAS PubMed;
(f) Z. Liu, H. Noda, M. Shibasaki and N. Kumagai, Org. Lett., 2018, 20, 612–615 CrossRef CAS PubMed;
(g) K. Michigami, T. Sakaguchi and Y. Takemoto, ACS Catal., 2020, 10, 683–688 CrossRef CAS;
(h) W. Muramatsu, T. Hattori and H. Yamamoto, J. Am. Chem. Soc., 2019, 141, 12288–12295 CrossRef CAS PubMed;
(i) W. Muramatsu and H. Yamamoto, J. Am. Chem. Soc., 2019, 141, 18926–18931 CrossRef CAS PubMed.
-
(a) L. E. Barstow and V. J. Hruby, J. Org. Chem., 1971, 36, 1305–1306 CrossRef CAS;
(b) D. C. Lenstra, F. P. J. T. Rutjes and J. Mecinovic, Chem. Commun., 2014, 50, 5763–5766 RSC;
(c) C. Zhang, S. Liu, B. Sun and J. Tian, Org. Lett., 2015, 17, 4106–4109 CrossRef CAS PubMed;
(d) W. Phakhodee, S. Wangngae and M. Pattarawarapan, RSC Adv., 2016, 6, 60287–60290 RSC;
(e) M. Zhang, X. A. Yuan, C. Zhu and J. Xie, Angew. Chem., Int. Ed., 2019, 58, 312–316 CrossRef CAS PubMed;
(f) Handoko, S. Satishkumar, N. R. Panigrahi and P. S. Arora, J. Am. Chem. Soc., 2019, 141, 15977–15985 CrossRef CAS PubMed.
-
(a) D. Hérault, D. H. Nguyen, D. Nuel and G. Buono, Chem. Soc. Rev., 2015, 44, 2508–2528 RSC;
(b) H. Fritzsche, U. Hasserodt and F. Korte, Chem. Ber., 1965, 98, 171–174 CrossRef CAS;
(c) K. Naumann, G. Zon and K. Mislow, J. Am. Chem. Soc., 1969, 91, 7012–7023 CrossRef CAS;
(d) A. Gevorgyan, S. Mkrtchyan, T. Grigoryan and V. O. Iaroshenko, Org. Chem. Front., 2017, 4, 2437–2444 RSC;
(e) M. Schirmer, S. Jopp, J. Holz, A. Spannenberg and T. Werner, Adv. Synth. Catal., 2016, 358, 26–29 CrossRef CAS;
(f) Y. Li, S. Das, S. Zhou, K. Junge and M. Beller, J. Am. Chem. Soc., 2012, 134, 9727–9732 CrossRef CAS PubMed;
(g) H. Fritzsche, U. Hasserodt and F. Korte, Chem. Ber., 1964, 97, 1988–1993 CrossRef CAS;
(h) H. Kawakubo, M. Kuroboshi, T. Yano, K. Kobayashi, S. Kamenoue, T. Akagi and H. Tanaka, Synthesis, 2011, 24, 4091–4098 Search PubMed;
(i) C. Petit, E. Poli, A. Favre-Réguillon, L. Khrouz, S. Denis-Quanquin, L. Bonneviot, G. Mignani and M. Lemaire, ACS Catal., 2013, 3, 1431–1438 CrossRef CAS;
(j) J. S. Elias, C. Costentin and D. G. Nocera, J. Am. Chem. Soc., 2018, 140, 13711–13718 CrossRef CAS PubMed;
(k) S. Manabe, C. M. Wong and C. S. Sevov, J. Am. Chem. Soc., 2020, 142, 3024–3031 CrossRef CAS PubMed;
(l) Ł. Kapuśniak, P. N. Plessow, D. Trzybiński, K. Woźniak, P. Hofmann and P. I. Jolly, Organometallics, 2021, 40, 693–701 CrossRef PubMed.
- A. Palma, J. Cardenas and B. A. Frontana-Uribe, Green Chem., 2009, 11, 283–293 RSC.
- For recent reviews, see:
(a) M. D. Kärkäs, Chem. Soc. Rev., 2018, 47, 5786–5865 RSC;
(b) S. Möhle, M. Zirbes, E. Rodrigo, T. Gieshoff, A. Wiebe and S. R. Waldvogel, Angew. Chem., Int. Ed., 2018, 57, 6018–6041 CrossRef PubMed;
(c) A. Wiebe, T. Gieshoff, S. Möhle, E. Rodrigo, M. Zirbes and S. R. Waldvogel, Angew. Chem., Int. Ed., 2018, 57, 5594–5619 CrossRef CAS PubMed;
(d) S. R. Waldvogel, S. Lips, M. Selt, B. Riehl and C. J. Kampf, Chem. Rev., 2018, 118, 6706–6765 CrossRef CAS PubMed;
(e) K. D. Moeller, Chem. Rev., 2018, 118, 4817–4833 CrossRef CAS PubMed;
(f) J. G. Ibanez, M. E. Rincón, S. Gutierrez-Granados, M. Chahma, O. A. Jaramillo-Quintero and B. A. Frontana-Uribe, Chem. Rev., 2018, 118, 4731–4816 CrossRef CAS PubMed;
(g) J. Yoshida, A. Shimizu and R. Hayashi, Chem. Rev., 2018, 118, 4702–4730 CrossRef CAS PubMed;
(h) Y. Okada and K. Chiba, Chem. Rev., 2018, 118, 4592–4630 CrossRef CAS PubMed;
(i) Y. Jiang, K. Xu and C. Zeng, Chem. Rev., 2018, 118, 4485–4540 CrossRef CAS PubMed;
(j) M. Yan, Y. Kawamata and P. S. Baran, Chem. Rev., 2017, 117, 13230–13319 CrossRef CAS PubMed.
-
(a) Y. Okada, R. Takasawa, D. Kubo, N. Iwanaga, S. Fujita, K. Suzuki, H. Suzuki, H. Kamiya and K. Chiba, Org. Process Res. Dev., 2019, 23, 2576–2581 CrossRef CAS;
(b) H. Wakamatsu, Y. Okada, M. Sugai, S. R. Hussaini and K. Chiba, Asian J. Org. Chem., 2017, 6, 1584–1588 CrossRef CAS;
(c) Y. Okada, H. Wakamatsu, M. Sugai, E. I. Kauppinen and K. Chiba, Org. Lett., 2015, 17, 4264–4267 CrossRef CAS PubMed;
(d) E. Matsumoto, Y. Fujita, Y. Okada, E. I. Kauppinen, H. Kamiya and K. Chiba, J. Pept. Sci., 2015, 21, 691–695 CrossRef CAS PubMed;
(e) Y. Okada, S. Hosoya, H. Suzuki and K. Chiba, Org. Lett., 2014, 16, 6448–6451 CrossRef CAS PubMed;
(f) Y. Okada, H. Suzuki, T. Nakae, S. Fujita, H. Abe, K. Nagano, T. Yamada, N. Ebata, S. Kim and K. Chiba, J. Org. Chem., 2013, 78, 320–327 CrossRef CAS PubMed;
(g) Y. Fujita, S. Fujita, Y. Okada and K. Chiba, Org. Lett., 2013, 15, 1155–1157 CrossRef CAS PubMed;
(h) S. Kitada, M. Takahashi, Y. Yamaguchi, Y. Okada and K. Chiba, Org. Lett., 2012, 14, 5960–5963 CrossRef CAS PubMed;
(i) G. Tana, S. Kitada, S. Fujita, Y. Okada, S. Kim and K. Chiba, Chem. Commun., 2010, 46, 8219–8221 RSC.
-
(a) N. Shida, Y. Imada, S. Nagahara, Y. Okada and K. Chiba, Commun. Chem., 2019, 2, 24 CrossRef;
(b) F. Cataldo, Eur. Chem. Bull., 2015, 4, 92–97 Search PubMed.
-
(a) Y. Okada, K. Kamimura and K. Chiba, Tetrahedron, 2012, 68, 5857–5862 CrossRef CAS;
(b) Y. Okada, Y. Yamaguchi and K. Chiba, Eur. J. Org. Chem., 2012, 243–246 CrossRef CAS;
(c) Y. Okada, T. Yoshioka, M. Koike and K. Chiba, Tetrahedron Lett., 2011, 52, 4690–4693 CrossRef CAS;
(d) Y. Okada and K. Chiba, Electrochim. Acta, 2010, 55, 4112–4119 CrossRef CAS;
(e) S. Kim, S. Noda, K. Hayashi and K. Chiba, Org. Lett., 2008, 10, 1827–1830 CrossRef CAS PubMed;
(f) K. Chiba, Y. Kono, S. Kim, K. Nishimoto, Y. Kitano and M. Tada, Chem. Commun., 2002, 1766–1767 RSC.
- J. H. Jones, W. I. Ramage and M. J. Witty, Int. J. Pept. Protein Res., 1980, 15, 301–303 CrossRef CAS PubMed.
- M. Fujino, T. Fukuda, S. Shinagawa, S. Kobayashi, I. Yamazaki and R. Nakayama, Biochem. Biophys. Res. Commun., 1974, 60, 406–413 CrossRef CAS PubMed.
Footnote |
† Electronic supplementary information (ESI) available. See DOI: 10.1039/d1sc03023j |
|
This journal is © The Royal Society of Chemistry 2021 |
Click here to see how this site uses Cookies. View our privacy policy here.