DOI:
10.1039/D1SC05007A
(Edge Article)
Chem. Sci., 2021,
12, 15935-15946
Going beyond the borders: pyrrolo[3,2-b]pyrroles with deep red emission†
Received
9th September 2021
, Accepted 19th November 2021
First published on 22nd November 2021
Abstract
A two-step route to strongly absorbing and efficiently orange to deep red fluorescent, doubly B/N-doped, ladder-type pyrrolo[3,2-b]pyrroles has been developed. We synthesize and study a series of derivatives of these four-coordinate boron-containing, nominally quadrupolar materials, which mostly exhibit one-photon absorption in the 500–600 nm range with the peak molar extinction coefficients reaching 150
000, and emission in the 520–670 nm range with the fluorescence quantum yields reaching 0.90. Within the family of these ultrastable dyes even small structural changes lead to significant variations of the photophysical properties, in some cases attributed to reversal of energy ordering of alternate-parity excited electronic states. Effective preservation of ground-state inversion symmetry was evidenced by very weak two-photon absorption (2PA) at excitation wavelengths corresponding to the lowest-energy, strongly one-photon allowed purely electronic transition. π-Expanded derivatives and those possessing electron-donating groups showed the most red-shifted absorption- and emission spectra, while displaying remarkably high peak 2PA cross-section (σ2PA) values reaching ∼2400 GM at around 760 nm, corresponding to a two-photon allowed higher-energy excited state. At the same time, derivatives lacking π-expansion were found to have a relatively weak 2PA peak centered at ca. 800–900 nm with the maximum σ2PA ∼50–250 GM. Our findings are augmented by theoretical calculations performed using TD-DFT method, which reproduce the main experimental trends, including the 2PA, in a nearly quantitative manner. Electrochemical studies revealed that the HOMO of the new dyes is located at ca. −5.35 eV making them relatively electron rich in spite of the presence of two B−–N+ dative bonds. These dyes undergo a fully reversible first oxidation, located on the diphenylpyrrolo[3,2-b]pyrrole core, directly to the di(radical cation) stage.
Introduction
In recent years, boron has found new distinct roles in materials science, being incorporated in various polycyclic aromatic hydrocarbons (PAHs).1–7 It has been discovered that doping with three-coordinate, sp2-hybridized boron has the most pronounced effect on aromatic systems among main group elements, due to its low Pauling electronegativity and enhanced π-conjugation resulting from its vacant pz orbital. As a consequence, in such systems boron atoms can act simultaneously as π-electron acceptors and σ-electron donors. The fascinating properties of the materials obtained in such a way (so-called B-PAHs), such as strong fluorescence8–11 and enhanced charge-transport characteristics,12,13 has resulted in a wide range of applications in optoelectronics, including materials for organic light-emitting diodes (OLEDs)14–16 circularly polarized luminescence,17 organic photovoltaics (OPVs)18 and organic field-effect transistors (OFETs)19–21 as well as materials for electrodes in lithium batteries.22 Much attention has also been paid to the B–N/O isosteres of PAHs, i.e. compounds in which two adjacent carbons in a π-conjugated core have been replaced by one boron and one N/O atom.23–28 Of particular importance are π-conjugated systems containing a B–N covalent bond, B←N coordination bond29 and N–B←N motif, as evidenced by growing number of reports dealing with BN-embedded heteroacenes29–32 and BODIPY analogues,33–37 mostly due to their excellent performance in OLEDs38a and OPVs.38b,c They were also computed to possess inverted singlet-triplet gap.39 In such systems, the N–B–N motif not only constrains the π-conjugated skeleton in a coplanar fashion, but also considerably lowers the LUMO level, making the core of the dye a strong acceptor.
1,2,4,5-Tetraarylpyrrolo[3,2-b]pyrroles (TAPPs)40 are aza-analogues of well-known thieno[3,2-b]thiophenes.41 Recent synthetic breakthroughs,42 prompted their application in research related to studying symmetry breaking in the excited state,43 solvatofluorochromism,43b,c direct solvent probing via H-bonding interactions44 photochromic analysis of halocarbons45 organic light emitting diodes46 resistive memory devices,47 bulk heterojunction organic solar cells,48 dye-sensitized solar cells,49 aggregation-induced emission50 and MOFs.51 Furthermore, the very high reactivity at positions 3 and 6 of the pyrrolo[3,2-b]pyrrole core makes them very convenient starting materials for the construction of ladder type heterocycles. Taking advantage of this feature, many reports have been published on the synthesis and properties of TAPP-based PAHs,52 however, there has only been one successful synthesis of BN-embedded TAPPs (Fig. 1).53 The resulting dyes, containing boron atoms incorporated into six-membered cycles, exhibited very high absorption coefficients and strong fluorescence, both in solution and the solid state, with very small Stokes shifts.
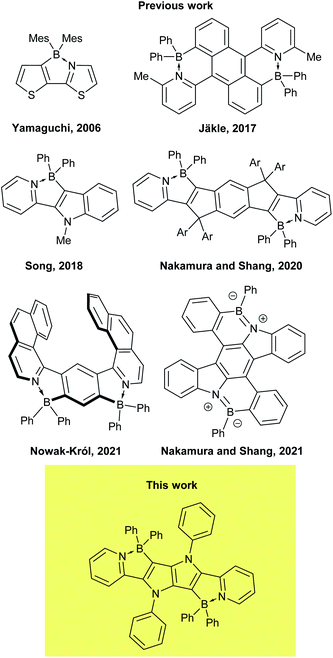 |
| Fig. 1 Chemical structures of the representative dyes possessing a B←N coordination motif. | |
Although the combination of suitable photophysical properties have made TAPPs and fused TAPP analogues popular chromophores for a range of applications, shifting their emission to the red region of the visible spectrum has proved difficult. We have sought to address this issue in this work. Here, we have considered the reaction of pyrrolo[3,2-b]pyrroles bearing 2-pyridil substituents in positions 2 and 5 (C
N bonds in N-heteroaromatic rings are effective in coordinating with boron moieties) with an appropriate boron source (Scheme 1). The resultant B←N
C five membered chelate ring fuses the pyrrolopyrrole and pyridine substituents together and effectively fixes the π-conjugated framework in a coplanar fashion resulting in the formation of a unique chromophore, which perfectly fits into the current intense research on BN-embedded heteroacenes.
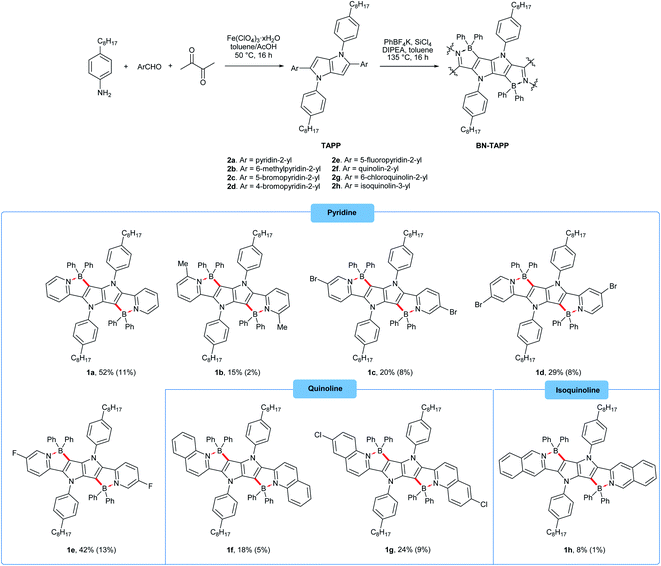 |
| Scheme 1 The synthesis of four-coordinate boron-containing pyrrolo[3,2-b]pyrroles. Isolated yields are given. The overall yields (after two steps) are given in parenthesis. | |
Results and discussion
Design and synthesis
Our approach towards new BN-embedded heterocycles capitalizes on pyrrolo[3,2-b]pyrroles' intrinsically high reactivity at positions 3 and 6, and on the straightforward access to derivatives bearing pyridyl substituents at positions 2 and 5 (Scheme 1). The necessary TAPPs 2a–2e were obtained in good yields from appropriate formylpyridines and 4-octylaniline using our optimized multicomponent condensation (see ESI† for details).42a TAPPs 2f–2h were formed in lower, but sufficient yields from appropriate quinoline- and isoquinoline-derived aldehydes. We anticipated that the planar structure of BN-embedded heterocycles would result in extensive π-stacking, therefore long alkyl chains were installed on the N-linked aryl substituents in order to secure good solubility of the final products. In the next step, we planned to apply a well-known strategy consisting of treatment of the parent pyrrolopyrrole with BBr3 in the presence of base, however, this led to complete decomposition of the starting material. An alternative route was to use diarylchloroboranes, but this seemed difficult due to the limited availability of such reagents. Fortunately, Song and co-workers recently developed a straightforward cascade B–Cl/C–B cross-metathesis and C–H bond borylation procedure,54 which we successfully used in the synthesis of the desired four-coordinated boron-containing pyrrolo[3,2-b]pyrroles 1a–1h (BN-TAPPs) (Scheme 1). According to Song's findings, in this procedure aryltrifluoroborate reacts with SiCl4 to form aryldichloroborane in highly selective manner, which then reacts with another molecule of aryltrifluoroborate to give diphenylchloroborane. Subsequent pyridine directed electrophilic aromatic borylation leads to four-coordinate triarylborane. The yields in the last step are greatly improved when sterically hindered organic base is applied. Importantly, this simple procedure can be conveniently applied to starting materials containing both pyridyl and quinolinyl substituents, and it tolerates the presence of halogens in the starting materials. Consequently, we prepared a few BN-embedded TAPPs substituted with various halogens and submitted them to Sonogashira or Buchwald–Hartwig cross-couplings, in order to further expand the π-system. Although the outcomes of these reactions were not obvious due to the presence of reactive boron and halogen substituents, the desired compounds 1i–1l (Schemes 2 and 3) were isolated in acceptable yields.
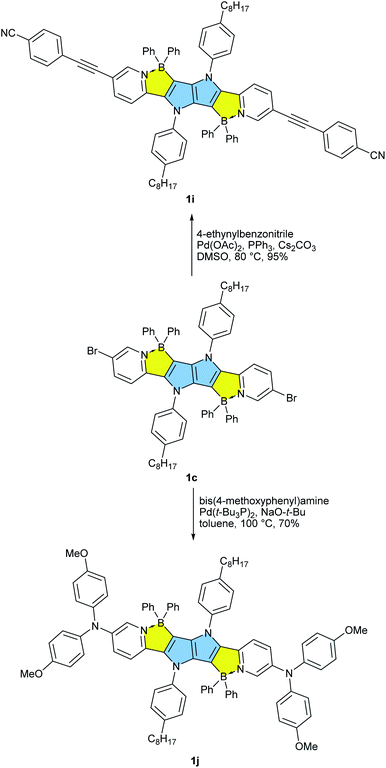 |
| Scheme 2 Post functionalization of BN-TAPP 1c leading towards dyes 1i and 1j. | |
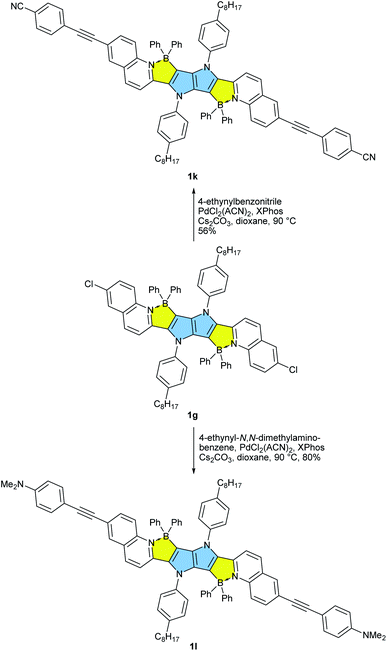 |
| Scheme 3 Post functionalization of BN-TAPP 1g leading towards dyes 1k and 1l. | |
X-ray analysis
The molecular structure of 1a was determined by X-ray crystallography. Single crystals of 1a suitable for analysis were obtained at ambient temperature by slowly diffusing hexanes into a tetrahydrofuran solution. Dye 1a crystallizes in the monoclinic space group P21/c, and the unit cell comprises of two molecules (Fig. 2). The pyrrolo[3,2-b]pyrrole core adopts a perfectly planar structure (180° N–C–C–N torsion angle) and the peripheral pyridine rings are both twisted by 5° from this plane.
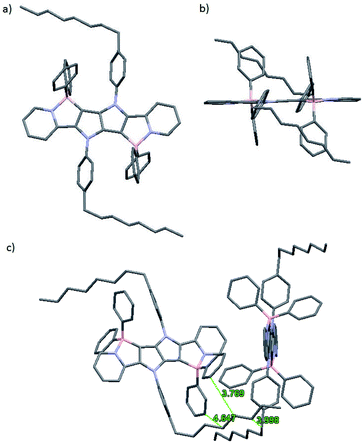 |
| Fig. 2 (a) Top view, (b) side view and (c) crystal packing of 1a as determined from X-ray crystallography (CCDC). Hydrogen atoms were omitted for were omitted for clarity. Distances are given in angstroms. | |
Benzene rings attached to nitrogen atoms of the pyrrolopyrrole core are twisted by 59° and 61°, while phenyl substituents attached to boron atoms are twisted by 83° and 78°. The lengths of the newly formed B–C bonds are 1.6 Å, which is a slightly shorter value than those reported for related compounds and suggests a stronger bond,35,55 while the lengths of the B–N bonds are 1.63 Å, which is similar to the B–N bond length found in analogous compounds55 and BODIPY-type molecules.56 Van der Waals interactions govern the crystal packing, with the main structural motif being the interaction of bent C8 aliphatic chain with two phenyl substituents attached to the boron atom adjacent to the same molecule and then with the octylphenyl substituent attached to the nitrogen atom of the neighboring molecule. No obvious π-stacking interactions are observed, probably due to the orientation of phenyl substituents on the boron atom that prevents these heteroacenes with extended π systems from approaching each other.
To characterize the photophysical properties of the novel dyes we conducted a multipronged campaign. The optical properties of TAPPs 2a–2h correspond very well to those reported earlier for this class of dyes (see ESI† for details).42a The incorporation of boron into the TAPPs backbone, however, brings spectacular changes in their optical properties (Table 1 and Fig. 3). The absorption maxima for compounds 1a–1g are bathochromically shifted by 122–155 nm when compared to parent TAPPs. Only for compound 1h, a somewhat lower, but still remarkable 68 nm red-shift of the absorption maxima was observed, accompanied by an unusual broadening of the band shape and by a low intensity band between 600 and 700 nm (see ESI†). Theoretical calculations indicate that the latter is most likely not connected to the isolated 1h structure (see below), whereas preliminary measurements did not detect formation of aggregates or other related structures. Similarly, the emission maxima were red-shifted by over 100 nm, with very small Stokes shifts of less than 1000 cm−1 and large fluorescence quantum yields for most of the dyes. These results clearly indicate a rigidifying effect of the boron component thanks to the character of boron atoms. Noticeably, in the case of compounds 1k and 1l, in which the π-systems were further expanded, the barrier of 600 nm for both absorption and emission maxima was broken, which means that these are the first examples of pyrrolo[3,2-b]pyrrole derivatives absorbing and emitting in the deep-red region. For dyes 1i, 1k and 1l, exceptionally high extinction coefficients were observed, exceeding 100
000 M−1 cm−1, together with large Φfl. This combination of properties is particularly rare for deep red-emitting dyes due to additional deactivation pathways by vibrational relaxation (energy gap law). A very weak emission was detected at 670 nm for dye 1h. The excitation spectrum however, is not consistent with the absorption profile, which points at potential more complex underlying photophysics to be addressed in subsequent investigations. When compared to recently published, structurally related B/N-doped p-arylenevinylene chromophores,35 BN-TAPPs absorb and emit at much longer wavelengths, with the difference being most noticeable for the derivatives bearing similar, unsubstituted quinoline moieties (63 nm and 80 nm bathochromic shift in the absorption and emission maxima, respectively). p-Arylenevinylene analogues of BN-TAPPs exhibit higher fluorescence quantum yields which are close to being quantitative, although the latter compounds are characterized by much higher molar extinction coefficients, which overall results in a similar emissive brightness for these two classes of organic chromophores.
Table 1 Spectroscopic properties of dyes 1a-1l in toluene
Compound |
λ
maxabs [nm] |
ε
max [M−1 cm−1] |
λ
maxem [nm] |
Stokes shift [cm−1] |
Φ
fl
|
2λmaxabs [nm] |
λ
max2PA [nm] |
σ
max2PA [GM] |
σ
max2PA
Φ
fl [GM] |
Determined with fluorescein in NaOH (0.01 M) as a standard.
Determined with cresyl violet perchlorate in MeOH as a standard.
Fluorescence of dye 1h was not investigated in 2PA due to the inconsistency of the one-photon fluorescence excitation results.
|
1a
|
502 |
42 500 |
521 |
730 |
0.78a |
1004 |
650 |
104 |
80 |
474 |
40 100 |
948 |
380 |
26 800 |
760 |
1b
|
504 |
52 500 |
520 |
610 |
0.77a |
1008 |
660 |
102 |
80 |
476 |
44 100 |
952 |
406 |
18 200 |
812 |
1c
|
528 |
42 800 |
553 |
860 |
0.28a |
1056 |
670 |
188 |
50 |
499 |
42 700 |
998 |
397 |
34 000 |
794 |
1d
|
513 |
48 700 |
533 |
730 |
0.42a |
1026 |
660 |
176 |
70 |
484 |
44 600 |
968 |
392 |
29 200 |
784 |
1e
|
520 |
20 900 |
557 |
1280 |
0.28a |
1040 |
640 |
93 |
25 |
494 |
25 500 |
988 |
391 |
34 500 |
782 |
1f
|
583 |
80 000 |
601 |
510 |
0.78b |
1166 |
940 |
200 |
160 |
542 |
57 600 |
1084 |
379 |
27 600 |
758 |
1g
|
598 |
80 900 |
616 |
490 |
0.75b |
1196 |
920 |
270 |
200 |
555 |
57 900 |
1110 |
388 |
29 800 |
776 |
1h
|
469 |
84 100 |
—c |
—c |
—c |
— |
—c |
— |
— |
1i
|
566 |
134 000 |
589 |
690 |
0.92b |
1132 |
800 |
1400 |
1300 |
431 |
90 700 |
|
862 |
420 |
28 200 |
|
840 |
1j
|
563 |
62 900 |
590 |
810 |
0.75b |
1124 |
790 |
590 |
440 |
533 |
51 600 |
1066 |
424 |
50 500 |
848 |
1k
|
620 |
150 000 |
639 |
480 |
0.78b |
1240 |
760 |
2500 |
2000 |
574 |
93 800 |
1148 |
536 |
37 500 |
1072 |
1l
|
618 |
135 000 |
639 |
530 |
0.88b |
1236 |
870 |
1800 |
1600 |
573 |
81 300 |
1146 |
534 |
29 600 |
1068 |
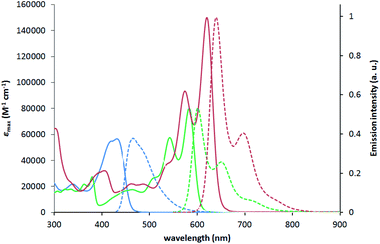 |
| Fig. 3 Absorption (solid lines) and normalized emission (dashed lines) of compounds 2f (blue), 1f (green) and 1k (red) measured in toluene. | |
Photostability
Photostability tests were carried out for compounds 1a and 2a as representative examples (Fig. 4). TAPPs, which generally exhibit very poor stability under irradiation, acquire remarkable photostability upon incorporation of boron, as demonstrated by comparing the properties of 1a with common dyes, such as BODIPY and diketopyrrolopyrroles. Even prolonged exposure to a strong light source did not cause any noticeable changes in the absorption spectrum of 1a.
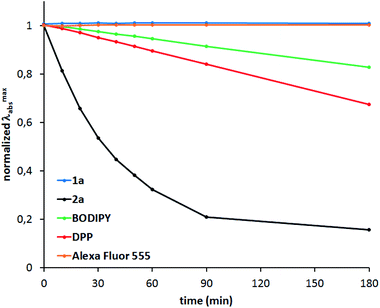 |
| Fig. 4 Photostability of 1a and 2a compared to a BODIPY (BODIPY 493/503), DPP (2,5-dioctyl-3,6-bis(3,4,5-trimethoxyphenyl)pyrrolo[3,4-c]pyrrole-1,4(2H,5H)-dione) and Alexa Fluor 555 measured using a collimated light source from a 300 W Xe lamp. | |
Two-photon absorption
Two-photon absorption studies were performed using a two-photon excited fluorescence (2PEF) method (Table 1 and Fig. 5). Similarly to previously studied symmetric TAPPs,40,43b,c the most prominent two-photon transitions are observed at higher energies, well above the lowest-energy one-photon allowed S0→S1 transition. The latter is only very weakly present in the two-photon spectra, thus following the Laporte rule for low-energy bands. At energies above the S0→S1 transition, the 2PA profiles of 1a-e show similar features comprising a double-band or vibronic progression at 800–950 nm and a distinct peak at ca. 650–670 nm. At <600 nm there is a steep increase of the cross section due to the near-resonance enhancement effect, followed by onset of linear absorption at even shorter wavelengths (not shown). When pyridine is replaced with quinoline (1f, 1g) and further with the addition of diarylamino groups, these spectral features undergo a gradual bathochromic shift. Two-photon absorption was not investigated in the case of dye 1h for two main reasons: (1) the fluorescence intensity is too weak for 2PEF method to be used; (2) the perplexing photophysics of this BN-TAPP has to be delineated before intrinsically more complex non-linear phenomena will be studied. The substantial change, however, comes only after adding strong electron-withdrawing groups at the periphery (dyes 1j and 1k).
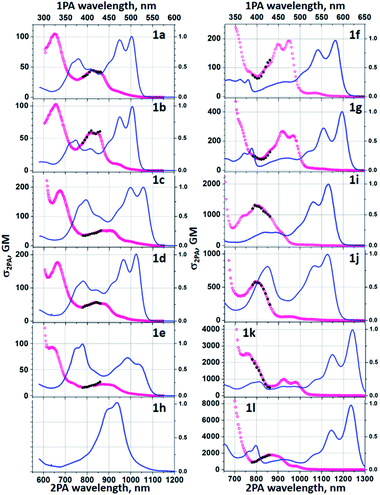 |
| Fig. 5 2PA cross section spectra of 1a–1l in toluene (red symbols, lower horizontal and left vertical axis); Normalized to the peak linear absorption spectra (blue line, upper horizontal and right vertical axis) is shown for comparison. Absolute σ2PA values were measured in the range λ2PA = 780–850 nm (black symbols). | |
In such case the peak σ2 becomes remarkably large, exceeding 103 GM. In the case of the dyes 1k and 1l, the peak values at 760–870 nm reach σ2 = 2500 GM and 1800 GM, respectively, whereas strongly resonance-enhanced values at higher energy become even larger. These values are significantly higher than for simpler A–D–A type TAPPs.43b,c An important feature to note is that the values are higher for the case with the peripheral diaryl-amino groups which would suggest that the new heterocyclic core is somewhat electron-withdrawing.
First-principle studies
Time-Dependent Density Functional Theory (TD-DFT) has been used to explore the nature of the excited-states involved in the BN-TAPPs 1a–1k. The technical details are given in the ESI.†Table 2 summarizes the main results obtained by the calculations.
Table 2 Computed vertical absorption wavelengths (in nm) for the lowest singlet states of all compounds with the corresponding oscillator strengths and 2PA cross-sections (in GM). For the lowest state, we also report the vertical emission wavelength and the computed 0–0 wavelength. See the experimental section for details
Compound |
Vert. λabs |
f
|
σ
2PA
|
Vert. λem |
λ
0–0
|
Nearly dark state after optimization.
|
1a
|
422 |
0.86 |
0 |
493 |
463 |
352 |
0.00 |
94 |
351 |
0.42 |
0 |
322 |
0.26 |
0 |
1b
|
424 |
0.88 |
0 |
496 |
465 |
351 |
0.38 |
0 |
348 |
0.00 |
119 |
314 |
0.23 |
0 |
1c
|
439 |
1.03 |
0 |
514 |
472 |
367 |
0.00 |
122 |
362 |
0.43 |
0 |
336 |
0.34 |
0 |
1d
|
430 |
0.86 |
0 |
502 |
460 |
362 |
0.42 |
0 |
358 |
0.00 |
118 |
330 |
0.30 |
0 |
1e
|
427 |
0.71 |
0 |
500 |
472 |
372 |
0.00 |
74 |
351 |
0.41 |
0 |
335 |
0.41 |
0 |
1f
|
479 |
1.14 |
0 |
560 |
n.d. |
384 |
0.00 |
418 |
379 |
0.39 |
0 |
317 |
0.29 |
3 |
1g
|
494 |
1.17 |
0 |
572 |
534 |
395 |
0.00 |
428 |
394 |
0.43 |
0 |
326 |
0.32 |
4 |
1h
|
424 |
1.04 |
0 |
541a |
484 |
418 |
0.00 |
6 |
417 |
0.77 |
0 |
1i
|
489 |
2.33 |
0 |
572 |
524 |
386 |
0.52 |
0 |
373 |
0.01 |
1790 |
343 |
0.353 |
1 |
1j
|
469 |
1.43 |
0 |
552 |
505 |
410 |
0.00 |
82 |
378 |
0.57 |
0 |
346 |
0.50 |
0 |
334 |
0.00 |
1760 |
1k
|
518 |
2.11 |
0 |
599 |
558 |
405 |
0.57 |
0 |
400 |
0.00 |
1600 |
340 |
0.92 |
5 |
1l
|
516 |
2.26 |
0 |
597 |
560 |
406 |
0.01 |
381 |
395 |
0.61 |
1 |
358 |
0.00 |
10 900 |
Let us start our analysis by the one-photon absorption. In the full series (except for 1h), the S0→S1 transition is well separated from the next one, and is strongly dipole allowed with oscillator strengths ranging from 0.71 to 2.33. The S0→S1 transition undoubtedly corresponds to the observed most red-shifted absorption band. Experimentally, this absorption band is typically composed of two peaks and a shoulder, resulting from vibronic couplings. To ascertain this statement, we have performed vibrationally resolved calculations within the FC-AH approach57 for a simplified version of dye 1f (see Fig. S4†). As can be seen, the overall topology of both the absorption and emission bands is reproduced confirming the vibronic nature of these two peaks. For 1h, the three lowest excited-states are very close according to theory (Table 1), with differences below the TD-DFT accuracy, so that it is not possible to have a definitive information regarding their ordering.
While one cannot compare directly computed vertical absorption wavelengths to experimental λmaxabs, it is also obvious that the trends in the series are reproduced by calculations. Indeed, taking 1a as reference, one notices that the strongest red-shift is obtained for 1c (theory: +17 nm, experiment: +26 nm) in the 1a–1e series. For the six dyes showing the most red-shifted absorption, the experimental order is 1j ∼ 1i < 1f < 1g < 1l ∼ 1k, whereas theory yields: 1j < 1f < 1i ∼ 1g < 1l ∼ 1k.
For all investigated dyes but 1j, the second and third excited states are very close on the energy scale, but possess vastly different oscillator strengths, which is a consequence of the nearly centro-symmetric nature of the investigated dyes. This second (or third) state corresponds to the second weaker absorption band found in the 380–420 nm region experimentally (Tables 1 and 2). For dye 1a, we show density difference plots in Fig. 6. For all three states the central pyrrolopyrrole unit acts as a donor group and the side pyridine rings are accepting moieties. The quadrupolar CT character is, however, not strongly marked for the lowest transition and becomes significantly stronger in the two higher ones. As can be seen, the nature of the state is conserved when going to 1f (derived from quinoline), the contribution of the additional benzene rings being trifling for the first state and remaining limited in the two higher states. In dye 1i, the lowest excited state is partially delocalized on the ethynyl bridges, whereas in 1j, the electron-donating amino groups play their expected role (Fig. 7) explaining the observed redshifts.
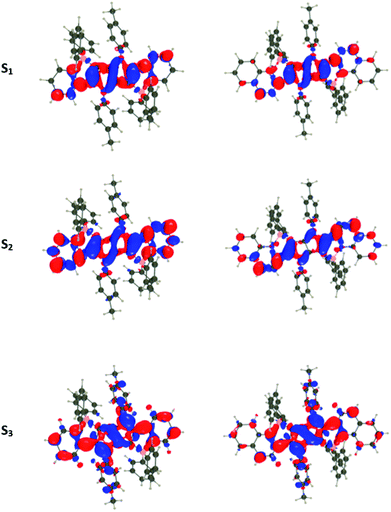 |
| Fig. 6 Density difference plots for the three lowest transitions of 1a (left) and 1f (right). From top to bottom excitation to S1, S2, and S3. The crimson and blueberry lobes indicate an increase and decrease of density upon excitation, respectively. Contour threshold: 0.001 au. | |
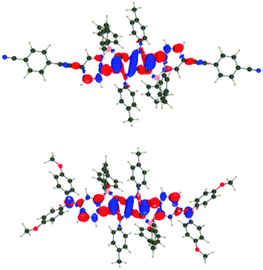 |
| Fig. 7 Density difference plots for the lowest transitions of 1i (top) and 1j (bottom). See caption of Fig. 6 for more details. | |
As far as fluorescence is concerned, for only one dye of the series, namely, 1h, the optimization of the excited-state geometry led to a nearly dark state (f = 0.009), suggesting a very slow radiative decay rate. This is consistent with the trifling emission quantum yield measured experimentally (see Table 1). Indeed the computational studies revealed that the lowest energy allowed transition is markedly blue-shifted placing is energetically close to the dark state. The fact that this pair of opposite-parity electronic states may become almost isoenergetic corroborates the anomalous photophysical behavior of dye 1h (vide infra). Apart from this specific case, one globally finds similar substituent effects as for absorption. In Table 2, we also report the 0–0 wavelengths that offer more physically well-grounded comparisons with experiment,57b and more precisely, with the crossing point between absorption and emission. Illustratively, for 1a, 1j and 1l, we compute λ0–0 of 463, 505, and 560 nm, respectively. These values are blue-shifted by +0.26, 0.31, and 0.24 eV as compared to the measured values of 512, 577, and 629 nm. These errors are on the upper side of the typical TD-DFT errors, which can be explained by the very specific nature of these boron-containing systems: TD-DFT is known to overshoot the transition energies of such states.58
We have also computed two-photon absorption cross sections. In 1a, the experimental rather broad response around 800–900 nm is due to the S0→S2 transition that is dark in one-photon, a typical outcome in quadrupolar dyes. Theory provides a peak σ2PA of 94 GM, of the same order of magnitude as the experimental response (45 GM). This response remains of the same order of magnitude but is slightly increased in 1b, 1c, and 1d, but slightly decreased in 1e, which holds both in the experiment and in the simulations. In 1f, the σ2PA attains the respectable value of ca. 220 GM experimentally, and it is clear that it is again the second state that is responsible for this response (418 GM theoretically). The situation is totally similar in 1g. In the more extended 1i, the calculations indicate that the 2PA response comes from the S0→S3 transition, which is consistent with the experimental spectra. Again, theory gives value that closely matches the experiment (1500 GM versus 1400 GM). In 1i, the experimental 2PA spectrum shows two bands, one moderately active due to the second transition, and one much more intense due to the fifth state. In 1k, the second and third states appear at almost the same energy but have different (pseudo) symmetry, one being active in one-photon, the other in two-photon absorption, potentially explaining the shape of the experimental spectra resembling a superposition between the two phenomena. The computed response is 1600 GM for the 2PA-active transition, again with the same order of magnitude as the experimental measurement. Eventually, the analysis is more difficult in 1l, but it appears that the second excited state should be responsible for the shoulder in the 2PA experimental spectrum at ca. 475 nm, whereas the fourth transition likely yields the much more intense peaks at ca. 440 nm.
Electrochemical properties
BN-TAPPs 1a–1l are characterized by the presence of a reversible redox pair of the first oxidation process (Fig. 8, red CVs, Table 3) which according to its potential value can be tentatively attributed to the oxidation of the N,N′-diphenylpyrrolo[3,2-b]pyrrole core. An exception is 1c where partial reversibility was found, the cause of which was further investigated during spectroelectrochemical measurements (vide infra). The lowest value of the first oxidation potential in the entire BN-TAPP series (0.11 V) was recorded for the derivative 1h with isoquinoline scaffolds. For the remaining dyes the first oxidation occurs between 0.25 and 0.45 V which corresponds to HOMO values in the range (−5.26 eV)–(−5.43 eV) (Table 3). Compared to structurally analogous dyes possessing two thiophene rings BN-TAPPs are slightly less electron-rich but they are more electron-rich than dyes π-expanded TAPPs possessing two thiophene-S,S-dioxide moieties.52b
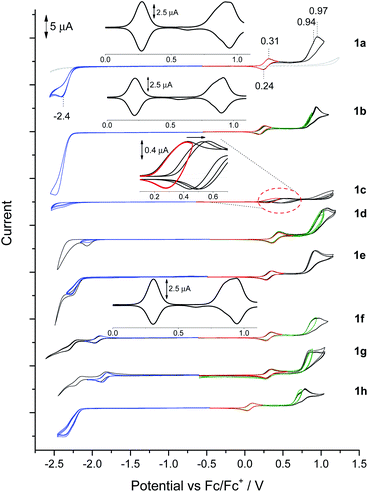 |
| Fig. 8 Cyclic voltammograms in the solution of BN-TAPP-series compounds (1 mM) in 0.1 M Bu4NBF4/DCM. Oxidation (red, black CVs), reduction (blue, black CVs), second oxidation (green, black CVs). | |
Table 3 Potential of cathodic peak (cp) and anodic peak (ap) of oxidation (ox) and reduction (red) processes of BN-TAPPs (1 mM) in 0.1 M Bu4NBF4/DCM; values in volts vs. Fc/Fc+ couple; potential sweep rate 0.05 V s−1
Compound |
E
1ox
|
E
2aox
|
E
2box
|
E
1red
|
E
2red
|
HOMO (eV) |
LUMO (eV) |
E
1ap
|
E
1cp
|
E
2aap
|
E
2bap
|
E
1cp
|
E
1ap
|
E
2cp
|
1a
|
0.31 |
0.24 |
0.94 |
0.97 |
−2.40 |
— |
— |
−5.30 |
−2.84 |
1b
|
0.25 |
0.19 |
0.87 |
0.93 |
−2.48 |
— |
— |
−5.26 |
−2.81 |
1c
|
0.42 |
0.28 |
— |
— |
−2.38 |
— |
— |
−5.31 |
−2.81 |
1d
|
0.45 |
0.36 |
1.02 |
1.08 |
−2.04 |
— |
— |
−5.43 |
−3.13 |
1e
|
0.34 |
0.26 |
0.90 |
— |
−2.30 |
— |
— |
−5.34 |
−2.98 |
1f
|
0.35 |
0.28 |
0.93 |
0.97 |
−1.97 |
−1.90 |
−2.32 |
−5.35 |
−3.24 |
1g
|
0.41 |
0.34 |
0.92 |
1.01 |
−1.78 |
−0.68 |
−1.11 |
−5.35 |
−3.32 |
1h
|
0.11 |
0.05 |
0.74 |
0.81 |
−2.46 |
— |
— |
−5.12 |
−2.96 |
The second oxidation curve is partially irreversible in all cases (Fig. 8, green and black CVs) and shows a dual nature more visible in differential pulse voltammetry (DPV) experiments (Fig. 8, e.g.1a and 1b – inserts). The double peak of the second oxidation state may indicate a slight difference in oxidation potential between both peripheral units, which was also registered in separate processes as green and black CV curves with the peak potential marked as E2aox and E2box, respectively. Dyes 1h and 1d possess, respectively, the lowest (0.74 V) and highest (1.02 V) potential of E2aox. Irreversibility of the second oxidation does not impact on the potential and reversibility of the first redox couple in subsequent CV cycles after polarity reversal.
DPV measurements also show that the peak areas of the first and second oxidation peaks are comparable, which indicates the full oxidation of the N,N′-diphenylpyrrolo[3,2-b]pyrrole scaffold under the potential of the first oxidation peak to dication or di(radical cation), while the second oxidation peak is associated with the oxidation of both the peripheral moieties.
One irreversible reduction process was registered for BN-TAPPs 1a–1e bearing two pyridine moieties at (−2.40)–(−2.04 V). In the case of dye 1f (bearing two quinoline moieties), we observed two reduction processes, and the first was fully reversible. The values of reduction were shifted towards positive potentials, compared with the pyridine series.
Spectroelectrochemistry
Changes in the UV-Vis-NIR absorption spectra during polarization within the first oxidation peak revealed a decrease in the intensity of the bands at 468, 492 nm (1a) and 495 and 522 nm (1c)59 (Fig. 9), while the shift of the absorption in the infrared direction of the output bands of 1c indicates a decrease in the energy of the π–π* transition due to a partial localization of the HOMO orbital also on the pyridine rings. Polarization within the first oxidation peak causes an increase in the bands at 530, 734 nm (1a) and 579, 773 nm (1c), characteristic for a di(radical cationic) state, as was observed in a previous spectroelectrochemical study of dyes based on the pyrrolo[3,2-b]pyrrole scaffold.52b The gradual formation of a radical cation and its transition to di(radical cation) was not observed.
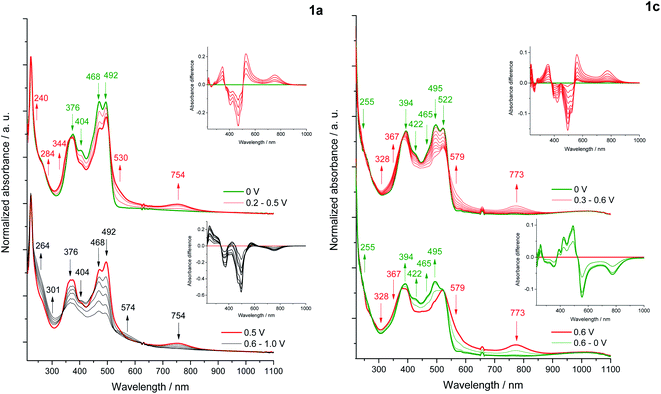 |
| Fig. 9
In situ UV-Vis-NIR spectra of 1a (left) and 1c (right); differential UV-Vis-NIR absorbance (insets) in 1 mM sample solution in 0.1 M Bu4NBF4/DCM within the potential of the first (red) (1a, 1c) and second (black) oxidation peaks (1a); at 0 V or the returning to 0 V (green). | |
ESR spectroelectrochemical measurements were performed for representative dyes 1a and 1c, where the reversible first redox process is associated with the formation of a di(radical cation) in both cases. Unpaired electrons of the di(radical cation) of 1a are located on the N,N′-diphenylpyrrolo[3,2-b]pyrrole core and are characterized by a low value of the g-factor (g = 2.0023). In turn, the ESR signal recorded under polarization of the first oxidation state of 1c gives the g-factor equal 2.0050, which may indicate the coupling of one of the electrons of the di(radical cation) with nitrogen and/or boron atoms, as proposed in Scheme S1.† Although the ESR signals are quite broad in both cases, which may indicate the cleavage of the energy levels of the unpaired electron by the nuclei coupled to it, the hyperfine structure was not recorded.
Conclusions
In summary, we have designed, synthesized and fully characterized a new family of BN-embedded heteroacenes. This was achieved through incorporation of duel cyclopenta[c][1,2]azaborole moieties with a pyrrolo[3,2-b]pyrrole core in a straightforward two-step synthetic procedure. The B−–N+ dative bond reduces the HOMO–LUMO gap of the parent dye, which results in a marked red-shift of absorption and emission and almost quantitative fluorescence quantum yield. Their excellent properties, such as superb photostability, strong absorption and intense emission in the orange to deep red region, together with large two-photon absorption cross sections and rich electrochemistry, opens the door for future applications in optoelectronics. Given the recent renaissance of interest in boron-doped PAHs and their related B–N/O isosteres, this work should inspire the future design and synthesis of pyrrolopyrrole and related frameworks with distinctive π-expanded architectures.
Data availability
Data associated with this article, including experimental procedures, compound characterization, steady-state absorption and emission along with the two-photon absorption details, electrochemical details and computational analysis details are available in the ESI.†
Author contributions
M. T. conceived the idea and wrote the manuscript. M. T., P. K and M. P. performed all synthetic experiments including condition optimizations and exploring the scope. M. C. and P. J. performed electrochemical and spectroelectrochemical measurements. M. B. performed several TD-DFT calculations, and vibronic analysis. M. R. performed 2PA measurements. M. Ł. wrote formal electrochemical analysis and reviewed the manuscript. A. R. performed 2PA measurements, wrote formal analysis of this part of the manuscript and reviewed the manuscript. D. J. performed DFT and TD-DFT calculations, analyzed data, wrote and reviewed the manuscript. D. G. supervised the project, performed formal analysis, wrote and reviewed the manuscript. All the authors discussed the results and commented on the manuscript.
Conflicts of interest
There are no conflicts to declare.
Acknowledgements
The work was financially supported by the Polish National Science Centre, Poland (HARMONIA 2018/30/M/ST5/00460), the Foundation for Polish Science (TEAM POIR.04.04.00-00-3CF4/16-00) and EC (MSC ITN CHAIR). A.R. and M.R. acknowledge support by the Ministry of Education and Research, Republic of Estonia grant PRG661 and A.R. by National Science Foundation award CHE 2103628. The authors also thank Dr David C. Young for proofreading the manuscript. M.H.E. and D.J. thank the CCIPL computational center installed in Nantes for generous allocation of computational time.
Notes and references
- M. Yang, I. S. Park and T. Yasuda, J. Am. Chem. Soc., 2020, 142, 19468–19472 CrossRef CAS PubMed.
-
(a) J. A. Knçller, G. Meng, X. Wang, D. Hall, A. Pershin, D. Beljonne, Y. Olivier, S. Laschat, E. Zysman-Colman and S. Wang, Angew. Chem., Int. Ed., 2020, 59, 3156–3160 CrossRef;
(b) Y. Chen, W. Chen, Y. Qiao, X. Lu and G. Zhou, Angew. Chem., Int. Ed., 2020, 59, 7122–7130 CrossRef CAS.
- R. E. Messersmith, S. Yadav, M. A. Siegler, H. Ottosson and J. D. Tovar, J. Org. Chem., 2017, 82, 13440–13448 CrossRef CAS.
- F. Qiu, F. Zhang, R. Tang, Y. Fu, X. Wang, S. Han, X. Zhuang and X. Feng, Org. Lett., 2016, 18, 1398–1401 CrossRef CAS PubMed.
- M. Numano, N. Nagami, S. Nakatsuka, T. Katayama, K. Nakajima, S. Tatsumi, N. Yasuda and T. Hatakeyama, Chem.–Eur. J., 2016, 22, 11574–11577 CrossRef CAS.
- G. Meng, T. Peng, Y. Shi, H. Li, X. Wang, X. Yin, D.-T. Yang, S. Wang and N. Wang, J. Mater. Chem. C, 2020, 8, 7749 RSC.
-
(a) V. M. Hertz, M. Bolte, H.-W. Lerner and M. Wagner, Angew. Chem., Int. Ed., 2015, 54, 8800–8804 CrossRef CAS;
(b) M. Fingerle, J. Dingerkus, H. Schubert, K. M. Wurst, M. Scheele and H. F. Bettinger, Angew. Chem., Int. Ed., 2021, 60, 15798–15802 CrossRef CAS PubMed;
(c) M. Fingerle, C. Maichle-Mössmer, S. Schundelmeier, B. Speiser and H. F. Bettinger, Org. Lett., 2017, 19, 4428–4431 CrossRef CAS PubMed.
-
(a) C. J. Saint-Louis, L. L. Magill, J. A. Wilson, A. R. Schroeder, S. E. Harrell, N. S. Jackson, J. A. Trindell, S. Kim, A. R. Fisch, L. Munro, V. J. Catalano, C. E. Webster, P. P. Vaughan, K. S. Molek, A. K. Schrock and M. T. Huggins, J. Org. Chem., 2016, 81, 10955–10963 CrossRef CAS;
(b) A. Caruso Jr, M. A. Siegler and J. D. Tovar, Angew. Chem., Int. Ed., 2010, 49, 4213–4217 CrossRef.
-
(a) A. Abengózar, D. Sucunza, P. García-García, D. Sampedro, A. Pérez-Redondo and J. J. Vaquero, J. Org. Chem., 2019, 84, 7113–7122 CrossRef PubMed;
(b) A. Wakamiya, K. Mori and S. Yamaguchi, Angew. Chem., Int. Ed., 2007, 46, 4273–4276 CrossRef CAS PubMed.
- K. Kamada, T. Namikawa, S. Senatore, C. Matthews, P.-F. Lenne, O. Maury, C. Andraud, M. Ponce-Vargas, B. LeGuennic, D. Jacquemin, P. Agbo, D. D. An, S. S. Gauny, X. Liu, R. J. Abergel, F. Fages and A. D'Aléo, Chem.–Eur. J., 2016, 22, 5219–5232 CrossRef CAS PubMed.
-
(a) C. Reus, S. Weidlich, M. Bolte, H.-W. Lerner and M. Wagner, J. Am. Chem. Soc., 2013, 135, 12892–12907 CrossRef CAS;
(b) N. Ando, T. Yamada, H. Narita, N. N. Oehlmann, M. Wagner and S. Yamaguchi, J. Am. Chem. Soc., 2021, 143, 9944–9951 CrossRef CAS;
(c) K. Schickedanz, J. Radtke, M. Bolte, H.-W. Lerner and M. Wagner, J. Am. Chem. Soc., 2017, 139, 2842–2851 CrossRef CAS PubMed;
(d) P.-F. Zhang, J.-C. Zeng, F.-D. Zhuang, K.-X. Zhao, Z.-H. Sun, Z.-F. Yao, Y. Lu, X.-Y. Wang, J.-Y. Wang and J. Pei, Angew. Chem., Int. Ed., 2021, 60, 23313–23319 CrossRef CAS;
(e) C. Li, Y. Shi, P. Li, N. Zhang, N. Wang, X. Yin and P. Chen, Org. Lett., 2021, 23, 7123–7128 CrossRef CAS.
- B. Adelizzi, P. Chidchob, N. Tanaka, B. A. G. Lamers, S. C. J. Meskers, S. Ogi, A. R. A. Palmans, S. Yamaguchi and E. W. Meijer, J. Am. Chem. Soc., 2020, 142, 16681–16689 CrossRef CAS.
- Y.-Q. Yan, Y.-B. Li, J.-W. Wang and C.-H. Zhao, Chem.–Asian J., 2013, 8, 3164–3176 CrossRef CAS.
- G. Li, Y. Zhao, J. Li, J. Cao, J. Zhu, X.-W. Sun and Q. Zhang, J. Org. Chem., 2015, 80, 196–203 CrossRef CAS PubMed.
- T. Hatakeyama, K. Shiren, K. Nakajima, S. Nomura, S. Nakatsuka, K. Kinoshita, J. Ni, Y. Ono and T. Ikuta, Adv. Mater., 2016, 28, 2777–2781 CrossRef CAS.
- Y. Xia, M. Zhang, S. Ren, J. Song, J. Ye, M. G. Humphrey, C. Zheng, K. Wang and X. Zhang, Org. Lett., 2020, 22, 7942–7946 CrossRef CAS PubMed.
- J. Full, S. P. Panchal, J. Götz, A.-M. Krause and A. Nowak-Król, Angew. Chem., Int. Ed., 2021, 60, 4350–4357 CrossRef CAS.
- M. Pinheiro Jr, F. B. C. Machado, F. Plasser, A. J. A. Aquino and H. Lischka, J. Mater. Chem. C, 2020, 8, 7793–7804 RSC.
- For review articles, see:
(a) A. Wakamiya and S. Yamaguchi, Bull. Chem. Soc. Jpn., 2015, 88, 1357–1377 CrossRef CAS;
(b) S. K. Mellerup and S. Wang, Trends Chem., 2019, 1, 77–89 CrossRef CAS;
(c) S. Agnoli and M. Favaro, J. Mater. Chem. A, 2016, 4, 5002–5025 RSC;
(d) J. Huang and Y. Li, Front. Chem., 2018, 6, 341 CrossRef PubMed;
(e) J. Huang, X. Wang, Y. Xiang, L. Guo and G. Chen, Advanced Energy and Sustainability Research, 2021, 2, 2100016 CrossRef;
(f) S. Mukherjee and P. Thilagar, J. Mater. Chem. C, 2016, 4, 2647–2662 RSC.
- X.-Y. Wang, F.-D. Zhuang, R.-B. Wang, X.-C. Wang, X.-Y. Cao, J.-Y. Wang and J. Pei, J. Am. Chem. Soc., 2014, 136, 3764–3767 CrossRef CAS PubMed.
- F. Miyamoto, S. Nakatsuka, K. Yamada, K. Nakayama and T. Hatakeyama, Org. Lett., 2015, 17, 6158–6161 CrossRef CAS PubMed.
- S. Osumi, S. Saito, C. Dou, K. Matsuo, K. Kume, H. Yoshikawa, K. Awaga and S. Yamaguchi, Chem. Sci., 2016, 7, 219–227 RSC.
- H. L. van de Wouw, J. Y. Lee, M. A. Siegler and R. S. Klausen, Org. Biomol. Chem., 2016, 14, 3256–3263 RSC.
- H. Helten, Chem, 2016, 22, 12972–12982 CrossRef CAS.
- Y. Zhang, C. Zhang, Y. Guo, J. Ye, B. Zhen, Y. Chen and X. Liu, J. Org. Chem., 2021, 86, 6322–6330 CrossRef CAS PubMed.
- J. Zhou, R. Tang, X. Wang, W. Zhang, X. Zhuang and F. Zhang, J. Mater. Chem. C, 2016, 4, 1159 RSC.
- S. Nakatsuka, N. Yasuda and T. Hatakeyama, J. Am. Chem. Soc., 2018, 140, 13562–13565 CrossRef CAS PubMed.
- H. Xin, J. Li, X. Yang and X. Gao, J. Org. Chem., 2020, 85, 70–78 CrossRef CAS.
-
(a) A. Wakamiya, T. Taniguchi and S. Yamaguchi, Angew. Chem., Int. Ed., 2006, 45, 3170–3173 CrossRef CAS;
(b) Y. Min, C. Dou, H. Tian, J. Liu and L. Wang, Chem.–Eur. J., 2021, 27, 4364–4372 CrossRef CAS;
(c) S. A. Iqbal, K. Yuan, J. Cid, J. Pahl and M. J. Ingleson, Org. Biomol. Chem., 2021, 13, 2949–2958 RSC;
(d) K. Liu, R. A. Lalancette and F. Jäkle, J. Am. Chem. Soc., 2017, 139, 18170–18173 CrossRef CAS;
(e) T. Sakamaki, T. Nakamuro, K. Yamashita, K. Hirata, R. Shang and E. Nakamura, Chem. Mater., 2021, 33, 5337–5344 CrossRef CAS.
-
(a) F. -D. Zhuang, Z. -H. Sun, Z. -F. Yao, Q. -R. Chen, Z. Huang, J. -H. Yang, J. -Y. Wang and J. Pei, Angew. Chem., Int. Ed., 2019, 58, 10708–10712 CrossRef CAS;
(b) A. Abengózar, I. Valencia, G. G. Otárola, D. Sucunza, P. García-García, A. Pérez-Redondo, F. Mendicuti and J. J. Vaquero, Chem. Commun., 2020, 56, 3669–3672 RSC;
(c) X. -Y. Wang, D. -C. Yang, F. -D. Zhuang, J. -J. Liu, J. -Y. Wang and J. Pei, Chem.–Eur. J., 2015, 21, 8867–8873 CrossRef CAS PubMed;
(d) A. Abengózar, P. García-García, D. Sucunza, A. Pérez-Redondo and J. J. Vaquero, Chem. Commun., 2018, 54, 2467–2470 RSC;
(e) G. Li, W.-W. Xiong, P.-Y. Gu, J. Cao, J. Zhu, R. Ganguly, Y. Li, A. C. Grimsdale and Q. Zhang, Org. Lett., 2015, 17, 560–563 CrossRef CAS;
(f) Y. Chen, W. Chen, Y. Qiao and G. Zhou, Chem.–Eur. J., 2019, 25, 9326–9338 CrossRef CAS PubMed;
(g) D.-T. Yang, Y. Shi, T. Peng and S. Wang, Organometallics, 2017, 36, 2654–2660 CrossRef CAS;
(h) H. Huang, Y. Zhou, Y. Wang, X. Cao, C. Han, G. Liu, Z. Xu, C. Zhan, H. Hu, Y. Peng, P. Yana and D. Cao, J. Mater. Chem. A, 2020, 8, 22023–22031 RSC.
- P. B. Pati, E. Jin, Y. Kim, Y. Kim, J. Mun, S. J. Kim, S. J. Kang, W. Choe, G. Lee, H.-J. Shin and Y. S. Park, Angew. Chem., Int. Ed., 2020, 59, 14891–14895 CrossRef CAS.
- M. Ando, M. Sakai, N. Ando, M. Hirai and S. Yamaguchi, Org. Biomol. Chem., 2019, 17, 5500 RSC.
-
(a) D. C. Young, M. Tasior, A. D. Laurent, Ł. Dobrzycki, M. K. Cyrański, N. Tkachenko, D. Jacquemin and D. T. Gryko, J. Mater. Chem. C, 2020, 8, 7708–7717 RSC;
(b) S. Shimizu, T. Iino, A. Saeki, S. Seki and N. Kobayashi, Chem.–Eur. J., 2015, 21, 2893–2904 CrossRef CAS;
(c) Y. Kage, S. Mori, M. Ide, A. Saeki, H. Furuta and S. Shimizu, Mater. Chem. Front., 2018, 2, 112–120 RSC;
(d) S. Shimizu, T. Iino, Y. Araki and N. Kobayashi, Chem. Commun., 2013, 49, 1621–1623 RSC;
(e) T. Marks, E. Daltrozzo and A. Zumbusch, Chem.–Eur. J., 2014, 20, 6494–6504 CrossRef CAS;
(f) T. Nakano, A. Sumida and K. Naka, J. Org. Chem., 2021, 86, 5690–5701 CrossRef CAS PubMed.
- K. Yang, G. Zhang and Q. Song, Chem. Sci., 2018, 9, 7666 RSC.
- H. Lu, T. Nakamuro, K. Yamashita, H. Yanagisawa, O. Nureki, M. Kikkawa, H. Gao, J. Tian, R. Shang and E. Nakamura, J. Am. Chem. Soc., 2020, 142, 18990–18996 CrossRef CAS.
- H. Shimogawa, Y. Murata and A. Wakamiya, Org. Lett., 2018, 20, 5135–5138 CrossRef CAS PubMed.
- C. Yu, Z. Huang, X. Wang, W. Miao, Q. Wu, W.-Y. Wong, E. Hao, Y. Xiao and L. Jiao, Org. Lett., 2018, 20, 4462–4466 CrossRef CAS.
-
(a) D. Li, H. Zhang and Y. Wang, Chem. Soc. Rev., 2013, 42, 8416–8433 RSC;
(b) R. Zhao, J. Liu and L. Wang, Acc. Chem. Res., 2020, 53, 1557–1567 CrossRef CAS PubMed;
(c) J. Huang and Y. Li, Front. Chem., 2018, 6, 341 CrossRef PubMed.
- S. Pios, X. Huang, A. S. Sobolewski and W. Domcke, Phys. Chem. Chem. Phys., 2021, 23, 12968–12975 RSC.
- M. Krzeszewski, D. Gryko and D. T. Gryko, Acc. Chem. Res., 2017, 50, 2334–2345 CrossRef CAS.
- M. E. Cinar and T. Ozturk, Chem. Rev., 2015, 115, 3036–3140 CrossRef CAS.
-
(a) M. Tasior, B. Koszarna, D. C. Young, B. Bernard, D. Jacquemin, D. Gryko and D. T. Gryko, Org. Chem. Front., 2019, 6, 2939–2948 RSC;
(b) A. Janiga, E. Glodkowska-Mrowka, T. Stoklosa and D. T. Gryko, Asian J. Org. Chem., 2013, 2, 411–415 CrossRef CAS.
-
(a) A. I. Ivanov, B. Dereka and E. Vauthey, J. Chem. Phys., 2017, 146, 164306 CrossRef PubMed;
(b) D. H. Friese, A. Mikhaylov, M. Krzeszewski, Y. M. Poronik, A. Rebane, K. Ruud and D. T. Gryko, Chem.–Eur. J., 2015, 21, 18364–18374 CrossRef CAS PubMed;
(c) Ł. G. Łukasiewicz, H. G. Ryu, A. Mikhaylov, C. Azarias, M. Banasiewicz, B. Kozankiewicz, K. H. Ahn, D. Jacquemin, A. Rebane and D. T. Gryko, Chem.–Asian J., 2017, 12, 1736–1748 CrossRef PubMed;
(d) B. Bardi, M. Krzeszewski, D. T. Gryko, A. Painelli and F. Terenziani, Chem.–Eur. J., 2019, 25, 13930–13938 CrossRef CAS PubMed.
- B. Dereka and E. Vauthey, Chem. Sci., 2017, 8, 5057–5066 RSC.
- J.-Y. Wu, C.-H. Yu, J.-J. Wen, C.-L. Chang and M.-k. Leung, Anal. Chem., 2016, 88, 1195–1201 CrossRef CAS.
- Y. Zhou, M. Zhang, J. Ye, H. Liu, K. Wang, Y. Yuan, Y.-Q. Du, C. Zhang, C.-J. Zheng and X.-H. Zhang, Org. Electron., 2019, 65, 110–115 CrossRef CAS.
- R. K. Canjeevaram Balasubramanyam, R. Kumar, S. J. Ippolito, S. K. Bhargava, S. R. Periasamy, R. Narayan and P. Basak, J. Phys. Chem. C, 2016, 120, 11313–11323 CrossRef CAS.
- R. Domínguez, N. F. Montcada, P. de la Cruz, E. Palomares and F. Langa, ChemPlusChem, 2017, 82, 1096–1104 CrossRef PubMed.
- J. Wang, Z. Chai, S. Liu, M. Fang, K. Chang, M. Han, L. Hong, H. Han, Q. Li and Z. Li, Chem.–Eur. J., 2018, 24, 18032–18042 CrossRef CAS.
-
(a) B. Sadowski, K. Hassanein, B. Ventura and D. T. Gryko, Org. Lett., 2018, 20, 3183–3186 CrossRef CAS;
(b) Y. Ji, Z. Peng, B. Tong, J. Shi, J. Zhi and Y. Dong, Dyes Pigm., 2017, 139, 664–671 CrossRef CAS;
(c) K. Li, Y. Liu, Y. Li, Q. Feng, H. Hou and B. Z. Tang, Chem. Sci., 2017, 8, 7258–7267 RSC;
(d) Y. Ma, Y. Zhang, L. Kong and J. Yang, Molecules, 2018, 23, 3255 CrossRef PubMed.
- C. S. Hawes, G. M. ÓMáille, K. Byrne, W. Schmitt and T. Gunnlaugsson, Dalton Trans., 2018, 47, 10080–10092 RSC.
-
(a) S. Mishra, M. Krzeszewski, C. A. Pignedoli, P. Ruffieux, R. Fasel and D. T. Gryko, Nat. Commun., 2018, 9, 1714 CrossRef;
(b) M. Tasior, M. Czichy, M. Łapkowski and D. T. Gryko, Chem.–Asian J., 2018, 13, 449–456 CrossRef CAS PubMed;
(c) D. Wu, J. Zheng, C. Xu, D. Kang, W. Hong, Z. Duan and F. Mathey, Dalton Trans., 2019, 48, 6347–6352 RSC.
-
(a) M. Tasior and D. T. Gryko, J. Org. Chem., 2016, 81, 6580–6586 CrossRef CAS PubMed;
(b) M. Krzeszewski, Ł. Dobrzycki, A. L. Sobolewski, M. K. Cyrański and D. T. Gryko, Angew. Chem., Int. Ed., 2021, 60, 14998–15005 CrossRef CAS PubMed.
- K. Yang, G. Zhang and Q. Song, Chem. Sci., 2018, 9, 7666–7672 RSC.
- D. Kunchala, S. Sa, P. Nayak, S. J. Ponniah and K. Venkatasubbaiah, Organometallics, 2019, 38, 870 CrossRef CAS.
- M. Más-Montoya, M. F. Montenegro, A. E. Ferao, A. Tárraga, J. N. Rodríguez-López and D. Curiel, Org. Lett., 2020, 22, 3356 CrossRef.
-
(a) F. Santoro, R. Improta, A. Lami, J. Bloino and V. Barone, J. Chem. Phys., 2007, 126, 084509 CrossRef;
(b) F. Santoro and D. Jacquemin, Wiley Interdiscip. Rev.: Comput. Mol. Sci., 2016, 6, 460–486 CAS;
(c)
J. Cerezo and F. Santoro, FCClasses 3.0, http://www.pi.iccom.cnr.it/fcclasses Search PubMed.
- B. Le Guennic and D. Jacquemin, Acc. Chem. Res., 2015, 48, 530–537 CrossRef CAS PubMed.
- M. Tasior, O. Vakuliuk, D. Koga, B. Koszarna, K. Górski, M. Grzybowski, Ł. Kielesiński, M. Krzeszewski and D. T. Gryko, J. Org. Chem., 2020, 85, 13529–13543 CrossRef CAS PubMed.
Footnote |
† Electronic supplementary information (ESI) available: Details of the synthesis and spectroscopic characterization of new compounds. CCDC 2101152. For ESI and crystallographic data in CIF or other electronic format see DOI: 10.1039/d1sc05007a |
|
This journal is © The Royal Society of Chemistry 2021 |
Click here to see how this site uses Cookies. View our privacy policy here.