DOI:
10.1039/D1GC03549E
(Paper)
Green Chem., 2022,
24, 218-226
Glyoxylate carboligase-based whole-cell biotransformation of formaldehyde into ethylene glycol via glycolaldehyde†
Received
27th September 2021
, Accepted 18th November 2021
First published on 19th November 2021
Abstract
A novel biocatalytic system for the synthesis of industrially relevant C2 chemicals (e.g., ethylene glycol (3)) from formaldehyde (1) was established. The biocatalytic system consisted of a newly discovered thermostable glyoxylate carboligase from Escherichia coli K-12 (EcGCL) and a lactaldehyde reductase (FucO) of E. coli K-12. EcGCL's affinity for formaldehyde was first improved by engineering the substrate access tunnel. One of the variants (i.e., EcGCLR484MN283QL478M) showed a high substrate affinity and catalytic efficiency of 18 mM and 5.2 M−1 s−1, respectively, for the condensation of two molecules of formaldehyde into one molecule of glycolaldehyde. The recombinant E. coli cells expressing both EcGCLR484MN283QL478M and FucO produced ethylene glycol (3) up to 6.6 mM from formaldehyde (1) with a bioconversion of 66% via glycolaldehyde (2), without leaving the reactants (1 and 2) in the reaction medium. This study demonstrated the biocatalytic synthesis of ethylene glycol from C1 compounds in an environment-friendly way.
Introduction
Formaldehyde (HCHO) is an emerging C1 source because it can be prepared from CO, CO2, HCOOH, and CH4 by biological and/or chemical means.1,2 However, it is challenging to convert formaldehyde into value-added molecules. It is a gas under atmospheric conditions and its aqueous solution, formalin, is not fully defined yet and exhibits stability issues.1 Moreover, formaldehyde is symmetrical and very reactive. In case of enzyme catalysis, protein deactivation or even denaturation may take place by the cross-linking reaction of formaldehyde with nucleophilic amino acid residues (e.g., cysteine).3
Enzymatic valorization of formaldehyde had been pursued by a number of research groups.4 First of all, Siegel et al.5 reported a computationally designed enzyme, formolase (FLS), which catalyzes the carboligation of three one-carbon formaldehyde molecules (1) into one three-carbon dihydroxyacetone molecule (4) or the condensation of two molecules of glycolaldehyde (2) into one molecule of four-carbon erythrulose (5)6 (Scheme S1†). However, FLS was not suitable for the preparation of glycolaldehyde (2) from formaldehyde (1). Another example has been recently reported by Lu et al.7 They investigated the engineering of a benzoylformate decarboxylase of Pseudomonas putida to produce glycolaldehyde from formaldehyde. The benzoylformate decarboxylase was evolved into glycolaldehyde synthase (GALS) via multiple mutations (Scheme S1†). However, this enzyme showed a rather high KM value for formaldehyde (i.e., 170 mM). The biosynthesis of glycolic acid from C1 compounds using 2-hydroxyacyl-coenzyme A (CoA) lyase as the key enzyme has also been recently reported,8 but the enzyme required formyl-CoA as a cosubstrate.
The production of ethylene glycol from biomass has been extensively examined;9,10 ethanol, which was prepared from glucose by yeast fermentation, was chemically converted into ethylene glycol.9 Another approach was to produce ethylene glycol from sugars (e.g., xylose and glucose) by metabolically engineered microbial cells.11 However, these processes are rather complicated, and generated byproducts. Therefore, a simple and green way to produce ethylene glycol remains to be investigated.
In this study, a novel method to biosynthesize ethylene glycol from formaldehyde, which could be prepared from C1 gas, has been examined. First, a novel carboligase (i.e., glyoxylate carboligase from Escherichia coli K-12 (EcGCL)), which catalyzes the condensation of two molecules of formaldehyde (1) into one molecule of glycolaldehyde (2), was discovered and characterized. Moreover, the enzyme was engineered to enhance the affinity for formaldehyde; triple mutation led to the reduction of the KM value from 62 to 18 mM. The combination of EcGCLR484MN283QL478M and a lactaldehyde reductase (FucO) of E. coli K-12 allowed the production of industrially relevant C2 chemicals (e.g., ethylene glycol (3)) via glycolaldehyde (2) from formaldehyde (1) with a rather high conversion (Scheme 1).
 |
| Scheme 1 C1 biotransformation pathways. Formaldehyde (1) is enzymatically converted into ethylene glycol (3) via glycolaldehyde (2). | |
Experimental procedures
Bacterial strains and culture medium
Escherichia coli BL21 Star(DE3) and recombinant E. coli BL21 Star(DE3) cells expressing heterologous proteins were cultivated in lysogeny broth (LB) (5 g L−1 yeast extract, 10 g L−1 tryptone, and 10 g L−1 NaCl) containing appropriate antibiotics (Table S1†).
Chemicals and reagents
Formaldehyde solution, glycolaldehyde dimer, glycolaldehyde-1-13C solution (0.1 M in water, 99 atom% 13C, 97% (CP)), ethylene glycol, thiamine diphosphate (ThDP), magnesium chloride hexahydrate, diphenyl amine, sulfuric acid, 1,3-propanediol, O-(2,3,4,5,6-pentafluorobenzyl) hydroxylamine hydrochloride (PFBHA), glycerol dehydrogenase, isopropyl β-D-thiogalactoside (IPTG), and ampicillin were purchased from Sigma (St Louis, MO, USA). LB was purchased from Difco Laboratories Inc. (Detroit, MI, USA). Phosphate-buffered saline (PBS) and potassium phosphate dibasic anhydrous were obtained from MP Biomedicals (CA, USA). N,O-Bis(trimethyl-silyl) trifluoroacetamide (BSTFA), acetylacetone, flavin adenine dinucleotide (FAD), and reduced form β-nicotinamide adenine dinucleotide disodium salt hydrate (NADH) were obtained from Tokyo Chemical Industry Co. (Tokyo, Japan). Ni-NTA agarose was purchased from Qiagen (Hilden, Germany). Ethyl acetate, ammonium acetate, n-butyl alcohol, potassium phosphate monobasic, and glacial acetic acid were purchased from Duksan Pure Chemical Co. (Ansan, Korea). Cyclohexanone was obtained from Samchun Pure Chemical Co. (Seoul, Korea).
Gene cloning and construction of recombinant plasmids
The genes coding for GCL were amplified by polymerase chain reactions (PCRs) from chromosomal DNA of E. coli K-12 and Deinococcus metallilatus using primers (Table S2†), which were designed for ligation-independent cloning (LIC).12 Each PCR product was separately treated with the T4 polymerase and ligated with the T4 polymerase treated vector pLIC.B3, a derivative pET-21a (Novagen, Madison, WI). The target gene was designed to translate the protein in a fused state with a 6xHis and tobacco etch virus (TEV) protease cleavage sequence at the N-terminus of the protein. The FLS gene was synthesized (Bioneer, Daejeon, Korea) and then amplified by PCR using primers (Table S2†). The amplified genes were respectively inserted into the E. coli expression vector pET21b(+) using NdeI and XhoI restriction enzymes. All the used primers to amplify the designated genes are listed in Table S2†. fucO was amplified by PCR from chromosomal DNA of E. coli K-12 using primers (Table S2†), which were designed for the In-Fusion cloning protocol (Takara Bio. Inc, CA, USA). The amplified gene was inserted into the linearized E. coli expression vector pACYCduet (Novagen, Madison, WI) using BamHI and HindIII and ligated with the In-Fusion enzyme.
Construction of EcGCL variants
The EcGCL variants were constructed by site-directed mutagenesis as previously reported.13 In short, it was performed by PCR using Pfu plus DNA polymerase with both sense and anti-sense primers. All the used primers to amplify the designated genes are listed in Table S2†. The PCR products were digested with DpnI to ensure the removal of the plasmid DNA template, followed by transformation into E. coli DH5α cells.
Expression and purification of the enzymes
The recombinant E. coli BL21 Star(DE3) cells expressing the heterologous enzymes were cultivated in LB containing appropriate antibiotics (Table S2†). When OD600 reached 0.4–0.6, 0.05 mM IPTG was added into the culture broth, followed by additional 16 h at 18 °C. The recombinant cells were harvested by centrifugation at 8660g for 20 min at 4 °C. The cell pellet was resuspended in a lysis buffer [20 mM Tris-HCl (pH 7.5), 300 mM NaCl, and 1 mM β-mercaptoethanol (β-ME)], containing 1 mM phenylmethylsulfonyl fluoride, and disrupted by sonication. The supernatant was collected by centrifugation at 8660g for 30 min at 4 °C to remove the cell debris. The soluble protein sample was loaded onto a nickel affinity column (Qiagen). The bound protein was eluted by a step gradient of imidazole in the lysis buffer. The eluted protein was concentrated and dialyzed against the lysis buffer by ultrafiltration with an Amicon Ultra centrifugal filter device (Millipore, USA) with a 30 kDa molecular-weight cutoff. For the crystallization of GCL from D. metallilatus (DmGCL), the recombinant protein was digested with a recombinant TEV protease to remove the 6xHis tag and was dialyzed by a buffer containing only 20 mM Tris-HCl (pH 7.5). DmGCL was further isolated using a HiTrap Q column (GE healthcare, Uppsala, Sweden) operated with a linear NaCl gradient. The eluted protein was concentrated and dialyzed with a buffer containing 20 mM Tris-HCl (pH 7.5) and 200 mM sodium chloride. The protein was finally concentrated against buffer by ultrafiltration with an Amicon Ultra centrifugal filter device (Millipore, USA) with a 30 kDa molecular-weight cutoff.
Assay of carboligation activity of the enzymes
The formaldehyde-carboligating activity of enzymes was determined by adding 1 mg mL−1 purified enzymes into 2 mL of the reaction medium (i.e., 50 mM phosphate buffer (pH 6.0)) containing 25 mM formaldehyde, 0.5 mM ThDP, and 5 mM MgCl2·6H2O, which was based on previous studies.14 The reaction was stopped by adding 10% (v/v) H2SO4. The product concentrations were measured by high performance liquid chromatography (HPLC) and/or gas chromatography/mass spectrometry (GC/MS), as previously reported.15
Reaction kinetics of the enzymes
GCL and variant kinetics.
The EcGCL reaction kinetics was evaluated on the basis of earlier studies.5 In brief, the enzyme activity was measured by the coupling reaction with glycerol dehydrogenase (GDH), which consumes NADH from the reduction of the EcGCL reaction product (i.e., glycolaldehyde). The reactions were carried out in 50 mM potassium phosphate buffer (pH 6.0), containing 5 mM MgCl2·6H2O, 0.5 mM ThDP, 0.8 mM NADH, and 0.5 units of GDH in final volume. The formaldehyde concentration was varied from 10 to 150 mM.
Glycolaldehyde reductase kinetics.
The DhaT and FucO reaction kinetics were determined by measuring the reaction rates by monitoring the decrease at Abs340 nm.16 The enzyme concentration was adjusted to 5 μg L−1 in 50 mM Tris-HCl buffer (pH 7.0) containing 0.01 mM NADH in 1 ml of final volume. The reaction was initiated by the addition of varying concentrations of glycolaldehyde from 0.5 to 80 mM.
Thermostability of the enzymes
The thermostability of the enzymes (e.g., EcGCL and FLS) was estimated by measuring the residual activities during incubation at various temperatures from 30 to 60 °C. The residual activities were determined by measuring the product concentrations over time by GC/MS, based on our previous studies.17
Tolerance to glycolaldehyde
The toxic effects were determined by measuring the activity of EcGCL after incubation for 1 h with different concentrations of glycolaldehyde from 0 to 100 mM as previously reported.18,19 The reaction was initiated by adding 25 mM formaldehyde into 50 mM potassium phosphate buffer (pH 6.0) containing 5 mM MgCl2·6H2O, 0.5 mM ThDP, and 1 mg mL−1 purified enzyme. The concentration of glycolaldehyde had been measured as in an earlier study.7 The chromogenic reagent was prepared as followed: 0.5 g of diphenyl amine was dissolved into the solution containing 33.3 mL of acetic acid and then 0.5 mL of H2SO4 was added. The plate was incubated at 90 °C for 15 min and 20 μL of the diluted sample was transferred to the plate and 100 μL of the chromogenic reagent was added to each well. A standard curve was prepared in the range of 0.5 mM to 1.5 mM. The absorbance was read at 650 nm with a Versamax microplate reader with the SoftMax Pro 5.2 software.
Structure determination of DmGCL
For crystallization, the purified DmGCL protein was concentrated to 11.8 mg mL−1 in 20 mM Tris-HCl (pH 7.5) and 200 mM NaCl. The protein concentration was determined by considering the extinction coefficient of 0.950 mg−1 mL cm−1, which was calculated from its amino-acid sequence. To obtain the protein crystals, screening using commercial solution kits was attempted by the sitting-drop vapor-diffusion method at 21 °C. The initial crystals were grown from a precipitant solution consisting of 0.1 M sodium citrate tribasic (pH 5.6), 0.1 M sodium chloride, and 18% (v/v) polyethylene glycol 400 (PEG400). The crystals suitable for diffraction experiments were obtained in 0.1 M sodium citrate tribasic (pH 5.6), 0.1 M sodium chloride, 18% (v/v) PEG400, and 0.5 mM ThDP, using hanging-drop vapor-diffusion method. For diffraction experiments, the crystals were immersed into the precipitant solution containing an additional 25% (v/v) ethylene glycol as a cryoprotectant for 5 s and placed in a 173 °C nitrogen-gas stream. Diffraction data of DmGCL was collected on the beamline BL-11C of the Pohang Accelerator Laboratory (PAL) in Korea. Indexing, integration, and scaling of the reflections were done using the HKL2000 suite.20 The electron-density map of DmGCL was calculated from molecular replacement with the PHENIX program.21 using the structure of EcGCL (PDB ID 2PAN) as a search model. Further model building was done manually using WinCoot,22 and subsequent refinement was performed with PHENIX.21 The data collection and refinement statistics are summarized in Table S3.† The atomic coordinates and structure factors were deposited in the Protein Data Bank (http://www.rcsb.org/pdb) with accession ID 7CT6.
Whole-cell biotransformation
The carboligation of formaldehyde by recombinant E. coli BL21 Star(DE3) pLIC.B3-EcGCL and variants was carried out in 50 mM potassium phosphate buffer (pH 7.0). The shaking speed and temperature were 250 rpm and 40 °C, respectively. After cultivation at 37 °C and gene expression at 18 °C overnight, the cells were harvested by centrifugation at 2602g for 15 min at 4 °C. The biotransformation was initiated by adding 20 mM formaldehyde into 50 mM potassium phosphate buffer (pH 7.0) containing 5 mM MgCl2·6H2O, 0.5 mM ThDP, and recombinant cells (cell concentration: 11 g dry cells per L). The biotransformation of formaldehyde into ethylene glycol by the recombinant E. coli BL21 Star(DE3) pLIC.B3-EcGCL and pACYCduet-FucO was conducted as described above.
Analytical methods
The formaldehyde concentrations were measured by a modification of the Nash reaction for 96-well plates.23 100 μL of the sample with 10% (v/v) H2SO4 was centrifuged 3 min at 11
883g and diluted to lower than 1.0 mM formaldehyde. 100 μL of the diluted sample was transferred to the plate and 100 μL of Nash reagent (0.2% (v/v) 2,4-pentanedione, 0.1 M acetic acid, and 3.89 M ammonium acetate) was added to each well. The plate was incubated at 37 °C for 30 min, and absorbance was read at 412 nm with a Versamax microplate reader with the SoftMax Pro 5.2 software. A standard curve was prepared in 9% (v/v) sulfuric acid solution in the range of 0.1 mM to 25 mM. The glycolaldehyde concentrations were determined as previously described.24 The samples with 10% (v/v) H2SO4 were vortexed and then centrifuged at 11
883g. After addition of the internal standard (cyclohexanone) and (PFBHA) solution, the mixture was left at room temperature for derivatization. Then, the sample was mixed with ethyl acetate as an extraction solvent, and vortexed and centrifuged for 3 min. Then 80 μL of the supernatant was evaporated and 25 μL of BSTFA was added at 65 °C for 30 min for derivatization. After derivatization, the samples were analysed by GC/MS. The GC/MS analyses were performed with a 7820A GC model (Agilent) with a HP-5ms fused silica capillary column (30 m length, 0.25 mm film thickness, Agilent Technologies, Palo Alto, CA, USA). Injections (1 μL) were performed with a 1/25 split ratio and the GC oven was programmed from 100 °C (held for 1 min) to 180 °C at 15 °C min−1, to 200 °C at 12 °C min−1 and then held at 250 °C for 2 min the total analysis time was 8.5 min. For the measurement of the ethylene glycol concentrations, 100 μL of n-butyl alcohol as an extraction solvent, containing 5 μL of the internal standard (2800 mg L−1 1,3-propanediol in distilled water), was added to 20 μL of the sample which was prepared by addition of 50% HCl.25 The samples were vortexed for a minute and then centrifuged for 2 min at 11
883g. The 20 μL of supernatant was evaporated and 25 μL of BSTFA was added at 65 °C for 30 min for derivatization. After derivatization, the samples were analysed by GC/MS. The GC/MS analyses were performed with a model 7820A GC (Agilent) with a HP-5ms fused silica capillary column (30 m length, 0.25 mm film thickness, Agilent Technologies, Palo Alto, CA, USA). Injections (1 μL) were performed with a 1/60 split ratio and the GC oven was programmed from 50 °C (held for 1 min) to 100 °C at 10 °C min−1, to 140 °C at 40 °C min−1 and then held at 200 °C for 1 min. The total analysis time was 7 min.
Results and discussion
Discovery of a carboligase for the synthesis of glycolaldehyde from formaldehyde
Screening of a carboligase.
In order to find out the candidate enzymes for ligation of two molecules of formaldehyde into one molecule of glycolaldehyde (Scheme 1), we performed the structural similarity search using the DALI server (http://ekhidna2.biocenter.helsinki.fi/dali) and the synthetic formaldehyde converting FLS (PDB ID 4QQ8) as a reference structure against the listed proteins in the protein data bank (http://www.rcsb.org). The search retrieved a number of structurally related enzymes (Z scores of more than 30 and root mean square deviations (RMSD) of less than 3 Å) (Table S4†), including acetolactate synthases (ALSs), oxalyl-CoA decarboxylases (OCDs, 2Q28), and glyoxylate carboligases (GCLs). Most of these enzymes are ThDP-dependent even though some enzymes require another cofactor, such as FAD, for their reported catalytic activities. Considering their minute structural features around the active site and substrate specificities (Fig. S1†), we selected two GCLs from E. coli (EcGCL)26 and Deinococcus metallilatus (DmGCL), two ALS from Vibrio vulnificus and Hydrogenobacter thermophilus, and OCD from E. coli(Fig. 1).
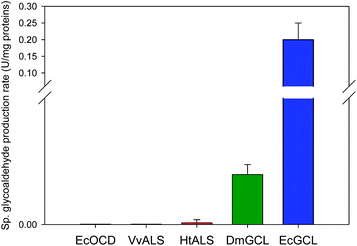 |
| Fig. 1 Comparison of the formaldehyde-carboligating activities of various carboligases. The enzymes (e.g., EcOCD, VvALS, HtALS, DmGCL, and EcGCL) were added to 1 mg mL−1 in 50 mM potassium phosphate buffer (pH 6.0) containing 25 mM formaldehyde (1) and sufficient amount of magnesium(II) ion, thiamine diphosphate (ThDP) and flavin adenine dinucleotide (FAD) (see the Materials and methods section for details). The initial bioconversion rates were determined based on the product concentrations measured by HPLC and/or GC/MS. | |
Activity of carboligase.
The C1 carboligation activities of the candidate enzymes were examined after expression in E. coli and purification by affinity chromatography on a Ni-NTA gel matrix (see the Materials and methods section for details). Most enzymes were expressed in a soluble form in E. coli and purified as a single band on SDS-PAGE (Fig. S2†). Among the tested enzymes, the glyoxylate carboligases (GCLs) exhibited greater carboligation activities for formaldehyde than other enzymes. In particular, the GCL from E. coli K-12 (EcGCL) has shown the best catalytic activity (Fig. 1 and Fig. S3†). The initial glycolaldehyde production rate of the EcGCL reached 0.2 U mg−1 proteins. Notably, glycolaldehyde (2) produced by EcGCL was not further converted into dihydroxyacetone (3), which had been observed in FLS catalysis5 (Scheme S1† and Table 1). Thus, the enzyme could be suitable for the production of glycolaldehyde (2) from formaldehyde (1) with a high conversion. The catalytic activities of EcGCL for other C2 and C3 aldehydes were also investigated. The EcGCL has little activity for ligation of acetaldehyde and 3-hydroxypropionaldehyde.
Table 1 Relative activities of carboligases for formaldehyde and acetaldehyde
Name |
Source |
Activity for formaldehyde |
Activity for acetaldehyde |
Ref. |
ND: not determined or not reported.
|
EcGCL |
Escherichia coli
|
++++ |
— |
This study |
DmGCL |
Deinococcus metallilatus
|
++ |
— |
This study |
FLS |
Pseudomonas fluorescens
|
++++a |
++++ |
5 and 15 |
GALS |
Pseudomonas putida
|
++++ |
NDa |
7
|
Characteristics of GCL
Thermostability of EcGCL.
The reaction temperature is one of the key parameters in enzyme catalysis. Therefore, thermostability of the newly discovered enzyme (EcGCL) was examined (Fig. 2). Interestingly, the catalytic activity of EcGCL was rather stably maintained at 50 °C for 5 h. This is outstanding as compared to thermal stability of FLS, which lost its activity at 50 °C within 30 min (data not shown).
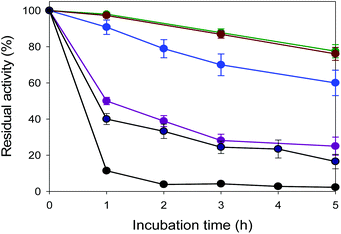 |
| Fig. 2 Thermostability of EcGCL. The thermal stability of EcGCL was evaluated during incubation in potassium phosphate buffer at various temperatures. The enzymes, which were produced by E. coli and purified via affinity chromatography on a Ni-NTA gel matrix, were used for the experiments. The initial activity of the enzyme was 0.2 U mg−1 proteins. The symbols indicate the residual activity during incubation at 30 °C ( ), 40 °C ( ), 50 °C ( ), 53 °C ( ), 55 °C ( ) and 60 °C (●) respectively. | |
Kinetic properties.
The steady-state kinetic studies revealed that EcGCL showed KM, kcat, and kcat/KM values of 62 mM, 0.1 s−1, and 1.6 M−1 s−1, respectively. Although the catalytic efficiency was lower than that of GALS,7 the substrate affinity of EcGCL was greater (KM: 62 mM vs. 170 mM). Overall, it was concluded that a new carboligase (i.e., EcGCL) for the production of glycolaldehyde from formaldehyde was successfully discovered.
Molecular features and presumed carboligase mechanisms of GCL
Naturally, GCL catalyzes the condensation of two glyoxylate molecules to yield a tartronate semialdehyde and carbon dioxide through the successive decarboxylation and reduction reactions (Scheme S2†).26 Only the crystal structure of EcGCL has been reported to date, which shares structural features with the typical pyruvate oxidase family of ThDP enzymes.27
In order to add structural information on GCL proteins, we determined the crystal structure of GCL from D. metallilatus (DmGCL) (Fig. 3A). Although DmGCL showed lower catalytic activity than EcGCL (Fig. 1), the overall structural features are similar to those of EcGCL (RMSD of 0.805 Å among the aligned 526 residues). One of the differences is that the α-helix (Ala474-Leu494 of EcGCL) provides residues for interactions with the ThDP and the magnesium ion in EcGCL, which is completely displaced from the active site in the DmGCL structure. Therefore, it is plausible that the flexibility of this region might be related to the lower activity of DmGCL than that of EcGCL.
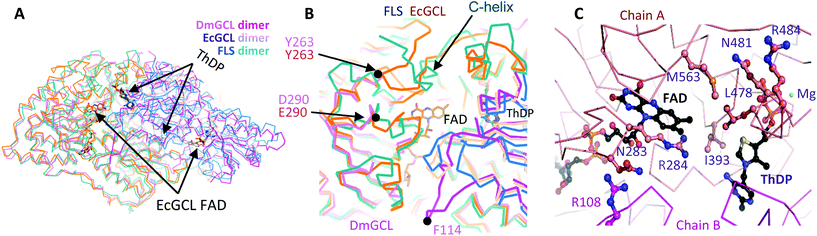 |
| Fig. 3 Structural features of GCLs. (A) Superposed structures of two dimeric GCLs on the dimeric FLS, which were differentiated by colours. The two cofactors of ThDP in EcGCL and FLS and FAD in EcGCL are depicted as stick models. (B) The magnified view of the active sites of three enzymes. The regions with large deviations upon superposition are indicated by arrows. The Phe114-containing loop of DmGCL is far apart from the active site. (C) Mutation points (e.g., R484, R284, R108, M563, Q481, I393, N283, and L478). Each subunit is displayed with alternating colours. Some residues and two cofactors of ThDP and FAD are drawn with ball-and-stick models and a magnesium ion is with a sphere. | |
Comparison of the two GCL structures with FLS reveals a variation in their active sites with a remarkable difference in the extended region at the extreme C-termini of three enzymes (C-helix in Fig. 3B) that contribute to forming the active sites of the GCL and the FLS enzymes. Notably, the two GCLs require another cofactor FADH2 near the THDP cofactor that is necessary for the reduction of the second intermediates. Nonetheless, the GCLs exactly reflect the structural features of the ThDP-dependent carboligase enzymes and does not require a reduction step for carboligation of two formaldehydes. Therefore, the catalytic mechanism for condensing the two formaldehyde molecules by GCL is presumably same as that of benzaldehyde lyase.28
Engineering of GCL
Improving affinity for formaldehyde.
Glyoxylate, the natural substrate of GCL, is a two-carbon compound with a negatively charged carboxylate group (Scheme S2†). Therefore, the revealed structure of EcGCL shows a number of positively charged residues at the entrance of the substrate access channel to the active site (Fig. 3C). Since formaldehyde is less polar than glyoxylate, we surmised that neutralization of a positive charge at the entrance of the active site may affect the catalytic activity of EcGCL. However, catalytic activities of most single mutants remained rather lower than that of the wild-type enzyme (Fig. S4†). Interestingly, one mutant (EcGCLR484M) showed the glycolaldehyde formation rate, which is comparable to the wild-type enzyme. Since this mutant was also rather more stable than the wild-type enzyme under the reaction condition (data not shown), it was adopted as a template for further mutational studies.
The next round of mutations focused on both neutralization of a positive charge and modification of the diameter of the tunnel to the active site. Added mutations such as R284M, R108L, M563V, Q481L, and I393G to EcGCLR484M led to decrease of the catalytic efficiencies, whereas a double mutant (i.g., EcGCLR484MN283Q) exhibited higher substrate affinity and catalytic efficiency for formaldehyde (Table 2 and Table S5†). Addition of another mutation at Leu478 to this double mutant, a triple mutant (EcGCLR484MN283QL478M), enabled the substrate affinity and catalytic efficiency to reach 18 mM and 5.2 M−1 s−1, respectively (Table 2 and Table S6†). The KM value was approximately 3-fold lower than that of the wild-type enzyme, resulting in a 3-fold greater kcat/KM value. Taken together, these results indicated that the size and the surface charge of the substrate access tunnel would be important to the catalytic efficiency of EcGCL for carboligation of two formaldehyde molecules.
Table 2 Kinetic parameters of wild-type EcGCL and its variantsa
Enzyme |
K
M (mM) |
k
cat (s−1) |
k
cat/KM (M−1 s−1) |
The reaction was initiated by adding purified EcGCL or its variants into potassium phosphate buffer (pH 6.0) containing glycerol dehydrogenase and various concentrations of formaldehyde. The reaction rate was determined by NADH assay.5
Glycolaldehyde synthase, which was constructed from benzoylformate decarboxylase of Pseudomonas putida via multiple mutations.7
|
Wild type |
62 |
0.1 |
1.6 |
EcGCLR484MN283Q |
39 |
0.1 |
2.8 |
EcGCLR484MN283QL478M |
18 |
0.1 |
5.2 |
GALSb |
170 |
1.6 |
9.6 |
Biotransformation performance of EcGCL variants for carboligation of formaldehyde.
With an aim to evaluate formaldehyde condensation activities of the EcGCL variants, biotransformation of formaldehyde into glycolaldehyde by recombinant E. coli expressing each EcGCL variant was carried out (Fig. 4). When the recombinant E. coli expressing EcGCL was used as a biocatalyst, glycolaldehyde was produced at a rate of 2.4 mM h−1 (3.6 U g−1 dry cells) in the reaction medium at t < 1 h. However, the use of the triple mutant (EcGCLR484MN283QL478M) enabled the glycolaldehyde production rate of 4.3 mM h−1 (6.5 U g−1 dry cells) (Fig. 4C). This result indicated that the greater catalytic efficiency of triple mutant led to the higher product formation rate.
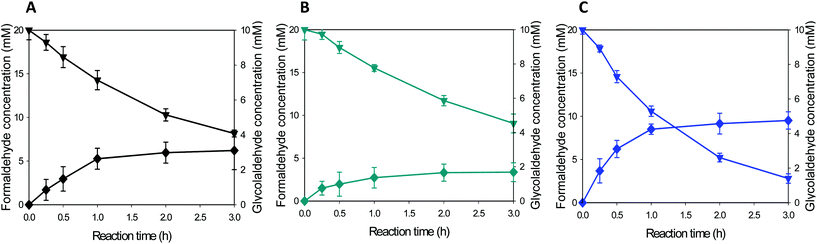 |
| Fig. 4 Time course of formaldehyde biotransformation by recombinant E. coli expressing EcGCL or its variants. The whole cell biotransformation was conducted by recombinant E. coli expressing EcGCL or EcGCL variants (cell density: 11 g dry cells per L) at 40 °C; EcGCL (A), EcGCLR484MN283Q (B), and EcGCLR484MN283QL478M (C). The symbols indicate the concentration of formaldehyde (▼) and glycolaldehyde (◆). | |
The product formation ceased at t > 1 h in all the biotransformations. One of the reasons might include deleterious reactions of glycolaldehyde to EcGCL (Fig. S5†). It was reported that glycolaldehyde may cause cross-linking reaction with nucleophilic amino acid residues (e.g., cysteine) in proteins.29
Enzymes for the reduction of glycolaldehyde into ethylene glycol
Lactaldehyde reductase (FucO) of E. coli K-12, which had been reported to catalyze reduction of glycolaldehyde into ethylene glycol,30 and the 1,3-propanediol dehydrogenase (DhaT) of Klebsiella pneumoniae31 were examined for the conversion of glycolaldehyde into ethylene glycol (Scheme 1). The kinetic studies revealed that FucO showed great catalytic efficiency for glycolaldehyde with rather low catalytic efficiency for formaldehyde (Table 3). In the case of DhaT, the catalytic efficiency for glycolaldehyde was similar to that for formaldehyde. Therefore, FucO would be more appropriate for whole-cell biotransformation of formaldehyde into ethylene glycol via glycolaldehyde for high conversion (Scheme 1).
Table 3 Kinetic constants of FucO and DhaT for formaldehyde (1) and glycolaldehyde (2)a
Enzyme |
Substrate |
K
M (mM) |
k
cat (s−1) |
k
cat/KM (mM−1 s−1) |
The reaction kinetics was determined by NADH assay. The enzymes were added to 5 μg L−1 in 50 mM potassium phosphate buffer (pH 7.0) containing 0.01 mM NADH and various concentrations of the reaction substrates.
|
FucO |
Formaldehyde |
15 |
0.7 |
0.05 |
Glycolaldehyde |
2.4 |
24 |
10 |
DhaT |
Formaldehyde |
24 |
2.6 |
0.08 |
Glycolaldehyde |
290 |
14 |
0.05 |
Whole-cell biotransformation of formaldehyde into ethylene glycol
Whole-cell biocatalysts for the conversion of formaldehyde into ethylene glycol were designed by using EcGCLR484MN283QL478M and FucO. In order to avoid accumulation of the toxic reaction intermediate (i.e., glycolaldehyde (2)) in the reaction medium, EcGCLR484MN283QL478M was cloned and expressed in a high-copy number vector (pET21a), while FucO was expressed in a low-copy number vector (pACYCDuet). The SDS-PAGE analysis of recombinant E. coli BL21 Star(DE3) expressing EcGCLR484MN283QL478M and FucO showed that both enzymes were expressed in a soluble form as designed (Fig. S6†).
The whole-cell biotransformation was initiated by adding 20 mM formaldehyde into the potassium phosphate buffer containing the recombinant E. coli cells to 11 g dry cells per L. The starting material was quickly converted into ethylene glycol via glycolaldehyde by the intracellular enzymes (Fig. 5). Notably, the carboligation of formaldehyde into glycolaldehyde was proceeded to a rate of 6 mM h−1 at t < 1 h, which is greater than the reaction rate of the recombinant E. coli BL21 Star(DE3) expressing solely EcGCLR484MN283QL478M (Fig. 4C). Furthermore, the final product concentration reached 6.6 mM in the reaction medium without leaving any reaction substrates (1) and intermediates (2). The final product concentration was approximately 50% greater than that of recombinant E. coli BL21 Star(DE3) expressing solely EcGCLR484MN283QL478M. This might be due to the result that the toxic reaction intermediate (2) was maintained below 1.5 mM in the reaction medium in the case of recombinant E. coli BL21 Star(DE3) expressing the EcGCLR484MN283QL478M and FucO. The second step reaction (i.e., reduction of glycolaldehyde into ethylene glycol (Scheme 1)) rate was comparable to that of the recombinant E. coli BL21 Star(DE3) expressing solely FucO. This result indicated that the cascade enzymatic reaction of formaldehyde into ethylene glycol via glycolaldehyde in a whole-cell proceeded efficiently proceeded as compared to the single step whole-cell reactions. The increase of formaldehyde concentration to 40 mM in the reaction medium did not lead to enhancement in the ethylene glycol concentrations (Fig. S7†). This might be due to toxic effects of formaldehyde and glycolaldehyde toward the bioconversion enzymes and/or E. coli cells. The final product (3) was isolated via extraction with acetonitrile after in vacuo evaporation of the reaction broth. The resulting organic layer was evaporated to yield the product as a transparent liquid. Overall, the target product (3) was isolated to a yield of ca. 45% from the reaction mixture, with a purity of >90% (Fig. S8†).
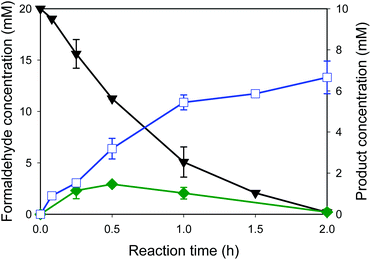 |
| Fig. 5 Time course of the cascade reaction of formaldehyde (1) into ethylene glycol (3). Biotransformation of formaldehyde into ethylene glycol was initiated by adding 20 mM formaldehyde at t = 0 into the potassium phosphate buffer containing 11 g dry cells per L of recombinant E. coli expressing the EcGCLR484MN283QL478M and FucO. The symbols indicate the concentration of formaldehyde (▼), glycolaldehyde ( ) and ethylene glycol ( ). | |
Although a number of C1 carboligases and their variants7,8 have been examined, biosynthesis of C2 chemicals from C1 compounds to a high conversion was rarely reported. This study demonstrated that one of the industrially relevant C2 chemicals, ethylene glycol could be produced from formaldehyde at a rather high conversion. This was possible probably due to overexpression of the bioconversion enzymes (i.e., EcGCL and FucO) to a functional form in the host cells and a rather low KM value of the EcGCL variants. One of the key factors to achieve high concentrations of the target products may include tolerance of the bioconversion enzymes and host cells towards the toxic and reactive reactants and products, as shown in Fig. S7.† Therefore, our further studies will focus on enhancing chemical resistance of the biotransformation enzymes and application of chemical tolerant strains as host cells, based on our previous studies.18,32
Conclusions
C1 chemicals of low molecular weights are volatile and not easily recovered from air. They contribute to many environmental issues that entail a high social cost. Interestingly, formaldehyde is more chemically active than the other C1 chemicals. A novel carboligase for the condensation of formaldehyde into glycolaldehyde (e.g., glyoxylate carboligase from E. coli K-12 (EcGCL)) was discovered and engineered for the successful whole-cell biotransformation of formaldehyde into a C2 compound (i.e., glycolaldehyde) at a high rate. Furthermore, this study demonstrated for the first time that this carboligase could be used for the cascade biotransformation of formaldehyde into ethylene glycol via glycolaldehyde. This study will contribute to the valorization of C1 compounds into not only C2 compounds but also C4 compounds (e.g., erythrulose) (Scheme S1†) in a cheap and environment-friendly way.
Accession number
Atomic coordinates of D. metallilatus GCL were deposited in the Protein Data Bank, http://www.rcsb.org (accession number 7CT6).
Author contributions
Hye-Jin Jo: data curation, formal analysis, investigation, visualization, writing – original draft, Jun-Hong Kim: data curation, formal analysis, investigation, visualization, writing – original draft, Ye-Na Kim: data curation, formal analysis, Pil-Won Seo: data curation, formal analysis, validation, Chae-Yun Kim: data curation, formal analysis, Ji-Won Kim: data curation, formal analysis, Han-na Yu: data curation, formal analysis, Huijin Cheon: data curation, formal analysis, validation, Eun Yeol Lee: conceptualization, writing – review & editing, Jeong-Sun Kim: conceptualization, funding acquisition, supervision, writing – review & editing, Jin-Byung Park: conceptualization, funding acquisition, supervision, writing – review & editing.
Conflicts of interest
There are no conflicts to declare.
Acknowledgements
The X-ray diffraction experiments were performed with Beamline 11C at the Pohang Accelerator in Korea. This work was supported by C1 Gas Refinery Research Center (NRF grant number: 2018M3D3A1A01055735) of the National Research Foundation (NRF) of Korea funded by Ministry of Science and ICT. D. metallilatus genomic DNA was obtained from the Radiation Bio-Resources Bank (RBB) at KAERI Advance Radiation Technology Institute, Republic of Korea. We also thank Prof. Sunghoon Park (Pusan National University) for the kind donation of dhaT.
References
-
(a) S. Desmons, R. Fauré and S. Bontemps, ACS Catal., 2019, 9, 9575–9588 CrossRef CAS
;
(b) L. E. Heim, H. Konnerth and M. H. G. Prechtl, Green Chem., 2017, 19, 2347–2355 RSC
.
-
(a) M. Rauch, Z. Strater and G. Parkin, J. Am. Chem. Soc., 2019, 141, 17754–17762 CrossRef CAS PubMed
;
(b) H. H. Cramer, B. Chatterjee, T. Weyhermüller, C. Werlé and W. Leitner, Angew. Chem., 2020, 132, 2–10 (
Angew. Chem., Int. Ed.
, 2020
, 59
, 2–10
) CrossRef
;
(c) E. Yang, J. G. Lee, D. H. Kim, Y. S. Jung, J. H. Kwak, E. D. Park and K. An, J. Catal., 2018, 368, 134–144 CrossRef CAS
.
-
(a) H. Fraenkel-Conrat and H. S. Olcott, J. Biol. Chem., 1948, 174, 827–843 CrossRef CAS
;
(b) H. Fraenkel-Conrat and D. K. Mecham, J. Biol. Chem., 1949, 177, 477–486 CrossRef CAS
;
(c)
M. Y. Feldman, in Prog. Nucleic Acid Res. Mol. Biol, Elsevier, 1973, vol. 13, pp. 1–49 Search PubMed
;
(d) B. A. Hansen, R. S. Lane and E. E. Dekker, J. Biol. Chem., 1974, 249, 4891–4896 CrossRef CAS PubMed
.
-
(a) T. Zhu, T. Zhao, O. E. Bankefa and Y. Li, Biotechnol. Adv., 2020, 39, 107467 CrossRef CAS
;
(b) Y. Wang, L. Fan, P. Tuyishime, P. Zheng and J. Sun, Trends Biotechnol., 2020, 38, 650–666 CrossRef CAS PubMed
;
(c) J. Yang, S. Sun, Y. Men, Y. Zeng, Y. Zhu, Y. Sun and Y. Ma, Catal. Sci. Technol., 2017, 7, 3459–3463 RSC
;
(d) A. D. Nguyen and E. Y. Lee, Trends Biotechnol., 2021, 39, 381–396 CrossRef CAS
;
(e) T. Li, Z. Tang, H. Wei, Z. Tan, P. Liu, J. Li, Y. Zheng, J. Lin, W. Liu and H. Jiang, Green Chem., 2020, 22, 6809–6814 RSC
.
- J. B. Siegel, A. L. Smith, S. Poust, A. J. Wargacki, A. Bar-Even, C. Louw, B. W. Shen, C. B. Eiben, H. M. Tran and E. Noor, Proc. Natl. Acad. Sci. U. S. A., 2015, 112, 3704–3709 CrossRef CAS
.
- S. Güner, V. Wegat, A. Pick and V. Sieber, Green Chem., 2021, 23, 6583–6590 RSC
.
- X. Lu, Y. Liu, Y. Yang, S. Wang, Q. Wang, X. Wang, Z. Yan, J. Cheng, C. Liu and X. Yang, Nat. Commun., 2019, 10, 1–10 CrossRef
.
-
(a) A. Chou, J. M. Clomburg, S. Qian and R. Gonzalez, Nat. Chem. Biol., 2019, 15, 900–906 CrossRef CAS
;
(b) M. Nattermann, S. Burgener, P. Pfister, A. Chou, L. Schulz, S. H. Lee, N. Paczia, J. Zarzycki, R. Gonzalez and T. J. Erb, ACS Catal., 2021, 11, 5396–5404 CrossRef CAS
.
-
(a) J. Pang, M. Zheng, A. Wang and T. Zhang, Ind. Eng. Chem. Res., 2011, 50, 6601–6608 CrossRef CAS
;
(b) D. Fan, D.-J. Dai and H.-S. Wu, Materials, 2012, 6, 101–115 CrossRef PubMed
.
- N. Ji, T. Zhang, M. Zheng, A. Wang, H. Wang, X. Wang, Y. Shu, A. L. Stottlemyer and J. G. Chen, Catal. Today, 2009, 147, 77–85 CrossRef CAS
.
- L. Salusjärvi, S. Havukainen, O. Koivistoinen and M. Toivari, Appl. Microbiol. Biotechnol., 2019, 103, 2525–2535 CrossRef PubMed
.
- C. Aslanidis and P. J. De Jong, Nucleic Acids Res., 1990, 18, 6069–6074 CrossRef CAS
.
- J.-M. Woo, E.-Y. Jeon, E.-J. Seo, J.-H. Seo, D.-Y. Lee, Y. J. Yeon and J.-B. Park, Sci. Rep., 2018, 8, 1–11 CAS
.
- L. Zhang, R. Singh, D. Sivakumar, Z. Guo, J. Li, F. Chen, Y. He, X. Guan, Y. C. Kang and J.-K. Lee, Green Chem., 2018, 20, 230–242 RSC
.
- P.-W. Seo, H.-J. Jo, I. Y. Hwang, H.-Y. Jeong, J.-H. Kim, J.-W. Kim, E. Y. Lee, J.-B. Park and J.-S. Kim, Catal. Sci. Technol., 2020, 10, 79–85 RSC
.
- S. Lama, S. M. Ro, E. Seol, B. S. Sekar, S. K. Ainala, J. Thangappan, H. Song, D. Seung and S. Park, Biotechnol. Bioprocess Eng., 2015, 20, 971–979 CrossRef CAS
.
- T.-H. Kim, S.-H. Kang, J.-E. Han, E.-J. Seo, E.-Y. Jeon, G.-E. Choi, J.-B. Park and D.-K. Oh, ACS Catal., 2020, 10, 4871–4878 CrossRef CAS
.
- E.-J. Seo, M.-J. Kim, S.-Y. Park, S. Park, D.-K. Oh, U. Bornscheuer and J.-B. Park, Adv. Synth. Catal., in press DOI:10.1002/adsc.202101044
.
- E.-J. Seo, C. W. Kang, J.-M. Woo, S. Jang, Y. J. Yeon, G. Y. Jung and J.-B. Park, Metab. Eng., 2019, 54, 137–144 CrossRef CAS
.
-
Z. Otwinowski and W. Minor, in Meth. Enzymol, Elsevier, 1997, vol. 276, pp. 307–326 Search PubMed
.
- P. D. Adams, P. V. Afonine, G. Bunkóczi, V. B. Chen, I. W. Davis, N. Echols, J. J. Headd, L.-W. Hung, G. J. Kapral and R. W. Grosse-Kunstleve, Acta Crystallogr., Sect. D: Biol. Crystallogr., 2010, 66, 213–221 CrossRef CAS
.
- P. Emsley and K. Cowtan, Acta Crystallogr., Sect. D: Biol. Crystallogr., 2004, 60, 2126–2132 CrossRef PubMed
.
- T. Nash, Biochem. J., 1953, 55, 416–421 CrossRef CAS
.
- S. Poust, J. Piety, A. Bar-Even, C. Louw, D. Baker, J. D. Keasling and J. B. Siegel, ChemBioChem, 2015, 16, 1950–1954 CrossRef CAS
.
- H. Neels, M. De Doncker, N. Vrydags, K. Schatteman, W. Uyttenbroeck, N. Hamers, D. Himpe and W. Lambert, Clin. Chem. Lab. Med., 2004, 42, 1341–1345 Search PubMed
.
- N. Nemeria, E. Binshtein, H. Patel, A. Balakrishnan, I. Vered, B. Shaanan, Z. e. Barak, D. Chipman and F. Jordan, Biochemistry, 2012, 51, 7940–7952 CrossRef CAS
.
- A. Kaplun, E. Binshtein, M. Vyazmensky, A. Steinmetz, Z. e. Barak, D. M. Chipman, K. Tittmann and B. Shaanan, Nat. Chem. Biol., 2008, 4, 113 CrossRef CAS PubMed
.
- T. G. Mosbacher, M. Mueller and G. E. Schulz, FEBS J., 2005, 272, 6067–6076 CrossRef CAS PubMed
.
-
(a) J. J. Kamps, R. J. Hopkinson, C. J. Schofield and T. D. Claridge, Commun. Chem., 2019, 2, 1–14 CrossRef CAS
;
(b) A. Klaus, R. Rau and M. A. Glomb, J. Agric. Food Chem., 2018, 66, 10835–10843 CrossRef CAS PubMed
.
-
(a) C. Alkim, Y. Cam, D. Trichez, C. Auriol, L. Spina, A. Vax, F. Bartolo, P. Besse, J. M. François and T. Walther, Microb. Cell Fact., 2015, 14, 1–12 CrossRef
;
(b) K. Li, W. Sun, W. Meng, J. Yan, Y. Zhang, S. Guo, C. Lü, C. Ma and C. Gao, Catalysts, 2021, 11, 214 CrossRef CAS
.
- S. Lama, E. Seol and S. Park, Bioresour. Technol., 2017, 245, 1542–1550 CrossRef CAS
.
-
(a) J. Shin, J. Yu, M. Park, C. Kim, H. Kim, Y. Park, C. Ban, E. Seydametova, Y.-H. Song and C.-S. Shin, ACS Synth. Biol., 2019, 8, 1055–1066 CrossRef CAS
;
(b) J.-M. Woo, J.-W. Kim, J.-W. Song, L. M. Blank and J.-B. Park, PLoS One, 2016, 11, e0163265 CrossRef PubMed
;
(c) A. H. Baek, E. Y. Jeon, S. M. Lee and J. B. Park, Biotechnol. Bioeng., 2015, 112, 889–895 CrossRef CAS PubMed
;
(d) J.-W. Song, J.-H. Seo, D.-K. Oh, U. T. Bornscheuer and J.-B. Park, Catal. Sci. Technol., 2020, 10, 46–64 RSC
.
Footnotes |
† Electronic supplementary information (ESI) available. See DOI: 10.1039/d1gc03549e |
‡ These authors contributed equally to this work. |
|
This journal is © The Royal Society of Chemistry 2022 |
Click here to see how this site uses Cookies. View our privacy policy here.